DOI:
10.1039/D2FO02610D
(Paper)
Food Funct., 2023,
14, 344-353
Ten-dimensional hyphenation including simulated static gastro-intestinal digestion on the adsorbent surface, planar assays, and bioactivity evaluation for meal replacement products†‡
Received
4th September 2022
, Accepted 23rd November 2022
First published on 24th November 2022
Abstract
Meal replacement products are normally consumed in weight-loss interventions and the treatment of obesity and diabetes. Changing lifestyles and eating habits made meal replacement products in the forms of shakes and bars a good alternative as To-go-meals, promoted as balanced in its composition and thus healthier compared to other ready-to-eat meals. This study aimed to evaluate the bioactivity of six differently flavoured powdered meal replacement products. Their analysis was made by a ten-dimensional hyphenation composed of digestion on the adsorbent surface, followed by normal-phase high-performance thin-layer chromatographic separation, multi-imaging, and planar assay application (effect-directed analysis), and then heart-cut elution/transfer of bioactive compound zones to reversed-phase high-performance liquid chromatography, diode array detection, and high-resolution tandem mass spectrometry. The on-surface digestion of saccharides, fats, and proteins through intestinal enzymatic activity revealed new breakdown products. These exhibited bioactivity in their different effect-profiles obtained by the Gram-negative Aliivibrio fischeri bioassay as well as α-/β-glucosidase and acetyl-/butyrylcholinesterease inhibition assays. The main bioactive compounds arising through simulated static pancreatic digestion were saturated and unsaturated free fatty acids. The synthetic sweetener sucralose was not influenced by simulated static intestinal digestion, but showed antimicrobial activity. In the prepared drinking meals with coffee and choco flavour, the acetylcholinesterase-inhibiting methylxanthines caffeine and theobromine were identified as bioactive compounds. Some other bioactive constituents could not be assigned to specific molecules and require further analyses. Although the studied meal replacement products showed health-beneficial properties through antimicrobial properties or inhibition of enzymes involved in the expression of the civilisation diseases, such as diabetes and Alzheimer's disease, plant foods, herbs and spices have been shown to be even richer and more versatile in bioactive compounds.
1. Introduction
The nutritional status plays an important role in health.1,2 Overweight, obesity, and health complaints are often consequences of malnutrition and a lack of micronutrients.2 A poor diet is influenced by many factors, e.g., a higher consumption of processed foods3,4 such as sugar-sweetened beverages5–7 or fast food,6 large portion sizes,6,7 or frequent snacking habits.4 Researchers hypothesized that long-term consumption of highly processed food plays a key role in the development of overweight, obesity,4 diabetes type 2,2,5 aggravation of Alzheimer's disease,8,9 and other civilization diseases.3 Food processing includes all processes that alter food from its natural state by drying, freezing, grinding, heating, mixing, pasteurizing, fermenting, packaging, adding salts or sugars, etc.1,3 The various processing steps are intended to maintain food safety and security,1 but the nutritional value may be compromised.10 Nevertheless, highly processed meal replacement products (MRPs) are applied in the treatment of obesity or diabetes.11,12 This seems contradictory at first sight, but usually MRPs have been formulated by nutrition professionals and represent the ideal composition for a balanced diet, which consists of the optimal ratio of proteins, fat, fibre, vitamins, and minerals.1 Due to better nutritional values, MRPs are generally promoted as healthy4,13 and often preferred over whole food in weight-loss interventions.4,14 It is clear that such surrogates cannot substitute the versatility of bioactive compounds present in natural plant-based foods, herbs and spices.15 Thus, the impact on health is unclear for a longer excessive use. Moreover, MRPs such as bars or shakes (e.g., https://uk.yfood.eu) also aim for a time-saving opportunity to maintain a balanced diet and could be a good alternative for busy lifestyles not allowing constant eating habits.16 Regardless of the purpose for which the MRPs are consumed, health-promoting properties are advertised to users.13
Besides a balanced diet through MRPs, it is assumed that individual biologically active ingredients can contribute to health positively as well. As human metabolization alters the bioactivity,17–20 it is significant if active compounds survive the gastrointestinal passage to exhibit their effects. Versatile metabolic transformation pathways of the gastrointestinal tract (GIT), e.g., microbial or enzymatic reactions, metabolise food components to get absorbed or influence the microbiome.18 The most prominent enzyme classes of the intestine are lipases, amylases, and proteases, responsible for the breakdown of fats, saccharides, and proteins, respectively. Digestive processes alter the constituents ingested with food through chemical, biochemical, and microbiological reaction cascades. Constituents could either be unaffected by digestive pathways or metabolized into an active or inactive form. Therefore, screening for health-beneficial substances from processed foods should always consider further processing by human metabolization.
In this study, the recently developed ten-dimensional bioanalytical hyphenation21 was employed to screen for stable, degraded or metabolised bioactive compounds in six differently flavoured MRPs. The bioanalytical workflow incorporated a miniaturized on-surface digestion (1D, nanoGIT), followed by normal-phase high-performance thin-layer chromatography (2D, NP-HPTLC) with multi-imaging under UV light (3D), white light illumination (4D, Vis), and fluorescence light detection (5D, FLD) plus effect-directed analysis (6D, EDA, +active). Dimensions 1D–6D were performed on the same planar surface. Active zones of interest were further evaluated after heart-cut elution and online desalting by reversed-phase high-performance liquid chromatography (7D, RP-HPLC) with diode array detection (8D, DAD) and high-resolution tandem mass spectrometry (9D/10D, HRMS/MS). This NP-HPTLC–nanoGIT+active–UV/Vis/FLD–EDA–heart cut–RP-HPLC–DAD–HRMS/MS hyphenation was used for non-target screening of highly processed foods, such as MRPs, for bioactive substances.
2. Materials and methods
2.1. Chemicals and materials
All solvents were chromatography grade, and all salts at least of p.a. quality unless stated otherwise. HPTLC silica gel 60 F254 MS-grade plates were obtained from Merck, Darmstadt, Germany. Disodium hydrogen phosphate (Na2HPO4, ≥99%), bovine serum albumin (BSA, fraction V, ≥98%), tris(hydroxymethyl)aminomethane (TRIS, ≥99.9%), hydrochloric acid (HCl, purest, 37%), glacial acetic acid (100%), n-hexane (≥98%), capric acid (>98%), stearic acid (>98%), and oleic acid (>99%) were purchased from Carl Roth, Karlsruhe, Germany. Bile extract (porcine), pancreatin from porcine pancreas (8 × USP specifications), acarbose (≥95%), rivastigmine (≥98%), caffeine (reagent plus), sodium acetate (>99%), ammonium acetate (≥99%), imidazole (≥99.5%), acetylcholinesterase (AChE) from Electrophorus electricus (≥245 U mg−1, 10 kU per vial), α-glucosidase from Saccharomyces cerevisiae (1000 U per vial), lauric acid (98%), myristic acid (≥99%), palmitic acid (>99%), linoleic acid (60–74%), 1-oleoyl-rac-glycerol (≥99%, monoacylglycerol, MAG), dioleoylglycerol (≥99%, diacylglycerol, DAG), glyceryl trioleate (≥99%, triacylglycerol, TAG), and ethanol (≥99.8%) were delivered by Fluka Sigma-Aldrich, Steinheim Germany. Butyrylcholinesterase (BChE, ≥245 U mg−1) from equine serum was obtained from SERVA, Heidelberg, Germany, and its substrate 1-naphthyl acetate (≥98%) from AppliChem, Darmstadt, Germany. 2-Naphtyl-α-D-glucopyranoside (99%) was from Fluorochem, Hadfield Derbyshire, UK. β-Glucosidase from almonds (3040 U mg−1) and 2-naphthyl-β-D-glucopyranoside (95%) were provided by ABCR, Karlsruhe, Germany. Dichloromethane was provided by Th. Geyer, Renningen, Germany. Methanol, water (MS-grade), and formic acid (99%) were from VWR, Darmstadt, Germany. n-Butanol, diethyl ether (99%), and linolenic acid (99%) were obtained from Acros Organics, Morris Plains, NJ, USA. Fast Blue B salt (95%) was provided by MP Biomedicals, Eschwege, Germany. The culture medium22 for the bioluminescent Aliivibrio fischeri bacteria (DSM-7151, German Collection of Microorganisms and Cell Cultures, Berlin, Germany) and the simulated intestinal fluid (SIF)23,24 are listed elsewhere. Rhodamine 6G (>97%) was provided by Alfa Aesar, Kandel, Germany. Double distilled water was prepared using a Heraeus Destamat Bi 18E from Thermo Fisher Scientific, Dreieich, Germany. Powdered MRPs in different flavours were purchased from yfood Labs, Munich, Germany.
2.2. Sample preparation and standard solutions
Each MRP was prepared according to the manufacturers’ instructions. Briefly, 29 g of each powder were solved in 100 mL double distilled water and homogenised by manual shaking. Then, 5 g of each liquid nutrient (Table S1‡) was mixed with 5 mL n-butanol, vortexed for 10 min, and ultrasonicated (Sonores Digiplus, Bandelin, Berlin, Germany) for 15 min. After centrifugation at 3000 × g for 5 min (Labofuge 400, Heraeus, Hanau, Germany), each upper phase was filtered through a 0.45 μm cellulose acetate filter (VWR, Darmstadt, Germany), and transferred to autosampler vials. Capric, lauric, myristic, palmitic, stearic, oleic, linoleic, and linolenic acids, and MAG, DAG, and TAG were prepared each as 10 mg mL−1 stock solutions in n-hexane and diluted to 1 mg mL−1 standard solutions.
2.3. NP-HPTLC–nanoGIT+active–UV/Vis/FLD–EDA workflow
The simulated intestinal fluid (SIF)23,24 and the NP-HPTLC–nanoGIT+active–UV/Vis/FLD–EDA workflow21 were prepared/performed as described. All HPTLC instruments controlled using visionCATS software (version 3.1.21109.3) were from CAMAG, Muttenz, Switzerland, if not stated otherwise. In brief, HPTLC silica gel 60 F254 MS-grade plates were prewashed twice with methanol–water (4
:
1, v/v) in a Simultaneous TLC Developing Chamber (Macherey-Nagel, Düren, Germany) and dried at 110 °C in a clean oven (Memmert, Schwabach, Germany) for 20 min.25 Plates were wrapped in aluminium foil and stored in a desiccator until use. n-Butanol extracts were applied twice as 6 mm bands (5 μL per band) on pre-washed plates with a track distance of 13.5 mm (Automated TLC Sampler 4). The positive control (PC) rapeseed oil (1 mg mL−1 in n-hexane, 5 μL) and the negative control (NC) pancreatin solution (panc, 20 mU μL−1 in SIF with bile extract, 5 μL) with respective co-factors (CaCl2, 6 pmol μL−1, 1 μL) were co-applied. The PC and one sample track each were oversprayed with panc and CaCl2. To simulate the on-surface digestion, the lower 2 cm of the plate were moistened with 0.1 M sodium chloride solution (1.25 mL, yellow nozzle, level 6). Thereby, the top 8 cm of the plate was covered with a second plate, so that exclusively the 2 cm lower application area was wetted.26 The stacked plates were placed horizontally in a moistened polypropylene box (KIS, 26.5 cm × 16 cm × 10 cm, ABM, Wolframs-Eschenbach, Germany) and incubated for 1 h at 37 °C in an oven (Memmert, Schwabach, Germany). The digested sample-loaded plate was dried for 4 min in a stream of cold air and developed with n-hexane–dichloromethane–methanol–water (40
:
50
:
10
:
1, v/v/v/v) up to 70 mm in a twin trough chamber. Note that the mobile phase consisted of almost 90% highly volatile organic solvents, i.e. n-hexane and dichloromethane, necessitating an absolutely tight development chamber. Documentation followed at Vis, UV 254 nm, and FLD 366 nm (TLC Visualizer 2). The selected α-/β-glucosidase and AChE/BChE inhibition assays as well as the A. fischeri bioassay were performed as previously described.15 The NP-HPTLC–nanoGIT+active–UV/Vis/FLD–EDA workflow took 2–3 h, depending on the assay. Each assay was repeated several times to confirm the observed responses. Functionality of each assay was proven by application (on the upper plate edge) of a respective PC, i.e. caffeine (1 mg mL−1 in methanol, 0.5, 1.5, and 3 μL per band) for A. fischeri bioassay as well as rivastigmine (0.1 mg mL−1 in methanol, 2, 4, and 8 μL per band) for AChE and BChE, acarbose (3 mg mL−1 in ethanol, 1, 3, and 6 μL per band) for α-glucosidase, and imidazole (1 mg mL−1, 3, 5, and 7 μL per band) for β-glucosidase inhibition assays.
2.4. Heart cut–RP-HPLC–DAD–HESI-HRMS/MS
In a previous study, a 10D workflow was established.21 All instrumental parameter settings concerning standalone pump, fully automated (open-source modified) autoTLC-MS interface,27 switching valve, desalting unit, HPLC, DAD and HESI-HRMS/MS were adopted. Shortly, bioactive zones were selected using the respective (bio)autogram, then automatically eluted from the plate by an oval elution head (2 mm × 4 mm), desalted by trapping on a pre-column and valve switching, and further characterized by RP-HPLC–DAD–HESI-HRMS/MS. The procedure took 12 min per zone.
3. Results and discussion
10D hyphenation is a powerful tool to maximize the information gained from a single run (Fig. 1).21 Pancreatic digestion in the GIT was minimalistically simulated on-surface of the HPTLC adsorbent. This simulated static digestion was performed on the humidified solid adsorbent particles. Due to this difference in in vitro digestion (in the liquid state), the miniaturized on-surface digestion24 was proven and successfully verified to provide similar results by comparison with the harmonized in vitro model23 for simulated static digestion. Information about digestible lipids, saccharides, and proteins present in the sample was obtained through lipolytic, amylolytic, and proteolytic activity. Exemplarily, 6 commercially available powdered MRPs of different flavours were studied. Having the same base formulation but different flavour additions, similarities and differences were expected in the chemical and effect-directed profiling. The side-by-side comparison of non-digested and digested sample in the NP-HPTLC chromatogram/bioautogram showed metabolic changes through digestion. Thus, tracking of metabolic activation or deactivation was embedded in the workflow. Evidence about substance polarity was given via the hRF value of a compound. Due to the different detection modes at Vis, UV 254 nm, and FLD 366 nm, chemical properties of sample components, such as chromophores, UV absorption, and natively fluorescent structural moieties, respectively, were evaluated. Subsequent EDA revealed antibacterial compounds against A. fischeri as well as inhibitors of α-glucosidase, β-glucosidase, AChE and BChE. Final heart cut–RP-HPLC–DAD–HRMS/MS analysis of the bioactive zone allowed further information/confirmation about polarity (retention time in the RP column), absorption (DAD), exact mass (HRMS), and fragmentation (HRMS/MS), which altogether facilitates the structure elucidation.
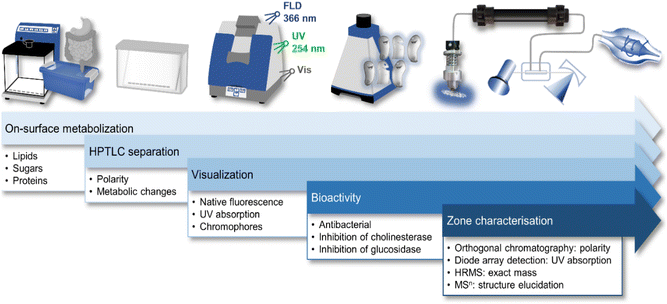 |
| Fig. 1 Schematic workflow of the 10D hyphenation NP-HPTLC–nanoGIT+active–UV/Vis/FLD–EDA–heart cut RP-HPLC–DAD–HESI-HRMS/MS and information obtained. | |
3.1. NP-HPTLC–nanoGIT+active–UV/Vis/FLD–EDA screening
Effect-directed profiling showed bioactive zones in all five assays (Fig. 2). Since the base formulation of the six differently flavoured MRPs is very similar or even the same (Tables S1 and S2‡), an observed similar effect-profile across the different MRPs pointed to active compounds which are constituents of the base formulation. Non-digested (−) and digested (+) samples were screened in parallel to visualize differences through pancreatic digestion. Bioactive zones of interest (marked I–XII) were further analysed via heart cut–RP-HPLC–DAD–HRMS/MS. Antibacterial effects against the Gram-negative A. fischeri bacteria were evident as dark zones on the bioluminescent background (depicted as the greyscale image, Fig. 2A). In contrast, compounds with a positive impact on the energetic cell metabolism are brighter than the background. Zone I almost migrated to the solvent front (hRF 99) and its bioactive response was hard to distinguish from the solvent front. The amount of antimicrobial substances increased through digestion (Fig. 2A), especially in the upper hRF region (hRF 60–90, zones II and III). This effect was so strong that the substances even diffused to the neighbouring tracks under aqueous bioassay conditions. This influence on the neighbouring tracks is avoided by increasing the distance between the sample tracks, but then fewer samples can be studied in parallel. Since this effect was also observed in the PC (digested rapeseed oil) and NC, albeit in an attenuated form, it was expected that the substances must be lipids. The same holds for the antibacterial zone at hRF 23 (zone X). Zones IV (hRF 50) and V (hRF 35) also exhibited antibacterial properties against A. fischeri. Both originated from metabolic digestion processes, since these were not detected/present in the non-digested samples. Only in this bioassay, zone IV could be clearly differentiated from the blurred zones II and III. Some compounds enhancing the energetic cell metabolism of the A. fischeri bacteria remained in the application zone. At least in the digested samples (+), this effect originated assumedly from the pancreatic enzyme salts, as it was also recognized in the NC. The non-digested (−) MRPs also showed bright zones, which could be explained by added salts. In any case, the brighter response in the application zone (hRF 0) must be caused by an MRP component of the base formulation, as it is ubiquitous in all flavour sorts. Remaining in the start zone indicated a weak interaction with the apolar mobile phase, while the polar stationary phase has a greater affinity. This fact made it possible to restrict the inquiring molecules to the polar components (Tables S1 and S2‡). Minor antibacterial effects were observed between hRF values ≤10 (zones XI and XII) in all samples, which were not affected by digestion.
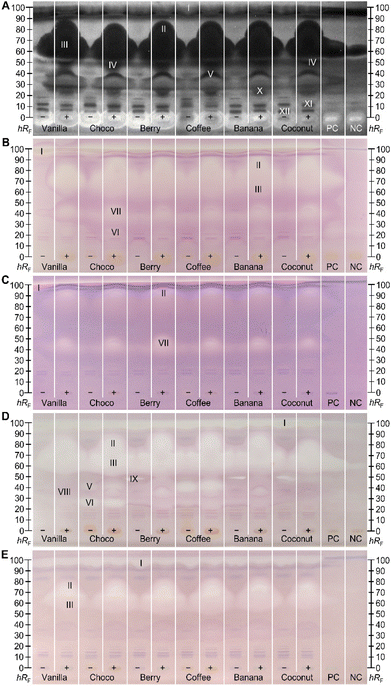 |
| Fig. 2 Side-by-side comparison of NP-HPTLC–nanoGIT+active profiles of six non-digested (−) and digested (+) MRPs (5 μL per band each, differently flavoured, assignments in Table S1‡) along with positive control (PC, digested rapeseed oil), and negative control (NC, pancreatic enzyme mix with bile salts), applied on HPTLC plate silica gel 60 F254 MS-grade, developed with n-hexane–dichloromethane–methanol–water (40 : 50 : 10 : 1, v/v/v/v) up to 70 mm and detected via bioluminescence after the A. fischeri bioassay (A, depicted as a greyscale image), and under white light illumination after the α-glucosidase (B), β-glucosidase (C), AChE (D), and BChE (E) inhibition assays. Heart-cut eluted bioactive zones (I–XII) were further characterized by RP-HPLC–DAD–HRMS/MS (Fig. 3). | |
Inhibitors of α- and β-glucosidase were evident as colourless zones on a purple background (Fig. 2B/C). They only differed in the inhibition intensity, which was apparently stronger towards α-glucosidase. The MRP ingredients are thus more potent in inhibiting the cleavage of α-1,4- or α-1,6-linked polysaccharides such as starch. In non-digested samples, a colourless inhibiting zone was detected within the solvent front (hRF 99, zone I), especially in the MRPs vanilla and choco. The chromatographic behaviour of this substance suggested that it has apolar properties since it did not interact with the polar silica gel plate. This substance was suspected to be completely digested, since it was not detected anymore in the digested (+) samples. Further inhibitory zones were detected at hRF 60–90 (zones II and III) in the A. fischeri bioautogram. Exclusively for the choco-flavoured MRP, another weak α-glucosidase inhibitory zone (VI, hRF 27) was determined which was also apparent in the AChE autogram (Fig. 2D). The latter proved no new metabolic changes through digestion that have not already been obvious for the α-glucosidase assay. At hRF 40–50, zone VII was solely observed in the glucosidase inhibition autograms (Fig. 2B/C). The intensity of the inhibitory zone increased through digestion in all samples.
The lipidic zones I–III in the cholinesterase inhibition profiles (Fig. 2D/E) were similar in response and intensity to the glucosidase inhibition profiles (Fig. 2B/C). This highlighted the influence of lipids on different metabolic processes. Screening for AChE inhibitors (Fig. 2D) revealed four further zones (V, VI, VIII, and IX). Zone VI (hRF 27) was predominantly active in the choco-flavoured and zone V (hRF 38) in coffee-flavoured MRPs, where both zones were not influenced by pancreatic digestion. These substances are assumed to originate from the sort-specific MRP ingredients (Table S1‡), i.e., fat-reduced cacao and coffee extract in choco- and coffee-flavoured MRPs, respectively. Zone VIII (hRF 37) was only apparent in the AChE inhibition assay for the digested samples except for coconut. Since there is no response at the same hRF value in non-digested analogues, it was suggested, that pancreatic digestion provided new AChE inhibition properties to metabolites. At hRF 48, a colourless zone (IX) was detectable in the non-digested fruit-based MRP berry, coffee, banana, and coconut, while this response was not present in the flavours vanilla and choco. After digestion, this effect was no longer detected. Modified chemical properties of the digestion product compared to its educt, resulted in either a change in the retention behavior on the NP-HPTLC plate or the loss of interaction with the binding site of the AChE. Since the BChE inhibition profiles (Fig. 2E) showed the same lipidic zones I–III as already observed through other (bio)assays, they were not further discussed in detail. However, it was striking that the signal intensities of zones I–III differed strongly between the (bio)assays. The weakest response was observed for β-glucosidase inhibition, while the inhibition intensity of BChE and α-glucosidase were very similar, and the AChE inhibition and antibacterial response (against Gram-negative A. fischeri) gave the strongest signals. The direct comparison is possible since the same sample amounts were applied.
3.2. Heart cut–RP-HPLC–DAD–HRMS/MS non-target screening results
Interesting active zones were selected for heart cut elution via their coordinates on the respective (bio)autogram. The fully automated TLC-MS interface27 moved to the selected position, lowered the elution head to isolate the zone, and eluted the compounds within directly from the (bio)autogram. This was performed as a sequence, i.e. one compound zone after the other was automatedly eluted and transferred to RP-HPLC–DAD–HRMS/MS. Most bioactive compounds identified were not explicitly listed in the ingredients list, but hidden behind super categories such as vegetable oils, coffee extract, or cacao, which hindered a direct assignment. The very apolar zone I (close to the solvent front, not so prominent) did not provide a mass signal in the heart cut–RP-HPLC–DAD–HESI-HRMS/MS analysis. It was assumed that triacylglycerols (TAGs) from animal fats and vegetable oils (Table S1‡) migrated to the solvent front (hRF 99) on the NP-HPTLC plate. This assumption was supported by the migration behaviour of lipid standards separated in a comparable chromatographic system (Fig. S1‡). The missing signals for TAGs originated from the setup of the 10D hyphenation. The zone elution solvent consists of 90% water and only 10% organic solvent. The aqueous solvent has good elution strength on NP-HPTLC plates, but solubility for TAGs is poor and thus the highly apolar TAGs could not be transferred from the plate to be detected by HRMS/MS. In addtion, the installed HESI ionization source is not suited for the ionisation of apolar compounds.
The most striking zones II–IV were detected in all assays. After heart cut–RP-HPLC–DAD–HESI-HRMS/MS, the benefit of the orthogonal separation was evident. Seven fatty acids (Table 1 and Fig. 3D) were detected at hRF 60–95. Three independent elutions were performed (zones II–IV) to cover the broad hRF range. All revealed comparable chromatograms but differed in the intensity of the fatty acids. It was expected that all fatty acids migrated to a similar (broad) hRF range in this chromatographic system.
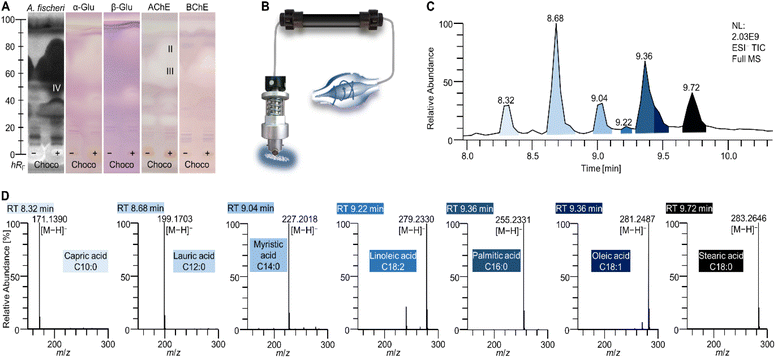 |
| Fig. 3 NP-HPTLC–nanoGIT+active–UV/Vis/FLD–EDA–heart cut–RP-HPLC–DAD–HRMS/MS results of zone III (zones II and IV showed a similar profile but with differing signal intensities). Profiles of the choco-flavoured MRP as in Fig. 2. (A). Zones II–IV were heart cut eluted, separated on an RP-HPLC column, and detected via orbitrap HRMS/MS (B, scheme) as a full scan HRMS chromatogram (C), and respective mass spectra indicating fatty acids (D). Related peaks and mass spectra are highlighted in the same colour. | |
Table 1 NP-HPTLC–nanoGIT+active–UV/Vis/FLD–EDA–heart cut–RP-HPLC–DAD–HESI-HRMS/MS signals of the bioactive zones I–XII (as in Fig. 2) and tentative HRMS signal assignments
Zone |
hR
F (±1) |
t
R [min] |
Molecular formula |
Ion species |
Precursor [m/z] |
Δm/z [ppm] |
MS2 [m/z] |
λ
max [nm] |
Tentative assignment |
Ref. |
hR
F: 100-fold retardation factor on NP-HPTLC, tR: retention time on RP-HPLC column, n.d.: not detected, sh: shoulder. |
I
|
99 |
n.d. |
II, III, IV |
60–95 |
8.32 |
C10H20O2 |
[M − H]− |
171.1390 |
0.49 |
No fragments |
— |
Capric acid |
28
|
8.68 |
C12H24O2 |
[M − H]− |
199.1703 |
0.42 |
No fragments |
— |
Lauric acid |
28
|
8.78 |
C18H34O3 |
[M − H]− |
297.2436 |
−0.24 |
No fragments |
— |
Unknown |
|
[M + Cl]− |
333.2204 |
−0.64 |
[M + HCOO]− |
343.2492 |
−0.47 |
9.00 |
C14H28O2 |
[M − H]− |
227.2019 |
−0.95 |
No fragments |
— |
Myristic acid |
29
|
[2M − H]− |
455.4112 |
−1.37 |
9.00 |
C18H30O2 |
[M − H]− |
277.2174 |
−0.49 |
No fragments |
— |
Linolenic acid |
29
|
9.21 |
C18H32O2 |
[M − H]− |
279.2330 |
−0.13 |
No fragments |
— |
Linoleic acid |
29
|
[2M − H]− |
559.4735 |
−0.65 |
9.43sh |
C16H32O2 |
[M − H]− |
255.2331 |
−0.65 |
No fragments |
— |
Palmitic acid |
29
|
[2M − H]− |
511.4734 |
−0.46 |
9.43 |
C18H34O2 |
[M − H]− |
281.2486 |
−0.09 |
No fragments |
— |
Oleic acid |
29
|
[2M − H]− |
563.5048 |
−0.49 |
9.68 |
C18H36O2 |
[M − H]− |
283.2644 |
−0.34 |
No fragments |
— |
Stearic acid |
29
|
[2M − H]− |
567.5364 |
−1.03 |
V
|
38 |
5.50 |
C8H10O2N4 |
[M + H]+ |
195.0879 |
−1.48 |
138.0664+ |
205, 275 |
Caffeine |
30
|
110.0718+ |
VI
|
27 |
4.45 |
C7H8O2N4 |
[M + H]+ |
181.0720 |
0.23 |
163.0615+ |
205, 275 |
Theobromine |
31
|
[M + NH4]+ |
198.0989 |
−1.81 |
138.0663+ |
[M + Na]+ |
203.0539 |
0.08 |
110.0717+ |
[M + K]+ |
219.0278 |
0.29 |
VII
|
48 |
6.63 |
C12H22O5 |
[M − H]− |
245.1395 |
−0.37 |
171.1028− |
— |
Unknown |
|
[M + Cl]− |
281.1164 |
−0.86 |
173.1168+ |
[M + HCOO]− |
291.1453 |
−1.31 |
155.1063+ |
[M + H3C − COO]− |
305.1609 |
−0.92 |
109.1012+ |
[M − H2O + H]+ |
229.1427 |
3.12 |
67.0549+ |
[M + NH4]+ |
264.1797 |
3.10 |
[M + Na]+ |
269.1349 |
4.04 |
[M + K]+ |
285.1089 |
3.62 |
7.37 |
C15H26O5 |
[M − H]− |
285.1710 |
−0.88 |
211.1337− |
— |
Unknown |
|
[M + Cl]− |
321.1476 |
−0.54 |
187.1337− |
[M + HCOO]− |
331.1765 |
−0.82 |
247.0965+ |
[M + H3C − COO]− |
345.1921 |
−0.64 |
215.0703+ |
[M − H2O + H]+ |
269.1746 |
0.51 |
187.0754+ |
[M + H]+ |
287.1852 |
0.53 |
[M + NH4]+ |
304.2117 |
0.49 |
[M + Na]+ |
309.1670 |
0.73 |
[M + K]+ |
325.1410 |
0.56 |
VIII
|
37 |
6.12 |
C16H31O7N |
[M − H]− |
348.2031 |
−0.95 |
274.1662− |
— |
Unknown |
|
[M + Cl]− |
384.1800 |
−1.36 |
226.1450− |
[M + HCOO]− |
394.2086 |
−0.97 |
208.1344− |
[M + H3C − COO]− |
408.2243 |
−1.03 |
104.0711+ |
[M + H]+ |
350.2174 |
−0.11 |
[M + Na]+ |
372.1994 |
−0.23 |
[M + K]+ |
388.1733 |
−0.18 |
IX
|
48 |
6.95 |
C10H11O4N |
[M − H]− |
208.0616 |
−0.18 |
151.0275− |
215, 275, 360 |
Unknown (fruit based powders) |
|
121.0295− |
X
|
23 |
8.07 |
C24H38O4 |
[M − H]− |
389.2702 |
−1.25 |
373.2741+ |
— |
Bile acid (7-ketolithocholic acid) |
|
[M + Cl]− |
425.2468 |
−1.01 |
355.2634+ |
[M + HCOO]− |
435.2756 |
−0.88 |
337.2517+ |
[M + H3C − COO]− |
449.2914 |
−1.10 |
319.2416+ |
[2M − H]− |
779.5474 |
−0.79 |
159.1170+ |
[2M + Na − 2H]− |
801.5300 |
−1.66 |
[2M + Cl]− |
815.5245 |
−1.32 |
[2M + HCOO]− |
825.5545 |
−2.70 |
[2M + H3C − COO]− |
839.5697 |
−2.20 |
[M − 2H2O + H]+ |
355.2634 |
−0.63 |
[M − H2O + H]+ |
373.2741 |
−0.99 |
[M + H]+ |
391.2847 |
−0.98 |
[M + NH4]+ |
408.3111 |
−0.75 |
[M + K]+ |
429.2405 |
−0.80 |
[2M + H]+ |
781.5623 |
−1.27 |
[2M + NH4]+ |
798.5888 |
−1.23 |
[2M + Na]+ |
803.5444 |
−1.48 |
[2M + K]+ |
819.5184 |
−1.44 |
XI
|
15 |
8.10, 8.46 |
C24H40O4 |
[M − H]− |
391.2859 |
−1.27 |
No fragments |
— |
Bile acids (hyodeoxycholic acid, ursodeoxycholic acid, chenodeoxycholic acid, deoxycholic acid) |
32
|
[M + Cl]− |
427.2623 |
−0.56 |
[M + HCOO]− |
437.2913 |
−1.02 |
[M + H3C − COO]− |
451.3070 |
−1.03 |
[2M−H]− |
783.5789 |
−1.14 |
[2M + Na − 2H]− |
805.5609 |
−1.09 |
[2M + Cl]− |
819.5561 |
−1.63 |
[M − 2H2O + H]+ |
357.2789 |
−0.35 |
[M − H2O + H]+ |
375.2894 |
0.06 |
[M + NH4]+ |
410.3266 |
−0.33 |
[M + Na]+ |
415.2817 |
0.41 |
[M + K]+ |
431.2558 |
0.06 |
[2M + H]+ |
785.5927 |
−0.13 |
[2M + NH4]+ |
802.6190 |
0.13 |
[2M + Na]+ |
807.5749 |
−0.40 |
[2M + K]+ |
823.5485 |
0.03 |
XII
|
7 |
5.73 |
C12H19O8Cl3 |
[M − H]− |
395.0072 |
0.20 |
359.0308− |
210 |
Sucralose (sweetener) |
33
|
[M + Cl]− |
430.9840 |
−0.19 |
323.0538− |
[M + HCOO]− |
441.0126 |
0.36 |
[M + H3C − COO]− |
455.0285 |
−0.20 |
[M + NH4]+ |
414.0488 |
−1.02 |
[M + K]+ |
434.9779 |
−0.46 |
This hypothesis was confirmed with respective co-developed standards and the A. fischeri bioassay (Fig. 4). Supposedly pure standards of unsaturated fatty acids, i.e. C18:1, C18:2, and C18:3, were prone to impurities and oxidative degradation.39 Lipolytic activity of pancreatic enzymes hydrolysed TAGs from milk protein and vegetable oils to their respective free fatty acids. Capric (C10:0) and lauric acid (C12:0) were cleavage products from animal fats,28 while myristic (C14:0), palmitic (C16:0), stearic (C18:0), oleic (C18:1), linoleic (C18:2), and linolenic acids (C18:3) resulted rather from sunflower and rapeseed oil.29 According to the nutritional value list of the MRPs (Table S2‡), the overall amount of fat is indicated as 18.2 ± 0.4 g per 300 mL shake, of which only 2.4 ± 0.1 g are saturated. C12:0 is reported to interrupt the membrane integrity of Gram-positive and Gram-negative bacteria, inducing its antibacterial effect.34 Weaker antimicrobial properties were described for the saturated C10:0, C16:0, and C18:0.34 The fatty acids, particularly n-3 (omega-3) polyunsaturated fatty acids, seem to alter the natural intestinal microbiome towards a balance between the main bacterial genera Firmicutes and Bacteroidetes.35,36 Fatty acids of the n-3 family are reported to exhibit AChE inhibitory activities.37 Of the detected fatty acids (Table 1), only C18:3 belongs to the n-3 family. Since the AChE inhibition signal was very broad, it was assumed that other fatty acids also contributed to the overall inhibitory response. In a previous study, standards of C14:0, C16:0, C18:0, and C18:1 were also found to inhibit AChE as well as BChE, although the inhibition of the latter was weaker.38 Further, α- and β-glucosidase inhibition properties for those four fatty acids were determined.38 The intense response of zones II–IV through all (bio)assays resulted from additive effects of the single fatty acids, which cannot be separated on NP-HPTLC plates (Fig. 4), but can be separated on the RP-HPLC column (Fig. 3). Furthermore, specific bioactive compounds were identified as caffeine (zone V, Table 1 and Fig. 2A/D) in coffee- and choco-flavoured MRPs, and theobromine (zone VI, Table 1 and Fig. 2B/D) in the choco-flavoured MRP. Both substances were not influenced by pancreatic digestion. Theobromine is known to have a stronger AChE inhibition (ca. 39%) compared to BChE (ca. 4%),31 confirming the presented assay results (Fig. 2D versus E). Inhibitory activity of caffeine against AChE in vivo was already proved by Souza et al.30 This confirmed that the on-surface methodology is suited to study highly efficient complex samples and preselect active compounds with potential impact in vivo.
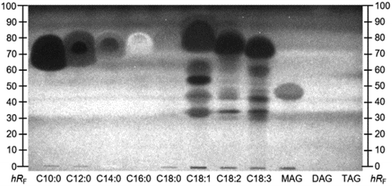 |
| Fig. 4 NP-HPTLC–Aliivibrio fischeri bioautogram of eight fatty acids (C10:0–C18:3), monoacylglycerol (MAG), diacylglycerol (DAG), and triacylglycerol (TAG) (5 μg per band each) applied on HPTLC plate silica gel 60 F254 MS-grade, developed with n-hexane–dichloromethane–methanol–water (40 : 50 : 10 : 1, v/v/v/v) up to 70 mm and A. fischeri bioluminescence depicted as a greyscale image. | |
The bioactive zones VII–IX (Fig. 2A–D) revealed HRMS mass signals that were used to calculate possible molecular formulae (Table 1), but were not assigned to individual substances. In zone VII, two polyphenols were assumed, while alkaloids were suspected in zones VIII and IX.
Zones X and XI in the A. fischeri bioautogram (Fig. 2A) were assigned to 7-ketolithocholic, hyodeoxycholic, ursodeoxycholic, chenodeoxycholic, or deoxycholic acids32 (Table 1) as constituents of the porcine bile salts added to the panc enzyme mixture. Since those antibacterial effects were not observed in the NC (only porcine bile salt, panc, and CaCl2), it was initially suspected that these antimicrobial effects originated from the MRPs, however, the identified compounds are not explainable by the list of ingredients. Based on the absence of any signal in the 7D–10D of the hyphenation, the substance exhibiting bioactivity against A. fischeri bacteria seems not to have any UV-absorption properties and not to be ionizable. Thus, it was not detectable by other means.
The isotope pattern of the unknown substance found in zone XII, indicated a trichlorinated compound. The precursor ion was obtained as a 27
:
27
:
9
:
1 quadruplet at m/z 395.0071, 397.0041, 399.0010, and 400.9976. HRMS signals revealed a molecular formula of C12H19O835Cl3 for m/z 395.0071. Further evidence of a trichlorinated compound is provided by the fragmentation spectrum, where two times chlorine split-off was observed (Table 1). According to spectral data and in accordance with the ingredients list, the sweetener sucralose was identified. Besides the antibacterial effect against A. fischeri (Fig. 2A), the synthetic sweetener has also been shown to have antimicrobial properties against other Gram-negative bacteria such as different Escherichia coli strains (HB101 and K-12).33 Further, sucralose was reported to alter the gut microbiome concerning the phyla Firmicutes and Bacteroidetes.33
4. Conclusions
The developed non-targeted 10D hyphenation provided comprehensive information and interesting comparative results for many samples in parallel in a single planar chromatographic separation. Since all important effective (known and unknown) compounds are detected and prioritized, it is an appropriate proactive tool with great potential to improve current food safety and consumer protection. It provided information about health-related bioactive ingredients and the activities of food constituents that are not directly listed as such on the packaging, as not regulated or required. Thus, health benefits of MRPs claimed by manufacturers were rapidly verified via the obtained bioactivity profiles. Additionally, information about bioavailability and bioactivity after simulated on-surface digestion of highly processed food was gained. Each comparative analysis of the original versus digested sample including effect-directed detection took 20–40 min, depending on the assay incubation time. The material costs were 2.90 Euro per digested and non-digested sample (calculated for 6 MRP samples analyzed in parallel on the same plate) plus 0.15 Euro per bioactive compound characterization (heart cut–RP-HPLC–DAD–HESI-HRMS/MS).
The demonstrated bioprofiling was exemplarily focused on detecting antibacterial compounds and the inhibitors of glucosidases and cholinesterases, but other effect mechanisms can be studied in the future. The data presented suggested that bioactive substances can be biologically stable and found in the MRPs despite the enormous mechanical, physical, and chemical processing or intestinal influences. The obtained results showed that MRPs and its digestion products inhibited the main enzymes involved in hyperglycaemia and cognitive decline, i.e. glucosidases and cholinesterases, respectively. Food products containing bioactive compounds that act against diabetes and Alzheimer's disease could make patients’ daily lives easier. Interestingly, plant foods, herbs and spices have been shown to be even richer and more versatile in bioactive compounds. The results obtained by the straightforward 10D hyphenation underline the famous quote by Hippocrates “Let food be thy medicine, and let medicine be thy food”, knowing that food is silently acting day after day in a low dose.
Abbreviations
AChE | Acetylcholinesterase |
BChE | Butyrylcholinesterase |
C10:0 | Capric acid |
C12:0 | Lauric acid |
C14:0 | Myristic acid |
C16:0 | Palmitic acid |
C18:0 | Stearic acid |
C18:1 | Oleic acid |
C18:2 | Linoleic acid |
C18:3 | Linolenic acid |
DAD | Diode array detection |
DAG | Diacylglycerol |
EDA | Effect-directed analysis |
FLD | Fluorescence light detection |
GIT | Gastrointestinal tract |
HPLC | High-performance liquid chromatography |
HPTLC | High-performance thin-layer chromatography |
HRMS | High-resolution mass spectrometry |
MAG | Monoacylglycerol |
MRP | Meal replacement product |
NC | Negative control |
NP | Normal phase |
panc | Porcine pancreatin |
PC | Positive control |
RP | Reversed phase |
sh | Shoulder |
SIF | Simulated intestinal fluid |
TAG | Triacylglycerol |
UV | Ultraviolet |
Vis | Visible light (white light detection) |
Author contributions
Tamara Schreiner: conceptualization, methodology, investigation, and writing – original draft. Naila M. Eggerstorfer: investigation. Gertrud E. Morlock: conceptualization, methodology, supervision, funding acquisition, and writing – review and editing.
Conflicts of interest
There are no conflicts to declare.
Acknowledgements
We thank Merck, Darmstadt, for supply of plates. Instrumentation was partially funded by the Deutsche Forschungsgemeinschaft (INST 162/536-1 FUGG and INST 162/471-1 FUGG).
References
- L. Sammugam and V. R. Pasupuleti, Balanced diets in food systems: Emerging trends and challenges for human health, Crit. Rev. Food Sci. Nutr., 2019, 59, 2746–2759 CrossRef PubMed
.
- M. I. Gómez, C. B. Barrett, T. Raney, P. Pinstrup-Andersen, J. Meerman, A. Croppenstedt, B. Carisma and B. Thompson, Post-green revolution food systems and the triple burden of malnutrition, Food Policy, 2013, 42, 129–138 CrossRef
.
- J. M. Poti, M. A. Mendez, S. W. Ng and B. M. Popkin, Is the degree of food processing and convenience linked with the nutritional quality of foods purchased by US households?, Am. J. Clin. Nutr., 2015, 101, 1251–1262 CrossRef CAS PubMed
.
- A. E. Mohr, C. Ramos, K. Tavarez and P. J. Arciero, Lower Postprandial Thermogenic Response to an Unprocessed Whole Food Meal Compared to an Iso-Energetic/Macronutrient Meal Replacement in Young Women: A Single-Blind Randomized Cross-Over Trial, Nutrients, 2020, 12, 2469 CrossRef CAS PubMed
.
- Y. Li, D. D. Wang, S. H. Ley, M. Vasanti, A. G. Howard, Y. He and F. B. Hu, Time Trends of Dietary and Lifestyle Factors and Their Potential Impact on Diabetes Burden in China, Diabetes Care, 2017, 40, 1685–1694 CrossRef PubMed
.
- L. A. Moreno, G. Rodriguez, J. Fleta, M. Bueno-Lozano, A. Lazaro and G. Bueno, Trends of dietary habits in adolescents, Crit. Rev. Food Sci. Nutr., 2010, 50, 106–112 CrossRef PubMed
.
- R. R. Briefel and C. L. Johnson, Secular trends in dietary intake in the United States, Annu. Rev. Nutr., 2004, 24, 401–431 CrossRef CAS PubMed
.
- B. Vellas, S. Lauque, S. Gillette-Guyonnet, S. Andrieu, F. Cortes, F. Nourhashémi, C. Cantet, P. J. Ousset and H. Grandjean, Impact of nutritional status on the evolution of Alzheimer's disease and on response to acetylcholinesterase inhibitor treatment, J. Nutr., Health Aging, 2005, 9, 75–80 CAS
.
- S. M. de La Monte and M. Tong, Mechanisms of nitrosamine-mediated neurodegeneration: potential relevance to sporadic Alzheimer's disease, J. Alzheimer's Dis., 2009, 17, 817–825 CAS
.
-
S. Sommano, in Advances in food science and nutrition, ed. P. M. Visakh, et al., John Wiley & Sons, Hoboken, NJ, 2014, pp. 361–390 Search PubMed
.
- J. Peng, J. Lu, X. Ma, L. Ying, W. Lu, W. Zhu, Y. Bao and J. Zhou, Breakfast replacement with a liquid formula improves glycaemic variability in patients with type 2 diabetes: a randomised clinical trial, Br. J. Nutr., 2019, 121, 560–566 CrossRef CAS PubMed
.
- I. Yip, V. L. Go, S. DeShields, P. Saltsman, M. Bellman, G. Thames, S. Murray, H. J. Wang, R. Elashoff and D. Heber, Liquid meal replacements and glycemic control in obese type 2 diabetes patients, Obes. Res., 2001, 9(Suppl 4), 341–347 CrossRef PubMed
.
- C. Hartmann, C. Keller and M. Siegrist, Compensatory beliefs, nutrition knowledge and eating styles of users and non-users of meal replacement products, Appetite, 2016, 105, 775–781 CrossRef PubMed
.
- D. Q. Rothacker, B. A. Staniszewski and P. K. Ellis, Liquid Meal Replacement vs Traditional Food: A Potential Model for Women Who Cannot Maintain Eating Habit Change, J. Am. Diet. Assoc., 2001, 101, 345–347 CrossRef CAS PubMed
.
- T. Schreiner, D. Sauter, M. Friz, J. Heil and G. E. Morlock, Is Our Natural Food Our Homeostasis? Array of a Thousand Effect-Directed Profiles of 68 Herbs and Spices, Front. Pharmacol., 2021, 12, 755941 CrossRef CAS PubMed
.
-
N. Bollmann and B. Kremer, The story behind yfood, https://uk.yfood.eu/pages/story, Accessed July 4, 2022.
- J. Correa-Betanzo, E. Allen-Vercoe, J. McDonald, K. Schroeter, M. Corredig and G. Paliyath, Stability and biological activity of wild blueberry (Vaccinium
angustifolium) polyphenols during simulated in vitro gastrointestinal digestion, Food Chem., 2014, 165, 522–531 CrossRef CAS PubMed
.
- S. Ketnawa, J. Suwannachot and Y. Ogawa, In vitro gastrointestinal digestion of crisphead lettuce: Changes in bioactive compounds and antioxidant potential, Food Chem., 2020, 311, 125885 CrossRef CAS PubMed
.
- X. Liu, J. Shi, J. Yi, X. Zhang, Q. Ma and S. Cai, The effect of in vitro simulated gastrointestinal digestion on phenolic bioaccessibility and bioactivities of Prinsepia utilis Royle fruits, LWT–Food Sci. Technol., 2021, 138, 110782 CrossRef CAS
.
- X. C. Sollano-Mendieta, O. G. Meza-Márquez, G. Osorio-Revilla and D. I. Téllez-Medina, Effect of In Vitro Digestion on the Antioxidant Compounds and Antioxidant Capacity of 12 Plum (Spondias purpurea L.) Ecotypes, Foods, 2021, 10, 1995 CrossRef CAS PubMed
.
-
T. Schreiner, N. M. Eggerstorfer and G. E. Morlock, Effects of gastrointestinal digestion on the bioactivity of convenience tomato products examined by ten-dimensional hyphenation, in submission.
-
European Committee for Standardization, Water Quality - Determination of the Inhibitory Effect of Water Samples on the Light Emission of Vibrio Fischeri (Luminescent Bacteria Test), 2009 Search PubMed
.
- M. Minekus, M. Alminger, P. Alvito, S. Ballance, T. Bohn, C. Bourlieu, F. Carrière, R. Boutrou, M. Corredig, D. Dupont, C. Dufour, L. Egger, M. Golding, S. Karakaya, B. Kirkhus, S. Le Feunteun, U. Lesmes, A. Macierzanka, A. Mackie, S. Marze, D. J. McClements, O. Ménard, I. Recio, C. N. Santos, R. P. Singh, G. E. Vegarud, M. S. J. Wickham, W. Weitschies and A. Brodkorb, A standardised static in vitro digestion method suitable for food - an international consensus, Food Funct., 2014, 5, 1113–1124 RSC
.
- G. E. Morlock, L. Drotleff and S. Brinkmann, Miniaturized all-in-one nanoGIT+active system for on-surface metabolization, separation and effect imaging, Anal. Chim. Acta, 2021, 1154, 338307 CrossRef CAS PubMed
.
- G. E. Morlock, Background Mass Signals in TLC/HPTLC–ESI-MS and Practical Advices for Use of the TLC-MS Interface, J. Liq. Chromatogr. Relat. Technol., 2014, 37, 2892–2914 CrossRef CAS
.
-
I. Müller and G. E. Morlock, Validation and quantification of the saccharide release of hydrothermally treated flours after salivary and pancreatic amylolysis by the HPTLC nanoGIT+active method, in submission.
- A. Mehl, W. Schwack and G. E. Morlock, On-surface autosampling for liquid chromatography-mass spectrometry, J. Chromatogr. A, 2021, 1651, 462334 CrossRef CAS PubMed
.
- S. Gallier, J. Cui, T. D. Olson, S. M. Rutherfurd, A. Ye, P. J. Moughan and H. Singh, In vivo digestion of bovine milk fat globules: effect of processing and interfacial structural changes. I. Gastric digestion, Food Chem., 2013, 141, 3273–3281 CrossRef CAS PubMed
.
- J. Orsavova, L. Misurcova, J. V. Ambrozova, R. Vicha and J. Mlcek, Fatty Acids Composition of Vegetable Oils and Its Contribution to Dietary Energy Intake and Dependence of Cardiovascular Mortality on Dietary Intake of Fatty Acids, Int. J. Mol. Sci., 2015, 16, 12871–12890 CrossRef CAS PubMed
.
- A. C. Souza, A. Souza, L. F. Medeiros, C. de Oliveira, V. L. Scarabelot, R. S. Da Silva, M. R. Bogo, K. M. Capiotti, L. W. Kist, C. D. Bonan, W. Caumo and I. L. S. Torres, Maternal caffeine exposure alters neuromotor development and hippocampus acetylcholinesterase activity in rat offspring, Brain Res., 2015, 1595, 10–18 CrossRef CAS PubMed
.
- G. Brunhofer, A. Fallarero, D. Karlsson, A. Batista-Gonzalez, P. Shinde, C. Gopi Mohan and P. Vuorela, Exploration of natural compounds as sources of new bifunctional scaffolds targeting cholinesterases and beta amyloid aggregation: the case of chelerythrine, Bioorg. Med. Chem., 2012, 20, 6669–6679 CrossRef CAS PubMed
.
- X. Qiao, M. Ye, D. Pan, W. Miao, C. Xiang, J. Han and D. Guo, Differentiation of various traditional Chinese medicines derived from animal bile and gallstone: simultaneous determination of bile acids by liquid chromatography coupled with triple quadrupole mass spectrometry, J. Chromatogr. A, 2011, 1218, 107–117 CrossRef CAS PubMed
.
- Q.-P. Wang, D. Browman, H. Herzog and G. G. Neely, Non-nutritive sweeteners possess a bacteriostatic effect and alter gut microbiota in mice, PLoS One, 2018, 13, e0199080 CrossRef PubMed
.
- G. Casillas-Vargas, C. Ocasio-Malavé, S. Medina, C. Morales-Guzmán, R. G. Del Valle, N. M. Carballeira and D. J. Sanabria-Ríos, Antibacterial fatty acids: An update of possible mechanisms of action and implications in the development of the next-generation of antibacterial agents, Prog. Lipid Res., 2021, 82, 101093 CrossRef CAS PubMed
.
- L. Costantini, R. Molinari, B. Farinon and N. Merendino, Impact of Omega-3 Fatty Acids on the Gut Microbiota, Int. J. Mol. Sci., 2017, 18, 2645 CrossRef PubMed
.
- M. Balfegó, S. Canivell, F. A. Hanzu, A. Sala-Vila, M. Martínez-Medina, S. Murillo, T. Mur, E. G. Ruano, F. Linares, N. Porras, S. Valladares, M. Fontalba, E. Roura, A. Novials, C. Hernández, G. Aranda, A. Sisó-Almirall, G. Rojo-Martínez, R. Simó and R. Gomis, Effects of sardine-enriched diet on metabolic control, inflammation and gut microbiota in drug-naïve patients with type 2 diabetes: a pilot randomized trial, Lipids Health Dis., 2016, 15, 78 CrossRef PubMed
.
- A. I. Zugno, H. Chipindo, L. Canever, J. Budni, A. Alves de Castro, M. Bittencourt de Oliveira, A. S. Heylmann, P. Gomes Wessler, F. da Rosa Silveira, L. S. Damázio, G. A. Mastella, L. W. Kist, M. R. Bogo, J. Quevedo and C. S. Gama, Omega-3 fatty acids prevent the ketamine-induced increase in acetylcholinesterase activity in an animal model of schizophrenia, Life Sci., 2015, 121, 65–69 CrossRef CAS PubMed
.
- N. G. A. S. S. Chandana and G. E. Morlock, Eight different bioactivity profiles of 40 cinnamons by multi-imaging planar chromatography hyphenated with effect-directed assays and high-resolution mass spectrometry, Food Chem., 2021, 357, 129135 CrossRef PubMed
.
- G. E. Morlock and D. Meyer, Designed genotoxicity profiling detects genotoxic compounds in staple food such as healthy oils, Food Chem., 2023 Search PubMed
, in revision.
Footnotes |
† Dedicated to the lifework of Justus von Liebig, Professor in Gießen, on the occasion of his 150th death anniversary. |
‡ Electronic supplementary information (ESI) available: Details of samples (Table S1); the nutritional values of samples (Table S2); the migration behaviour of lipidic standards (Fig. S1). See DOI: https://doi.org/10.1039/d2fo02610d |
|
This journal is © The Royal Society of Chemistry 2023 |
Click here to see how this site uses Cookies. View our privacy policy here.