DOI:
10.1039/D2FO03201E
(Paper)
Food Funct., 2023,
14, 292-304
Anti-obesity effects of red pepper (Capsicum annuum L.) leaf extract on 3T3-L1 preadipocytes and high fat diet-fed mice†
Received
24th October 2022
, Accepted 23rd November 2022
First published on 24th November 2022
Abstract
Patients with obesity mostly have metabolic syndrome and this can lead to multiple health problems. In the present study, we evaluated the anti-obesity effect of water-soluble red pepper (Capsicum annuum L.) leaf extract (PLE) on 3T3-L1 adipocytes and high-fat diet (HFD)-fed mice. The adipocyte lipid content was determined using Oil Red O staining, which revealed that 100 μg mL−1 PLE markedly reduced fat accumulation without affecting the cell viability. PLE exhibited high prebiotic activity scores by modulating probiotic strains, contributing to host health improvement. In vivo investigation in HFD-fed mice revealed that PLE supplementation significantly decreased the HFD-induced increases in the body weight, amount of white adipose tissue, and serum triglyceride, total cholesterol, leptin, and insulin levels. Consistent with its effects on reduced lipid droplet formation in the liver, PLE supplementation suppressed the expression of lipid synthesis-related proteins including SREBP-1, FAS, and PPAR-γ in the liver and increased that of PGC-1α, CPT1, and adiponectin in epididymal WAT. PLE treatment improved intestinal barrier function and inflammation and reduced harmful intestinal enzyme activities in the feces. Collectively, these results indicate that PLE inhibits fat accumulation in HFD-fed mice via the suppression of adipogenesis and lipogenesis, suggesting its potential in preventing obesity.
1. Introduction
Obesity, defined as abnormal or excessive fat accumulation, has become a worldwide health concern leading to an increased risk of many diseases, such as hyperlipidemia, hypertension, type 2 diabetes mellitus, coronary heart disease, and cancer, and their complications.1 Consumption of a high-fat diet (HFD) is closely related to multiple metabolic diseases associated with obesity. Adipose tissues are responsible for energy storage, which depends on processes managing the size and number of adipocytes. During adipocyte differentiation, an increase in the number of adipocytes accounts for the adipose tissue expansion observed in obesity.2 Thus, several prevention options are available for the treatment of obesity. Losing weight by restricting calorie intake and engaging in physical exercise are the most common treatments. However, the use of weight-loss medication may have side effects. Therefore, there is a need for identifying suitable methods for the management of obesity for each individual.3
In recent years, the demand for numerous natural products, including crude extracts and compounds or phytochemicals isolated from plants, has increased because of their beneficial role in the treatment and prevention of diet-induced obesity.4 Red pepper (Capsicum annuum L.) is the most popular spice in Southeast Asia, Africa, and the Mediterranean region. It has been used as a food coloring pigment and hot flavoring agent and in traditional medicines.5 Pepper is a vegetable containing various carotenoid pigments, including β-carotene with pro-vitamin A activity, and a high level of bioactive compounds including carotenoids (capsanthin, capsorubin, cryptocapsin, and capsaicinoid), quercetin, and luteolin, many of which have been reported to have antioxidant properties.6 Pepper fruits consist of a stem, pericarp, placenta, and seeds. High levels of capsaicin, which has been reported to reduce oxidative damage by activating anti-oxidative defense systems, lowering cholesterol levels, improving blood lipid profiles, and decreasing the serum triacylglycerol content by enhancing energy metabolism, are present in the placenta.7
Numerous studies evaluated the antioxidant, anti-obesity, and anti-diabetic effects of pepper fruits including seed extracts. However, studies on pepper leaves have not yet been reported. Therefore, we hypothesized that water-soluble pepper leaf extract (PLE) might have prebiotic activity and anti-obesity effects and improve obesity and the related metabolic syndrome in a HFD-induced obesity mouse model.
2. Materials and methods
2.1. Preparation of PLE
Dried C. annuum L. leaves were collected from Wanju-gun, Republic of Korea, and ground into a fine powder (≤10 mm). The powder (100 g) was extracted with distilled water (2 L) at 80 °C for 3 h, at a ratio of 1
:
20 (w/v). Then, it was filtered and concentrated at 40 °C using a vacuum rotary evaporator, and then, lyophilized to obtain PLE. PLE has a color of light brown and highly water-soluble properties up to 200 mg mL−1.
2.2. UPLC-qTOF-MS analysis
Chemical profiling of PLE was performed using an ultra-performance liquid chromatograph coupled with a Waters Synapt G2-Si quadrupole time-of-flight mass spectrometer (UPLC–qTOF-MS, Waters, Manchester, UK). Separation was performed by gradient elution using a Waters Acquity® HSS T3 C18 column (2.1 × 50 mm, 1.8 μm), and the column oven temperature was set as 35 °C. The mobile phase was 0.1% formic acid in water (A) and 0.1% formic acid in acetonitrile (B) and delivered at 0.5 mL min−1 according to the following linear gradient: 0–1 min, 5% B; 1–20 min, 5–100% B; 20–22.3 min, 100% B; 22.3–22.4 min, 100–5% B; 22.4–25 min, 5% B. The injection volume was 2 μL. The mass spectra were acquired in the negative ion mode by scanning from m/z 55–1100. The optimized MS parameters were as follows: sampling cone, 18 V; collision energy, 5 kV; capillary, 1 kV; source temperature, 110 °C; desolvation gas flow, 780 L h−1; desolvation temperature, 350 °C. Data analysis was performed using Waters UNIFI software.
2.3.
In vitro assays
2.3.1. Cell culture, viability, and adipocyte differentiation.
Mouse 3T3-L1 preadipocytes were obtained from the American Type Culture Collection (ATCC, Manassas, VA, USA) and cultured in Dulbecco's modified Eagle's medium (DMEM; HyClone Laboratories, Helsinki, Finland) supplemented with 10% bovine calf serum (BCS; Gibco/BRL, Gaithersburg, MD, USA), 100 U mL−1 penicillin, and 100 μg mL−1 streptomycin incubated at 37 °C and under 5% CO2. Cell viability was analyzed using a Cell Counting Kit-8 assay (CCK-8; Dojindo, Tokyo, Japan), according to the manufacturer's protocol. 3T3-L1 preadipocytes were seeded in 96-well plates (5 × 104 cells per well) and allowed to reach confluence for 48 h. Upon confluence, the media were changed and replaced with differentiation cocktail (MDI, 0.5 mM 3-isobutyl-1-methylxanthine, 1 μM dexamethasone, and 10 μg mL−1 insulin) in DMEM supplemented with 10% fetal bovine serum (FBS; Gibco/BRL) for 48 h. The preadipocytes were maintained and re-fed every 48 h with 10% BCS-DMEM medium. To examine the effect of PLE on adipocyte differentiation, the preadipocytes received 50 and 100 μg ml−1 PLE (in DMEM medium) every two days, starting at two days post confluence until the eight day of culture. PLE solution was prepared at a concentration of 50 and 100 μg mL−1 and filtered using a 0.2 μm PVDF filter. Garcinia gummi-gutta (GG) extract used in commercial functional food as an ingredient was obtained from Novarex Inc. (Ochang, South Korea) and used as a positive control.
2.3.2. Measurement of lipid accumulation and the adiponectin concentration.
The effect of PLE on intracellular lipid accumulation was determined using Oil Red O (ORO) staining. After the removal of culture media, cells were washed twice with PBS, fixed in 10% formalin, and stained with ORO solution for 30 min. The stained lipid droplets were photographed using an inverted microscope (Eclipse Ti-U, Nikon, Tokyo, Japan). To quantify the lipid accumulation, lipid and ORO were dissolved in isopropanol and the absorbance was measured using a microplate reader (Spectra MAX M2; Molecular Device, Sunnyvale, CA, USA) at 520 nm.
The adiponectin contents were analyzed using the Mouse Adiponectin/Acrp30 Quantikine ELISA Kit (R&D Systems, Inc., Minneapolis, MN, USA) according to the manufacturer's protocol.
2.4. Prebiotic activity score
2.4.1. Probiotic bacteria and microbial culture.
Commercial probiotics isolated from the human intestine, Lacticaseibacillus paracasei (MG310), Bifidobacterium bifidum (MG731), and B. longum (MG723) were obtained from Mediogen (Jecheon, Republic of Korea); Escherichia coli cells (KCTC2441) were purchased from the Korean Collection for Type Cultures (Jeongeup, Republic of Korea), and Lactococcus lactis (KF140) was isolated from fermented kimchi at the Korea Food Research Institute. Lacticaseibacillus and Lactococcus strains were activated in MRS (de Man, Rogosa, and Sharpe) agar and MRS broth, and E. coli was activated in tryptic soy agar and tryptic soy broth at 37 °C for 24 h. The Bifidobacterium strains were activated in BL (blood and liver) agar and BL broth anaerobically at 37 °C for 48 h. The broth and agar were purchased from BD Biosciences (Franklin Lakes, NJ, USA). After activation in the broth, the bacterial strains were centrifuged (1000g, 5 min), and then suspended in M9 broth supplemented with 0.1% glucose, 0.0015% CaCl2, and 0.05% MgSO4. An optical density of 2.5 ± 0.01 at 600 nm was determined using a spectrophotometer (Jenway 6715, Cole-Parmer Ltd, Vernon Hills, IL, USA).
2.4.2. Determination of the prebiotic activity score.
The prebiotic activity score shows the ability of the substrate to support and sustain the growth of beneficial bacteria, such as Lactobacillus spp. and Bifidobacterium spp., and not those of enteric bacteria, such as E. coli. The prebiotic activity score is also related to the growth levels of organisms in glucose (non-prebiotic substrate). Inulin (commercial prebiotic) was used as a positive control. The prebiotic activity score was determined using a previously reported method.8 Diluted bacterial cultures were inoculated at a concentration of 1% (v/v) into respective tubes containing M9 medium supplemented with prebiotics (inulin and PLE) or glucose. Bacterial cultures with adjusted absorbance inoculated with 0.5% (w/v) glucose, 0.5% (w/v) inulin, or 0.1% (w/v) and 0.5% (w/v) PLE dissolved in M9 broth were incubated for 24 h at 37 °C. The prebiotic activity score was calculated using the following equation:
The pH of the fermented broth was determined using a pH meter (Orion Star A211, Thermo Fisher Scientific).
2.5.
In vivo assays
2.5.1. Mice and diets.
Six-week-old male C57BL/6J mice were procured from Central Laboratory Animal Inc. (Seoul, Korea) and the experiments were approved by the Korea Food Research Institutional Animal Care and Use Committee (KFRI-M-18027). The mice were kept under controlled conditions (22 ± 2 °C temperature, 55% humidity, and 12 h light/dark cycle). After 1 week of acclimation, the mice were housed in groups of three mice per cage and separated into five groups (n = 9 per group) as follows; AIN-93G diet (Dyets, Bethlehem, PA, USA), normal control (NC), high-fat diet (HFD) (60% kcal fat diet, TD.06414, Harlan, Madison, WI, USA), HFD supplemented with 50 mg kg−1G. gummi-gutta extract (GG), and HFD supplemented with 50 mg kg−1 PLE (PLE50) or 100 mg kg−1 PLE (PLE100). GG was used as a positive control. GG and PLE were dissolved in water and administered orally for 8 weeks. Body weight and food intake were measured each week.
At the end of the 8-week treatment period, mice were fasted for 12 h, and then anesthetized with isoflurane and sacrificed. Blood samples were subsequently taken from the abdominal vena cava for serum biomarker analysis. The epididymal white adipose tissue (eWAT) and liver were excised immediately, rinsed with 0.9% saline, and weighed. All samples were stored at −80 °C until analysis.
2.5.2. Histological analysis.
Histological analyses were performed using the ORO staining method9 with slight modifications. The tissues were immediately fixed in 10% formalin (Sigma-Aldrich, St Louis, MO, USA), after paraffin embedding, and 6 μm-thick sections were prepared and stained with ORO for the evaluation of lipid accumulation in the liver. In addition, paraffin-embedded eWAT was stained with the hematoxylin and eosin (H&E) stain. Images were obtained using an optical microscope (Axioskop 50; Carl Zeiss, Jena, Germany), at ×400 magnification. The adipocyte area (9–10 adipocytes per mouse) was analyzed using ImageJ software (NIH, Bethesda, MD, USA).
2.5.3. Blood serum and biochemical analysis.
After 8 weeks, the animals were fasted for 12 h, anesthetized with isoflurane, and then euthanized. Blood samples were collected from the abdominal vena cava and centrifuged at 3000g for 10 min at 4 °C. The serum samples were analyzed for triglyceride (TG) and total cholesterol (TC) levels using a commercial enzyme-linked immunosorbent assay (ELISA) kit (Cell Biolabs, Inc., Beverly, MA, USA). The leptin, insulin, adiponectin, and leukotriene B4 (LTB4) levels were determined using a commercial kit from R&D Systems (Minneapolis, MN, USA). The serum lipopolysaccharide (LPS) levels were determined using the Pierce™ LAL Chromogenic Endotoxin Quantitation Kit (Thermo Fisher Scientific, Waltham, MA, USA), according to the manufacturer's protocols. Hepatic malonyl-CoA was tested using the mouse malonyl coenzyme A ELISA kit (MyBioSource, San Diego, CA, USA) according to the manufacturer's instructions. Liver tissues (100 mg) were homogenized in 1× PBS and the homogenates were centrifuged (5000g, 5 min, 4 °C). The supernatant was obtained and the absorbance was measured at 450 nm. The hepatic malonyl-CoA level was expressed as optical density per mg protein.
2.5.4. Intestinal permeability analysis and the colon pH measurement.
Intestinal permeability was evaluated using the fluorescein isothiocyanate (FITC)-dextran method at 8 weeks. The mice were fasted for 6 h and FITC-dextran (FD4; 3000–5000 Da; Sigma-Aldrich) (400 mg per kg body weight) was administered orally at a single dose. After 1, 2, and 4 h, blood was collected from the tail vein and centrifuged (12
000g, 5 min, 4 °C). Fluorescence was measured using a microplate reader (Spectra MAX M2) in 96-well plates (excitation: 485 nm, emission: 535 nm). A standard curve was created by diluting FITC-dextran in untreated plasma diluted with phosphate-buffered saline.
Colon pH was determined using a pH meter (Orion star A211, Thermo Fisher Scientific) after emptying the colon with sterile water.
2.5.5. Myeloperoxidase (MPO) activity and fecal enzyme activity of the intestinal microflora.
MPO activity in the colonic mucosa and fecal enzyme activities were measured as previously described.10 Briefly, approximately 200 mg of the fresh colon was homogenized in ice-cold 50 mM hexadecyltrimethyl ammonium bromide buffer. The suspensions were kept at −70 °C for 3 h and then centrifuged (12
000g, 15 min, 4 °C). This process was repeated thrice, and 100 μL of the supernatant was mixed with 400 μL of 50 mM sodium phosphate buffer containing 0.129 mg mL−1O-dianisidine and 0.0005% H2O2. The absorbance was measured at 492 nm using a microplate reader (Spectra MAX M2). MPO activity was expressed as optical density per mg protein.
The activities of β-glucosidase and β-glucuronidase in the fecal samples were determined based on the rates of p-nitrophenol release. The activity of tryptophanase was measured at 550 nm with indole as a standard.
2.5.6 Western blot analysis.
Total protein was extracted from the liver and eWAT using PRO-PREP™ (iNtRON Biotechnology, Seongnam, Republic of Korea). The protein concentration was determined using a protein assay kit (Bio-Rad, Hercules, CA, USA). Protein (20 μg) was separated using SDS-PAGE on a 4–20% polyacrylamide gel and transferred to polyvinylidene difluoride membranes. The membrane was blocked with 5% skimmed milk in Tris-buffered saline containing 0.1% Tween-20 (TBST), and incubated overnight at 4 °C with primary antibodies against sterol regulatory element binding protein 1 (SREBP1; ab191857), fatty acid synthase (FAS; ab22759), acetyl-CoA carboxylase (ACC; ab72046), peroxisome proliferator-activated receptor-γ (PPAR-γ; ab59256), PPAR-α (ab215270), PPAR-γ coactivator-1α (PGC-1α; ab54481), adiponectin (ab22554), and β-actin (ab8224) purchased from Abcam (Cambridge, MA, USA), carbohydrate-responsive element binding protein (ChREBP; 58069) purchased from Cell Signaling Technology (Beverly, MA, USA), and carnitine palmitoyltransferase 1 (CPT1; sc-393070) purchased from Santa Cruz Biotechnology (Dallas, TX, USA). The membranes were incubated for 1 h at room temperature with secondary antibodies, and bands were visualized using a ChemiDoc XRS+ imaging system (Bio-Rad, Hercules, CA, USA).
2.6. Statistical analysis
The results are presented as the mean ± standard deviation (SD). Data were analyzed using one-way analysis of variance with Duncan's multiple range test using SPSS version 20 (SPSS Inc., Armonk, NY, USA). A p-value of <0.05 was considered statistically significant.
3. Results
3.1. Chemical profiling of PLE
UPLC-qTOF-MS chromatograms of PLE are shown in Fig. 1. The known compounds in the pepper leaf were identified by comparing with reference compounds according to the mass spectrum and retention time. The unknown compounds were identified by analyzing the MS/MS fragmentation and by referring to previous reports. As a result, 24 compounds were unambiguously identified by comparison with the references. The major peaks are summarized in ESI Table 1,† and the most commonly identified compound was flavonoid-O-glycoside.
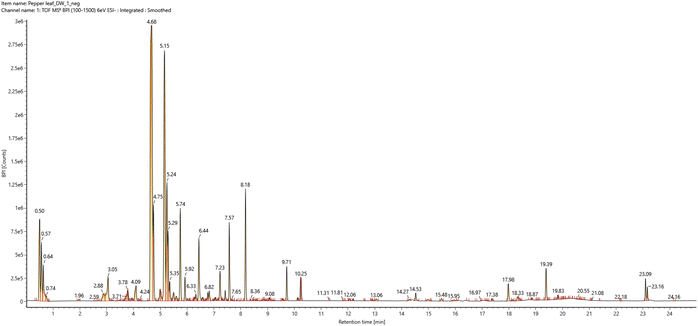 |
| Fig. 1 The total ion chromatogram of the water-soluble extract from pepper (Capsicum annuum L.) leaves in negative ion mode ESI-qTOF-MS detection. | |
3.2.
In vitro adipogenesis and the prebiotic effects of PLE
3.2.1. Effects of PLE on adipogenesis in 3T3-L1 cells.
The difference of cell viability was not significant at concentrations up to 250 μg mL−1 (Fig. 2A). The results indicated that PLE concentrations of 50 and 100 μg mL−1 should be used for further experiments in 3T3-L1 cells. As shown in Fig. 2B, no significant differences were found in the adiponectin contents in the PLE-treated cells compared with those in the control group.
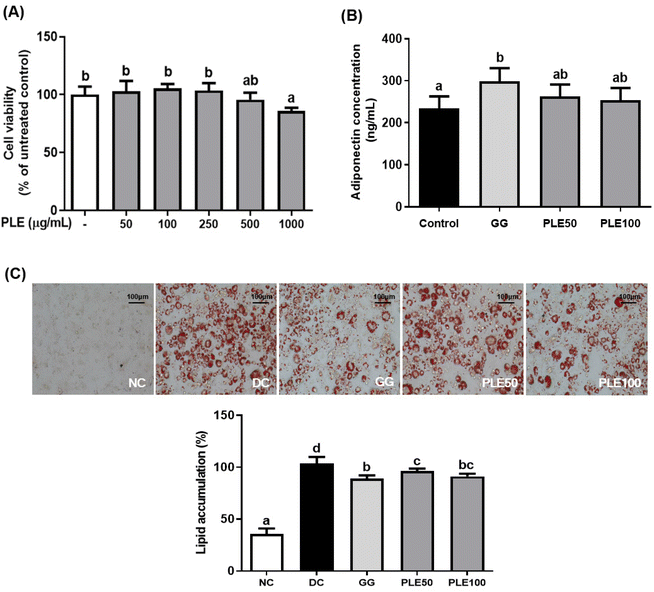 |
| Fig. 2 Effect of the water-soluble extract from pepper leaves (PLE) on the 3T3-L1 cells. (A) Cell viability; (B) adiponectin concentration; and (C) lipid accumulation. Data are presented as the mean ± standard deviation (n = 3). The different letters (a–d) indicate a significant difference (p < 0.05) determined by Duncan's multiple range test. Garcinia gummi-gutta extract was used as a positive control. NC, undifferentiated normal control; DC, differentiated control with MDI; GG, treated G. gummi-gutta (50 μg mL−1) with MDI; PLE50, treated PLE (50 μg mL−1) with MDI; PLE100, treated PLE (100 μg mL−1) with MDI. | |
Fig. 2C shows the ORO staining of lipid accumulation in the 3T3-L1 cells. The cells were induced to differentiate and the lipid accumulation levels in the PLE100 group were compared with those in the control group (lipid accumulation set to 100%). Lipid accumulation was significantly (p < 0.05) lower in the PLE100 and GG groups compared with that in the control group. These results showed that PLE suppressed lipid accumulation during differentiation.
3.2.2. Prebiotic effect of PLE on probiotic strains.
PLE showed a significantly higher prebiotic activity score compared with that of the control group (p < 0.05) (Fig. 3). The prebiotic activity scores for L. paracasei were 1.28-fold higher in the PLE0.5% group compared with those in the inulin group (not significant). The prebiotic activity scores for L. lactis were 1.40-fold higher in the inulin group compared with those in the PLE0.5% group. In contrast, those for B. bifidum and B. longum were 2.40- and 2.56-fold significantly higher in the PLE0.5% group compared with those in the inulin group (p < 0.05), respectively. Furthermore, the pH of the final medium (24 h of incubation) was assessed as shown in ESI Table 2.† The pH values of the medium containing the PLE0.5% group strains were similar to or lower than that of the medium containing the inulin group strains (p < 0.05). However, after 24 h of incubation, the medium containing PLE showed significantly lower pH values than those of the control group, except for L. lactis (p < 0.05).
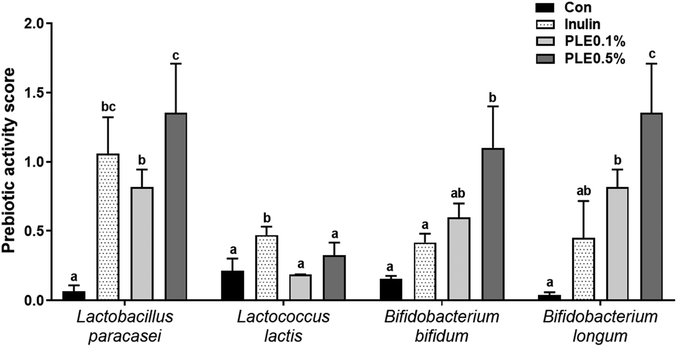 |
| Fig. 3 Effect of the water-soluble extract from pepper leaves (PLE) on the prebiotic activity in probiotic strains after 24 h of incubation. Data are presented as the mean ± standard deviation (n = 3). The different letters (a–c) indicate a significant difference (p < 0.05) between the probiotic strains of the groups determined by Duncan's multiple range test. Inulin was used as a positive control. | |
3.3.
In vivo anti-obesity effects of PLE
3.3.1. Effects of PLE on body weight, food intake, and eWAT weight.
The body weight and food intake changes at the end point of the experiments are shown in Fig. 4A and B. The body weight in the HF group was significantly increased compared with that in the NC group (p < 0.05). The GG, PLE50, and PLE100 groups did not differ significantly in body weight. However, they showed 8.8%, 10.5%, and 11.8% lower weights, respectively, compared with that in the HF group. There were no significant differences in food intake among the experimental groups. Importantly, the eWAT weight per body weight for the PLE100 group was significantly lower compared with that of the HF group (p < 0.05; Fig. 4C).
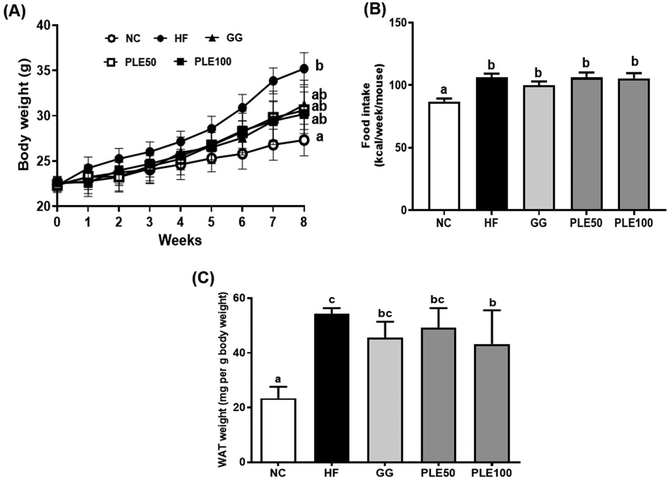 |
| Fig. 4 Effect of the water-soluble extract from pepper leaves (PLE) on the HFD-fed mice. (A) Body weight changes during the 8 weeks of feeding; (B) food intake during the 8 weeks of feeding; and (C) white adipose tissue weight (mg per g body weight). Data are presented as the mean ± standard deviation (n = 9). The different letters (a–c) indicate a significant difference (p < 0.05) between the experimental groups determined by Duncan's multiple range test. Garcinia gummi-gutta extract was used as a positive control. NC, normal control; HF, high-fat diet; GG, 50 mg kg−1G. gummi-gutta + HFD; PLE50, 50 mg kg−1 PLE + HFD; PLE100, 100 mg kg−1 PLE + HFD. | |
3.3.2. Effects of PLE on the serum biomarkers in the serum and liver.
The concentrations of serum TG and TC are shown in Fig. 5A and B, respectively. The serum TG and TC levels in the PLE100 group were 26.1 and 12.2% lower, respectively, compared with those in the HF group (p < 0.05), suggesting that PLE exerts a hypolipidemic effect in HFD-fed mice. Furthermore, the levels of not only insulin but also those of leptin, an adipocyte-derived hormone, of the HF group were found to be significantly higher than those of the NC (Fig. 5C and D) and PLE groups, suggesting that the HFD-induced increased levels of insulin and leptin had been decreased by PLE supplementation.
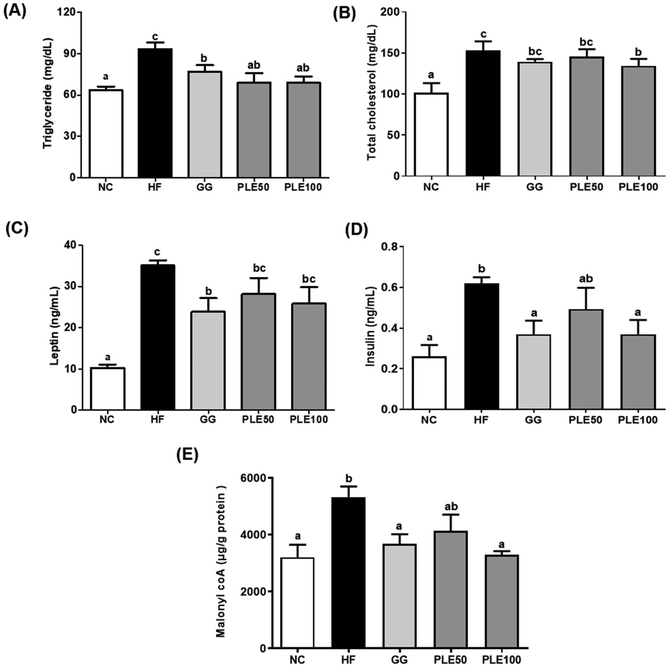 |
| Fig. 5 Effect of the water-soluble extract from pepper leaves (PLE) on the serum lipid profiles in the HFD-fed mice. (A) Triglyceride; (B) total cholesterol; (C) leptin; and (D) insulin in the serum, and (E) hepatic malonyl-coenzyme A in the hepatic tissues. Data are presented as the mean ± standard deviation (n = 9). The different letters (a–c) indicate a significant difference (p < 0.05) between the experimental groups determined by Duncan's multiple range test. Garcinia gummi-gutta extract was used as a positive control. NC, normal control; HF, high-fat diet; GG, 50 mg kg−1G. gummi-gutta + HFD; PLE50, 50 mg kg−1 PLE + HFD; PLE100, 100 mg kg−1 PLE + HFD. | |
In addition, the hepatic malonyl-CoA levels were measured in the hepatic tissues (Fig. 5E). The PLE50 and PLE100 groups decreased the hepatic malonyl-CoA levels by 22.4 and 38.3%, respectively, as compared with those of the HF groups (p < 0.05).
3.3.3. Effects of PLE on the histological changes.
Liver lipid deposition and hepatic steatosis were monitored in each group using ORO staining (Fig. 6A). The NC group had no lipid deposition in the liver, but the HF group had higher proportions of lipid droplets in the liver compared with those of the PLE groups (p < 0.05). The GG, PLE50, and PLE100 groups showed 12.2, 16.2, and 21.3% less staining than that of the HF group, respectively. The adipocyte sizes in the PLE50 and PLE100 groups were decreased by 35.6 and 61.0%, respectively (Fig. 6B). The PLE100 group exhibited significantly decreased adipocyte sizes (p < 0.05) than those of the HF group.
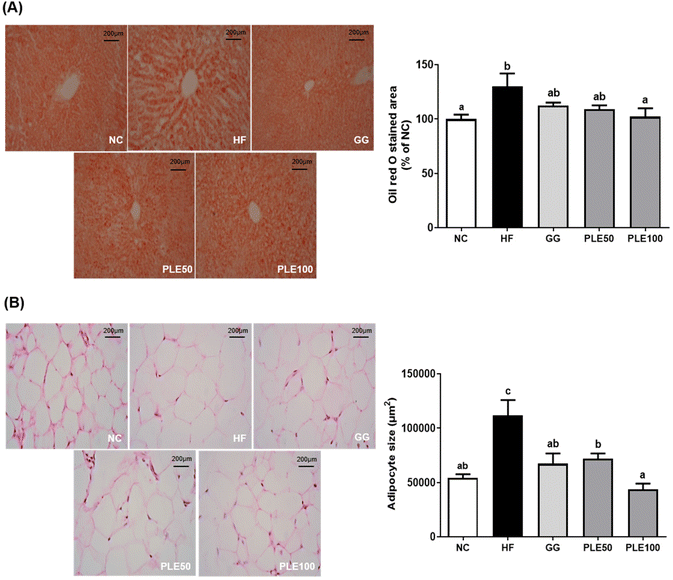 |
| Fig. 6 Effect of the water-soluble extract from pepper leaves (PLE) on the histology of the liver and epididymal white adipose tissues (eWAT) in the HFD-fed mice. (A) Oil red O staining of the liver tissues and quantification of the stained area, and (B) hematoxylin and eosin staining of the eWAT and quantification of the adipocyte size. Data are presented as the mean ± standard deviation (n = 9). The different letters (a–c) indicate a significant difference (p < 0.05) between the experimental groups determined by Duncan's multiple range test. Garcinia gummi-gutta extract was used as a positive control. NC, normal control; HF, high-fat diet; GG, 50 mg kg−1G. gummi-gutta + HFD; PLE50, 50 mg kg−1 PLE + HFD; PLE100, 100 mg kg−1 PLE + HFD. | |
3.3.4. Effects of PLE on the inflammatory biomarkers and gut permeability.
The concentrations of serum LPS and LTB4 are shown in Fig. 7A and B. The serum LPS and LTB4 levels in the PLE100 group were 39.2 and 26.1% lower, respectively, compared with those of the HF group (p < 0.05). In addition, the colon was significantly longer in the PLE100 group than that in the HF group, whereas the colon pH was significantly lower in the PLE groups than that in the HF group (p < 0.05; Fig. 7C and D). We examined the effects of PLE on gut permeability in mice using the FITC-dextran levels in the mouse blood samples, as shown in Fig. 7E and F. The HF group showed 4.4 times larger area under the plasma FITC-dextran curve compared to the PLE100 group. The concentration of FITC-dextran in the GG, PLE50, and PLE100 groups was 32.5%, 67.7%, and 62.0% lower than that in the HF group (p < 0.05), respectively.
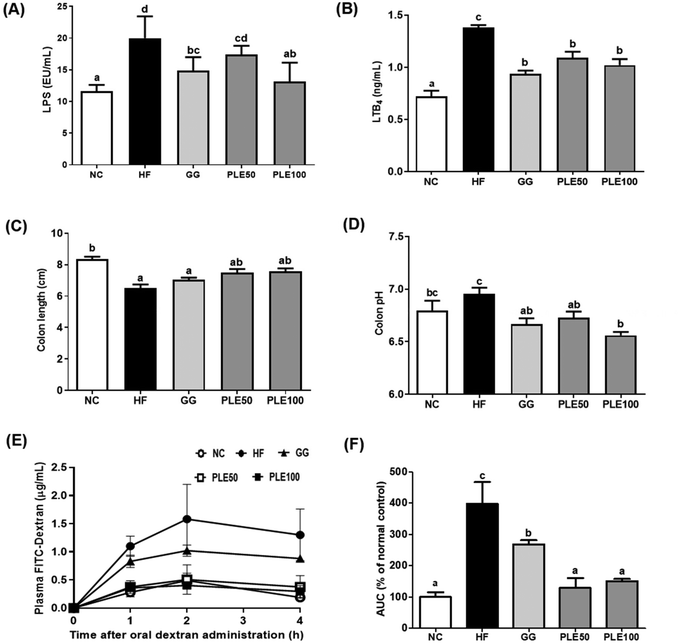 |
| Fig. 7 Effect of the water-soluble extract from pepper leaves (PLE) on gut health and inflammation in the HFD-fed mice. (A) Serum LPS; (B) serum LTB4; (C) colon length (cm); (D) colon pH; (E) plasma FITC dextran concentration; and (F) area under the curve of plasma FITC dextran levels. Data are presented as the mean ± standard deviation (n = 9). The different letters (a–c) indicate a significant difference (p < 0.05) between the experimental groups determined by Duncan's multiple range test. Garcinia gummi-gutta extract was used as a positive control. NC, normal control; HF, high-fat diet; GG, 50 mg kg−1G. gummi-gutta + HFD; PLE50, 50 mg kg−1 PLE + HFD; PLE100, 100 mg kg−1 PLE + HFD. | |
3.3.5. Effects of PLE on the MPO activity and fecal enzyme activity in the intestinal microflora.
MPO activity was higher in the HF group than that in the other groups (Fig. 8A). The GG, PLE50, and PLE100 groups showed 55.9%, 64.6%, and 78.3% lower activity levels compared to that of the HF group, respectively (p < 0.05). The enzymatic activity of intestinal microorganisms (β-glucosidase, β-glucuronidase, and tryptophanase) in the feces is shown in Fig. 8B–D, respectively. The activities of these enzymes were significantly lower in the PLE50 and PLE100 groups compared with those in the HF group (p < 0.05).
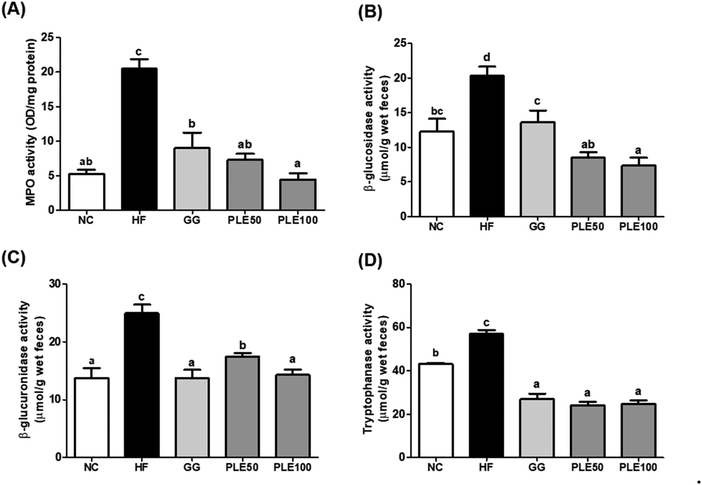 |
| Fig. 8 Effect of the water-soluble extract from pepper leaves (PLE) on the myeloperoxidase (MPO) and fecal enzyme activities of the intestinal microflora in the HFD-fed mice. (A) MPO activity in the colon tissues, and (B) β-glucosidase, (C) β-glucuronidase and (D) tryptophanase activities in the feces. Data are presented as the mean ± standard deviation (n = 9). The different letters (a–c) indicate a significant difference (p < 0.05) between the experimental groups determined by Duncan's multiple range test. Garcinia gummi-gutta extract was used as a positive control. NC, normal control; HF, high-fat diet; GG, 50 mg kg−1G. gummi-gutta + HFD; PLE50, 50 mg kg−1 PLE + HFD; PLE100, 100 mg kg−1 PLE + HFD. | |
3.3.6. Effects of PLE on lipid metabolism in the liver and eWAT.
To evaluate whether PLE treatment could change the gene expression associated with eWAT lipid metabolism, PGC-1α, CPT1, adiponectin, and PPAR-α protein expressions were measured (Fig. 9A). The PGC-1α, CPT1, adiponectin, and PPAR-α protein expression levels were 1.7-, 3.7-, 2.3-, and 2.2-fold decreased, respectively, in the HF group compared with those in the NC group. The PLE50 and PLE100 groups showed increased protein expression levels.
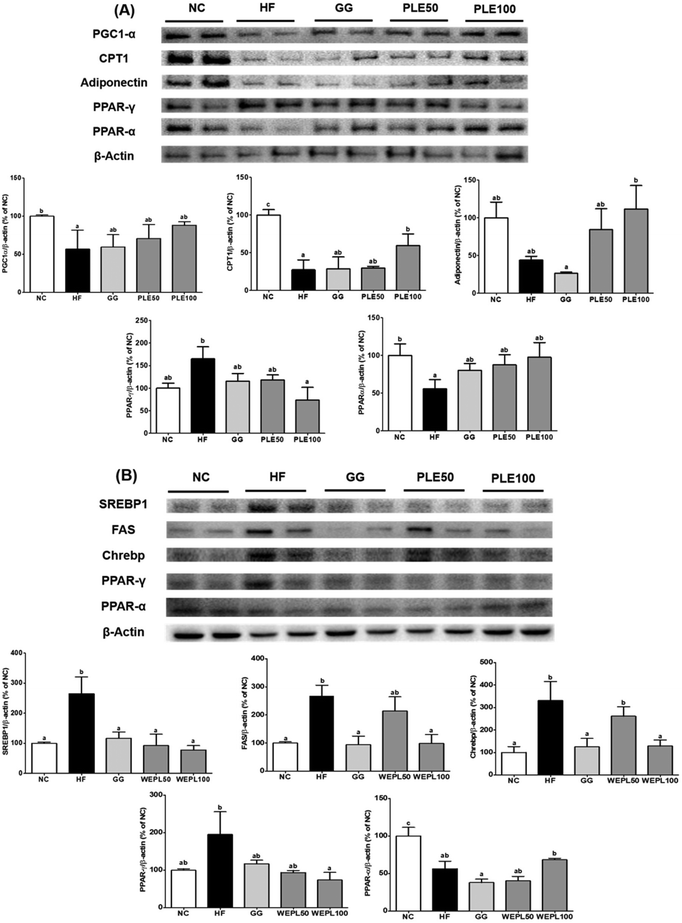 |
| Fig. 9 Effect of the water-soluble extract from pepper leaves (PLE) on the changes of lipid metabolism in the epididymal white adipose tissue (eWAT) and liver in the HFD-fed mice. (A) The protein expressions of peroxisome proliferator-activated receptor gamma coactivator 1-alpha (PGC-1α), carnitine palmitoyltransferase 1 (CPT1), adiponectin, peroxisome proliferator-activated receptor gamma (PPAR-γ) and peroxisome proliferator-activated receptor alpha (PPAR-α) in the eWAT, and (B) the protein expressions of sterol regulatory element binding protein 1 (SREBP1), fatty acid synthase (FAS), carbohydrate-responsive element binding protein (ChREBP), peroxisome proliferator-activated receptor gamma (PPAR-γ) and peroxisome proliferator-activated receptor alpha (PPAR-α) in the liver. Data are presented as the mean ± standard deviation (n = 9). The different letters (a–c) indicate a significant difference (p < 0.05) between the experimental groups determined by Duncan's multiple range test. Garcinia gummi-gutta extract was used as a positive control. NC, normal control; HF, high-fat diet; GG, 50 mg kg−1G. gummi-gutta + HFD; PLE50, 50 mg kg−1 PLE + HFD; PLE100, 100 mg kg−1 PLE + HFD. | |
In addition, PPARγ protein expression was reduced by 55.2% in the PLE100 group compared with that in the HF group. In the liver tissues, SREBP1, FAS, ChREBP, and PPAR-γ protein expressions were significantly decreased in the PLE100 group (p < 0.05) by 70.7, 63.2, 61.2, and 61.9%, respectively compared to those in the HF group (Fig. 9B). Furthermore, the PPAR-α protein expression was reduced by 43.6% in the HF group compared to that in the NC group.
4. Discussion
Obesity is a major causative factor of many metabolic diseases associated with numerous health problems, including cancer, diabetes mellitus, vascular disease, and hypertension.11 An HFD induces inflammation, obesity, and other metabolic disorders. Therefore, many studies have been performed on obesity and obesity-induced diseases.12 Consumption of fruits and vegetables has been associated with the prevention of chronic diseases. A constant supply of phytochemical-containing plants with health benefits can reduce the risk of chronic diseases in humans.13C. annuum L., which has various beneficial physiological activities and is characterized by its chemopreventive, anti-inflammatory, antioxidant, hypolipidemic, and weight-reducing effects, has been adopted as a pungent food additive worldwide.14 The present study showed that the water extract of red pepper leaves had anti-obesity effects in both in vitro and in vivo experiments. In particular, its effect on adipogenic differentiation in 3T3-L1 pre-adipocytes cells and body weight and lipid profiles and gene expressions involved in energy metabolism was assessed using HFD-fed C57BL/6J mice.15G. gummi-gutta extract, a commercial anti-obesity ingredient, was used as a positive control to compare the efficacy of PLE.16
Adipogenesis, the cellular differentiation of preadipocytes to adipocytes, is a complex process regulated by many factors. The 3T3-L1 preadipocyte cell line is the most popular cell model for adipogenesis and a reliable in vitro model for studying the molecular mechanisms of adipocyte differentiation.17 In this study, we observed that PLE decreases the induction of morphological changes and lipid accumulation in these cells. The cell viability assay during differentiation revealed that the effect of PLE was not due to the effects on 3T3-L1 cell death. The levels of lipid accumulation were significantly decreased following the treatment with 100 μg mL−1 of PLE. Adiponectin is a protein produced by adipocytes. It acts as a hormone and plays an important role in modulating glucose and lipid metabolism as an insulin-sensitizing adipocytokine.18 PLE treatment suppressed the release of adiponectin during differentiation. These results showed that PLE decreased lipid accumulation and the adiponectin concentration.
Prebiotics such as fructooligosaccarides, galactooligosaccharides, and inulin selectively enhance the effects of one or a group of beneficial bacteria, directly or indirectly improving the health of the host.19 We quantified the functional stability of inulin and PLE by comparing their prebiotic activity before (0 h) and after (24 h) exposure to four representative probiotic strains. Acidic pH conditions, as might occur in the fermentation of inulin and PLE, influenced the prebiotic activity of all prebiotic strains. Probiotic strains had the highest or similar prebiotic activity score in PLE compared to those in commercial prebiotics, such as inulin.
The findings of this study showed that the oral administration of PLE reduced the HFD-induced weight gain in mice without altering food intake. Furthermore, obesity is characterized by an increase in fat tissue mass and adipocyte size. In addition, PLE treatment decreased the serum levels of TG, TC, leptin, and insulin, which are associated with energy expenditure and weigh management, during exposure to a HFD. Leptin also interacts with insulin, impacting glucose and lipid homeostasis.20 PLE dose-dependently decreased lipid accumulation in the liver tissues with lipid droplets and significantly decreased the adipocyte size in the eWAT. Furthermore, recent studies have shown that HFD intake induces lipid droplet formation and leads to increased TG levels, which are associated with lipid accumulation in the liver.21 These results suggest that PLE can regulate body weight gain, serum hormone levels, and lipid droplet production. Malonyl-CoA acutely regulates fatty acid oxidation in the liver in vivo. In the liver, glucose and insulin inhibit fatty acid oxidation by altering the malonyl-CoA levels.22 The concentration of hepatic malonyl-CoA was determined in HFD-induced mice treated with PLE. The results showed that the concentration of malonyl-CoA was higher in the HF group compared with those in the GG and PLE groups.
Many studies have reported that HFD-induced metabolic endotoxemia and inflammation are closely related to LPS. LPS, a major component of Gram-negative bacteria, is well known to induce pro-inflammatory responses in macrophages, and an HFD results in a higher serum LPS concentration, known as endotoxemia.23 LTB4 has also been studied as a pro-inflammatory lipid mediator generated from arachidonic acid and it was found that HFD-fed mice exhibit increased LTB4 levels in the liver, muscles, and adipose tissue compared to normal diet-fed mice.24 Our results suggested that PLE-administered mice showed suppressed serum LPS and LTB4 levels compared to those in the HF group. Moreover, obesity-related gut microbiota dysbiosis is directly linked to HFD and characterized by increased gut barrier permeability, known as leaky gut, enhancing the concentration of LPS in the blood stream, which causes inflammation and related metabolic diseases in rodents and humans.25 In agreement with these findings, increased gut permeability and enhanced serum LPS production are associated with an HFD, which were recovered by PLE treatment in vivo. Furthermore, MPO, a highly abundant protein in neutrophils, is considered a marker of inflammation, oxidative stress, lipoprotein oxidation, and atherosclerosis development; MPO may have a regulatory role in diet-induced obesity.26 The activity of MPO in the colon was more potently increased in the HF group compared with that in the PLE groups. Hence, PLE has the potential to prevent intestinal inflammation in rodent models. In addition, fecal enzyme activities such as those of β-glucosidase, β-glucuronidase, and tryptophanase were increased in mice after the consumption of an HFD. These bacterial enzymes exert potentially harmful effects on the host. Fecal enzymes are involved in the conversions of endogenous toxins and the increased growth of harmful E. coli and Enterobacteriaceae species.27 These three bacterial enzyme levels were significantly decreased in the PLE groups compared to those in the HF group. These results showed that PLE ameliorates the gut dysbiosis induced by chronic high fat consumption.
Eight-week administration of PLE significantly downregulated the expressions of PGC1-α, CPT1, and adiponectin in the eWAT of the HFD-fed mice. PGC-1α is a major transcriptional cofactor for mitochondrial biogenesis.28 These proteins can interact in adipose tissues. Increased PPAR-γ expression is observable after HFD treatment. It is well established that PPAR-γ activation decreases adiponectin secretion.29 As expected, PPAR-γ expression was significantly decreased in the PLE group, accompanied by the corresponding increase in adiponectin expression. SREBP1 and ChREBP are central regulators of most hepatic lipid synthesis-related genes, including ACC, FAS, and stearoyl-CoA desaturase 1.30 In the PLE groups, which were on HFD feeding, the expressions of lipogenic genes, such as SREBP1 and ChREBP, were significantly downregulated. PPAR-α is predominantly expressed in the liver, and PPAR-α activation has been shown to be beneficial in hepatic lipid accumulation and protect mice from HFD-induced hepatic steatosis.31 We found increased gene expression of PPAR-α in the PLE100 group compared with that in the HF group. These results suggest that PPAR-γ and PPAR-α regulate the expressions of downstream target genes, such as SREBP1, ChREBP, and FAS.
5. Conclusions
The results of the present work demonstrated that PLE exhibited prebiotic activity with anti-obesity effects. In vitro, PLE increases the growth of beneficial microbes and inhibits intracellular lipid accumulation in 3T3-L1 preadipocytes. In vivo, PLE inhibited HFD-induced body weight gain, decreased the serum endotoxin level and inflammation, and regulated lipid metabolism. Taken together, our findings suggest that PLE possesses the potential to be beneficial for the prevention of obesity and related metabolic diseases.
Author contributions
Ho-Young Park designed the research; Mi-Jin Oh, Hye-Bin Lee, and Guijae Yoo conducted the experiments; Miri Park and Inwook Choi analyzed the data and performed the statistical analysis; Chang Hyun Lee collected the experimental pathology data; Mi-Jin Oh and Ho-Young Park wrote the manuscript. All authors discussed the results and approved the final manuscript.
Conflicts of interest
The authors declare no conflict of interest.
Acknowledgements
This research was supported by the Main Research Program (E0210602-02) of the Korea Food Research Institute (KFRI) funded by the Ministry of Science and ICT.
References
- J.-H. Wang, S. Bose, H.-G. Kim, K.-S. Han and H. Kim, Fermented Rhizoma Atractylodis Macrocephalae alleviates high fat diet-induced obesity in association with regulation of intestinal permeability and microbiota in rats, Sci. Rep., 2015, 5, 1–10 Search PubMed.
- J. Rhyu, M. S. Kim, M.-K. You, M.-A. Bang and H.-A. Kim, Pear pomace water extract inhibits adipogenesis and induces apoptosis in 3T3-L1 adipocytes, Nutr. Res. Pract., 2014, 8, 33–39 CrossRef PubMed.
- B. O. Cho, J. Choi, H. J. Kang, D. N. Che, J. Y. Shin, J. S. Kim, S. J. Kim and S. I. Jang, Anti-obesity effects of a mixed extract containing Platycodon grandiflorum, Apium graveolens and green tea in high-fat-diet-induced obese mice, Exp. Ther. Med., 2020, 19, 2783–2791 Search PubMed.
- M. L. Bonet, J. A. Canas, J. Ribot and A. Palou, Carotenoids and their conversion products in the control of adipocyte function, adiposity and obesity, Arch. Biochem. Biophys., 2015, 572, 112–125 CrossRef PubMed.
- K.-H. Sim and Y.-S. Han, The antimutagenic and antioxidant effects of red pepper seed and red pepper pericarp (Capsicum annuum L.), Prev. Nutr. Food Sci., 2007, 12, 273–278 CrossRef.
-
M. Imran, M. S. Butt and H. A. R. Suleria, Capsicum annuum bioactive compounds: health promotion perspectives, in Bioactive Molecules in Food, ed. J. M. Mérillon and K. Ramawat, 2017, pp. 1–22 Search PubMed.
- W.-Y. Song, K.-H. Ku and J.-H. Choi, Effect of ethanol extracts from red pepper seeds on antioxidative defense system and oxidative stress in rats fed high-fat· high-cholesterol diet, Nutr. Res. Pract., 2010, 4, 11–15 CrossRef PubMed.
- S. Zhang, H. Hu, L. Wang, F. Liu and S. Pan, Preparation and prebiotic potential of pectin oligosaccharides obtained from citrus peel pectin, Food Chem., 2018, 244, 232–237 CrossRef PubMed.
- A. Affan, N. Al-Jezani, P. Railton, J. N. Powell and R. J. Krawetz, Multiple mesenchymal progenitor cell subtypes with distinct functional potential are present within the intimal layer of the hip synovium, BMC Musculoskeletal Disord., 2019, 20, 1–12 CrossRef PubMed.
- Y. Xiu, K. Wang, J. Chen, Z. Zhuo and Y. Xiu, Liposomal N-acylethanolamine-hydrolyzing acid amidase (NAAA) inhibitor F96 as a new therapy for colitis, RSC Adv., 2020, 10, 34197–34202 RSC.
- H.-W. Lee and S. Pyo, Acrylamide induces adipocyte differentiation and obesity in mice, Chem.-Biol. Interact., 2019, 298, 24–34 CrossRef CAS PubMed.
- M. Gao, Y. Ma and D. Liu, High-fat diet-induced adiposity, adipose inflammation, hepatic steatosis and hyperinsulinemia in outbred CD-1 mice, PLoS One, 2015, 10, e0119784 CrossRef PubMed.
- S. M. Frank, J. Webster, B. McKenzie, P. Geldsetzer, J. Manne-Goehler, G. Andall-Brereton, C. Houehanou, D. Houinato, M. S. Gurung and B. W. Bicaba, Consumption of fruits and vegetables among individuals 15 years and older in 28 low-and middle-income countries, J. Nutr., 2019, 149, 1252–1259 CrossRef PubMed.
- K. Srinivasan, Biological activities of red pepper (Capsicum annuum) and its pungent principle capsaicin: a review, Crit. Rev. Food Sci. Nutr., 2016, 56, 1488–1500 CrossRef CAS PubMed.
- M.-C. Kang, N. Kang, S.-C. Ko, Y.-B. Kim and Y.-J. Jeon, Anti-obesity effects of seaweeds of Jeju Island on the differentiation of 3T3-L1 preadipocytes and obese mice fed a high-fat diet, Food Chem. Toxicol., 2016, 90, 36–44 CrossRef.
- C. Guillén-Enríquez, V. Lopez-Teros, U. Martín-Orozco, J. A. López-Díaz, D. Hierro-Ochoa, A. Ramos-Jiménez, H. Astiazarán-García, N. D. R. Martínez-Ruiz and A. Wall-Medrano, Selected physiological effects of a Garcinia gummi-gutta extract in rats fed with different hypercaloric diets, Nutrients, 2018, 10, 565 CrossRef PubMed.
- K. M. de Melo, F. T. B. de Oliveira, R. A. C. Silva, A. L. G. Quinderé, J. D. B. Marinho-Filho, A. J. Araújo, E. D. B. Pereira, A. A. Carvalho, M. H. Chaves and V. S. Rao, α, β-Amyrin, a pentacyclic triterpenoid from Protium heptaphyllum suppresses adipocyte differentiation accompanied by down regulation of PPARγ and C/EBPα in 3T3-L1 cells, Biomed. Pharmacother., 2019, 109, 1860–1866 CrossRef PubMed.
- S. Behl, A. Adem, A. Hussain and J. Singh, Effects of rilpivirine, 17β-estradiol and β-naphthoflavone on the inflammatory status of release of adipocytokines in 3T3-L1 adipocytes in vitro, Mol. Biol. Rep., 2019, 46, 2643–2655 CrossRef PubMed.
- I. Figueroa-Gonzalez, G. Rodriguez-Serrano, L. Gomez-Ruiz, M. Garcia-Garibay and A. Cruz-Guerrero, Prebiotic effect of commercial saccharides on probiotic bacteria isolated from commercial products, Food Sci. Technol., 2019, 39, 747–753 CrossRef.
- K. Mendoza-Herrera, A. A. Florio, M. Moore, A. Marrero, M. Tamez, S. N. Bhupathiraju and J. Mattei, The leptin system and diet: a mini review of the current evidence, Front. Endocrinol., 2021, 12, 749050 CrossRef.
- I. Korovila, A. Höhn, T. Jung, T. Grune and C. Ott, Reduced liver autophagy in high-fat diet induced liver steatosis in new zealand obese Mice, Antioxidants, 2021, 10, 501 CrossRef CAS PubMed.
- S. Rodriguez and M. J. Wolfgang, Targeted chemical-genetic regulation of protein stability in vivo, Chem. Biol., 2012, 19, 391–398 CrossRef CAS PubMed.
- S.-Y. Kwon, M. Ro and J.-H. Kim, Mediatory roles of leukotriene B4 receptors in LPS-induced endotoxic shock, Sci. Rep., 2019, 9, 1–8 CrossRef.
- P. Li, D. Y. Oh, G. Bandyopadhyay, W. S. Lagakos, S. Talukdar, O. Osborn, A. Johnson, H. Chung, M. Maris, J. M. Ofrecio, S. Taguchi, M. Lu and J. M. Olefsky, LTB4 causes macrophage–mediated inflammation and directly induces insulin resistance in obesity, Nat. Med., 2015, 21, 239–247 CrossRef CAS PubMed.
- I. J. Malesza, M. Malesza, J. Walkowiak, N. Mussin, D. Walkowiak, R. Aringazina, J. Bartkowiak-Wieczorek and E. Mądry, High-fat, western-style diet, systemic inflammation, and gut microbiota: a narrative review, Cells, 2021, 10, 3164 CrossRef PubMed.
- M. G. Qaddoumi, M. Alanbaei, M. M. Hammad, I. Al Khairi, P. Cherian, A. Channanath, T. A. Thanaraj, F. Al-Mulla, M. Abu-Farha and J. Abubaker, Investigating the role of myeloperoxidase and angiopoietin-like protein 6 in obesity and diabetes, Sci. Rep., 2020, 10, 1–10 CrossRef PubMed.
- W. Dworzański, E. Cholewińska, B. Fotschki, J. Juśkiewicz and K. Ognik, Oxidative, epigenetic changes and fermentation processes in the intestine of rats fed high-fat diets supplemented with various chromium forms, Sci. Rep., 2022, 12, 9817 CrossRef PubMed.
- M. Kobayashi, Y. Deguchi, Y. Nozaki and Y. Higami, Contribution of pgc-1α to obesity-and caloric restriction-related physiological changes in white adipose tissue, Int. J. Mol. Sci., 2021, 22, 6025 CrossRef PubMed.
- J. F. Landrier, E. Kasiri, E. Karkeni, J. Mihály, G. Béke, K. Weiss, R. Lucas, G. Aydemir, J. Salles and S. Walrand, Reduced adiponectin expression after high–fat diet is associated with selective up–regulation of ALDH1A1 and further retinoic acid receptor signaling in adipose tissue, FASEB J., 2017, 31, 203–211 CrossRef PubMed.
- A. G. Linden, S. Li, H. Y. Choi, F. Fang, M. Fukasawa, K. Uyeda, R. E. Hammer, J. D. Horton, L. J. Engelking and G. Liang, Interplay between ChREBP and SREBP-1c coordinates postprandial glycolysis and lipogenesis in livers of mice, J. Lipid Res., 2018, 59, 475–487 CrossRef PubMed.
- A. Ammazzalorso, I. Bruno, R. Florio, L. De Lellis, A. Laghezza, C. Cerchia, B. De Filippis, M. Fantacuzzi, L. Giampietro and C. Maccallini, Sulfonimide and amide derivatives as novel PPARα antagonists: Synthesis, antiproliferative activity, and docking studies, ACS Med. Chem. Lett., 2020, 11, 624–632 CrossRef.
|
This journal is © The Royal Society of Chemistry 2023 |
Click here to see how this site uses Cookies. View our privacy policy here.