DOI:
10.1039/D2FO03138H
(Paper)
Food Funct., 2023,
14, 471-488
Effects of dietary restriction on genome stability are sex and feeding regimen dependent†
Received
18th October 2022
, Accepted 17th November 2022
First published on 1st December 2022
Abstract
Preserving genome stability is essential to prevent aging and cancer. Dietary restriction (DR) is the most reproducible non-pharmacological way to improve health and extend lifespan in various species. Whether DR helps to preserve genome stability and whether this effect is altered by experimental variables remain unclear. Moreover, DR research relies heavily on experimental animals, making the development of reliable in vitro mimetics of great interest. Therefore, we tested the effects of sex and feeding regimen (time-restricted eating, alternate day fasting and calorie restriction) on genome stability in CF-1 mice and whether these effects can be recapitulated by cell culture paradigms. Here, we show that calorie restriction significantly decreases the spontaneous micronuclei (MN), a biomarker of genome instability, in bone marrow cells of females instead of males. Alternate day fasting significantly decreases cisplatin-induced MN in females instead of males. Unexpectedly, daily time-restricted eating significantly exacerbates cisplatin-induced MN in males but not in females. Additionally, we design several culture paradigms that are able to faithfully recapitulate the key effects of these DR regimens on genome stability. In particular, 30% reduction of serum, a mimetic of calorie restriction, exhibits a strong ability to decrease spontaneous and cisplatin-induced MN in immortalized human umbilical vein endothelial cells. We conclude that the effects of different DR regimens on genome stability are not universal and females from each diet regimen sustain a more stable genome than males. Our results provide novel insight into the understanding of how DR influences genome stability in a sex and regimen dependent way, and suggest that our in vitro DR mimetics could be adopted to study the underlying molecular mechanisms.
1 Introduction
All organisms need food to obtain external nutrients for maintaining tissue and cellular homeostasis. The idea of health promotion by food or dietary modifications has received significant attention because a large amount of data derived from diverse epidemiological and experimental studies combined with clinical intervention trials underscores that food contents and quantity can have beneficial, or in contrast, harmful effects on human health.1–6 Dietary restriction (DR) is a broad term referring to several intervention regimens including the reduction/restriction of specific dietary components or in amounts or times of food provided.7 Generally, DR includes three types of regimens: calorie restriction (CR), intermittent fasting and macronutrient dilution. CR is a regimen describing a 20–40% reduction of calories from a normal ad libitum (AL) diet without malnutrition and is the first DR regimen to increase rodent lifespan in the 1930s.8 Despite its remarkable benefits, it is hard for humans to sustain long periods of CR, thereby motivating efforts to find alternative and sustainable regimens, including intermittent fasting, fasting-mimicking diet, ketogenic diet and macronutrient dilution.9 Among these, intermittent fasting usually encompasses a period of enforced fasting followed by a period of AL diet. Alternate day fasting (ADF), daily time-restricted eating/feeding (TRE) and weekly TRE are three commonly used methods to impose fasting.10
As the average lifespan of humans has increased, the study of aging and aging-related diseases becomes particularly important for public health. DR has grown in popularity in the past few years as multiple pieces of evidence have shown that different regimens of DR are potentially beneficial for retarding aging and increasing life- and health-spans in a wide variety of organisms including humans, with very few exceptions (reviewed in ref. 7). Studies in different organisms have identified an essential role of nutrient-responsive signaling molecules like mTOR, sirtuin family proteins and IGF-1 in mediating the beneficial effects of DR (reviewed in ref. 1). Despite extensive research, mechanisms by which DR extends lifespan and delays disease pathology are not fully dissected, as no single genetic or pharmaceutical manipulation mimics the multiple molecular and physiological benefits that occur with DR.
Aging is accompanied by numerous molecular alterations, including a progressive loss of genome stability.7 In eukaryotic cells, genome stability is fundamental for virtually all cellular processes. Although multiple mechanisms have evolved to ensure genome stability, the genome throughout the lifespan is continuously threatened by stresses of both exogenous and endogenous origins, such as environmental mutagens and cellular metabolites and biological processes.11 Depending on the degree, these insults induce DNA mutations, copy-number variants in some genomic regions, global genomic instability (GIN) and finally cell senescence and death. GIN is a well-established biomarker for both cancer12 and aging.13,14 In recent years, tremendous efforts have been made to reveal that clonal single-nucleotide variants and chromosomal alterations in human blood cells are associated with a shorter lifespan and a wide spectrum of aging-related diseases like cancers, cardiovascular diseases and neurodegenerative diseases, suggesting that loss of genome stability is a common pathway to the pathological risk of a wide range of human diseases.11
The DR field has largely focused on the beneficial effects of DR to attenuate the metabolic measurements that are relevant to age and longevity, such as plasma glucose, core body temperature, body weight, plasma insulin and leptin. Considering these, studies are needed to untangle whether protection against GIN is one under-determined means for DR to delay aging and disease pathology. In addition, as in most biomedical preclinical research studies, DR has been primarily characterized in male animals. It has been known that the physiological system involved in metabolic homeostasis exhibits a sex difference in most animals.15 In line with this, the existing data have pointed to a clear sex difference in physiological response to DR in rodents.16 However, studies on whether DR has sex-dependent potential to modulate the genome stability are still lacking. As considering sex a biological variable becomes mandatory in preclinical research,17 understanding the inherent differences of genome stability between males and females in response to DR is therefore of utmost importance and may present an opportunity to identify new factors that help to preserve a genome more stable in one sex than the other.
On the other hand, despite the aforementioned beneficial effects of DR, a long-term DR regimen has been shown to also have some safety concerns.10 One aspect of DR regimens is that inevitably one or more macro-/micronutrients are reduced or increased. We have previously shown that disrupting the balance of certain macro-/micronutrients or metabolites like folate,18 sugars,19,20 glutamine,21 homocysteine22,23 and bile acids24 has harmful or beneficial effects on genome stability in human in vitro cells and/or in mice in a dose-dependent manner. Hydrogen sulfide (H2S) produced from an endogenous trans-sulfuration pathway is essential for DR-mediated stress resistance.25 However, we have shown that a high concentration of H2S induces GIN in human cells.23 Besides, we have found that metformin and resveratrol, two CR mimetics, induce GIN in human non-cancerous cells in a dose-dependent manner.20,26 In addition, some metabolites may exert specific effects independent of total calorie intake. For instance, in order to provide energy for most tissues during periods of fasting, fatty acids in the liver are converted to ketone bodies that can reach milli-molar levels in human and rodent plasma within 24 hours of fasting.27 Recently, an in vitro study has shown that milli-molar levels of ketone bodies are able to cause chromosome mis-segregation and micronucleation.28 Overall, as the food composition, calorie intake and the duration and frequency of the fasting period are varied across the different forms of DR, we speculate that some DR regimens may have adverse effects on genome stability.
In order to successfully translate the DR regimens into medical treatment for humans, both their beneficial and adverse effects on genome stability need to be thoroughly investigated. To address this conceptually critical question, we designed the present study to primarily examine the effects of different DR regimens, including CR, ADF and TRE, on the genome stability of CF-1 mice. The secondary aim was to develop in vitro DR-mimic regimens that can recapitulate the genome-stability-modulating effects of DR in the immortalized human umbilical vein endothelial cells. Our results indicate that the effects of different DR regimens on genome stability are not universal and females from each diet regimen sustain a more stable genome than males. Importantly, our in vitro DR mimetics can be potentially adopted to study the underlying molecular mechanisms of DR regimens in modulating genome stability. This is the first study, to the best of our knowledge, that provides a parallel systematic evaluation of the effects of three well-known DR regimens on genome stability in both male and female mice.
2 Materials and methods
2.1 Mice and ethics approval
All mice used in this study were CF-1 between the ages of 12 and 20 weeks and the experimental groups were age-matched with one another. The mice were group housed (5 per cage) in ventilated cages with a 12 h light/12 h dark cycle (lights on from 08:00 am to 20:00 pm) and controlled temperature (22–25 °C), with AL access to food and water. The Committee on the Ethics of Biomedical Research at Yunnan Normal University (Kunming, China) approved all animal protocols used in this study and our mice experiments were performed according to the Guide for Care and Use of Laboratory Animals.
2.2 Experimental diets
Standard maintenance diet (SPF level) for mice: 3.4 kcal g−1 (14.23 kJ g−1) including 23.07% proteins, 11.85% fat and 65.08% carbohydrate. The diet was purchased from Keao-Xieli Feed Co., Ltd (Beijing, China) and the macronutrient composition can be obtained from the website of Keao-Xieli Feed (https://www.keaoxieli.com). Mice not consuming experimental diets were fed with a standard chow diet.
2.3 Feeding regimens
We chose three feeding regimens (TRE, ADF and CR) that have previously shown to extend the lifespan in mice (Fig. 1A). TRE mice were fed once daily and the provided food matched the caloric intake of the AL group (isocaloric). Since mice have a robust circadian rhythm with high activity during the night (nocturnal), TRE is ideal to set the meal time within the physically active phase of the day.29 According to the published protocols,30 TRE mice were allowed free access to food for 12 h (21:00 pm to 9:00 am) and food deprivation for 12 h (9:00 am to 21:00 pm). The 12 h daily eating period was selected based on the available data on rodents and humans which suggest that this period is ideal for balancing the beneficial effects and side effects.31 ADF was conducted to allow free access to food for 24 h and remove food for 24 h. Food was provided to the ADF cohorts at 9:00 am and withdrawn at 9:00 am on the next day. In the feeding days of the ADF regimen, each mouse was provided with a sufficient amount of food (15–20 g). As a control regimen, AL-fed mice were granted unlimited access to food anytime. Experiments were ended at 4 weeks from the start of the DR interventions. During the intervention period of each cohort, all mice were provided free access to water. Our use of 4 weeks of intervention was in line with several rodent studies using these DR regimens.
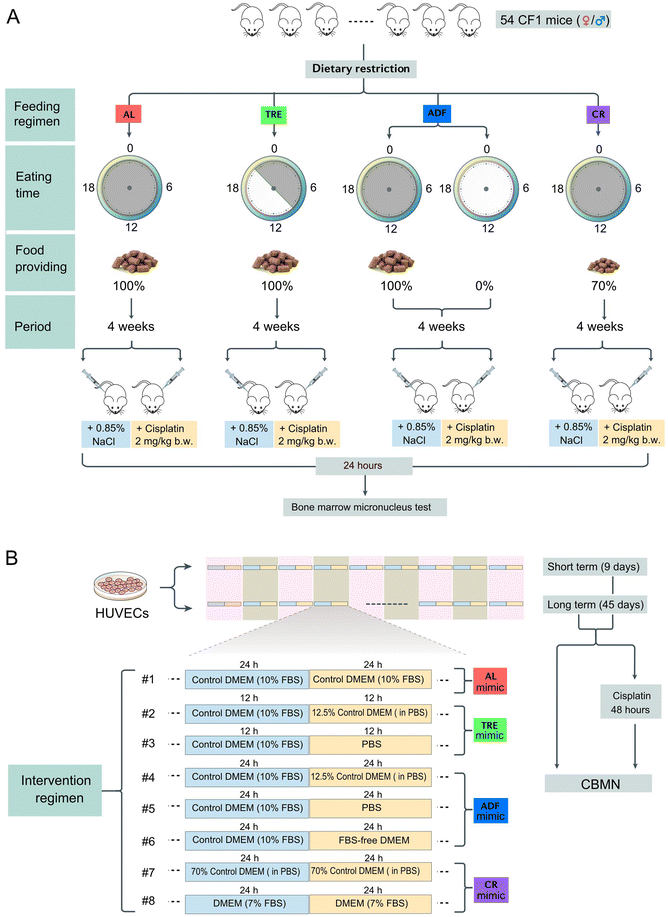 |
| Fig. 1 Schematic illustration of the in vivo and in vitro experimental designs. (A) Mice were subjected to a feed with an ad libitum (AL), time restricted eating (TRE), alternative day feeding (ADF) or calorie restriction (CR) diet for 4 weeks. AL-fed mice were granted unlimited access to food anytime. TRE mice were fed once daily (21:00–9:00) and the provided food matched the food intake of the AL group. ADF-fed mice were allowed free access to food for 24 h and removed food for 24 h. CR mice were fed 70% of the food consumed under the AL conditions. Each mouse represents an independent sample replicate. Following the 4-week period of intervention, mice of each cohort were either intraperitoneally injected with 2 mg per kg b.w. cisplatin or the same volume of saline. 24 h after the treatment, mice were killed and the bone marrows were isolated to perform the micronuclei test. (B) HUVECs were cultured in 8 different media that were designed to mimic the DR regimens in mice. Specifically, culture paradigm #1 was designed to mimic AL, culture paradigms #2 and #3 were designed to mimic TRE, culture paradigms #4, #5 and #6 were designed to mimic ADF, and culture paradigms #7 and #8 were designed to mimic CR. Cell intervention was either performed for 9 or 45 days. After each cycle of intervention, cells were treated with or without cisplatin for 48 h. Then, cells were harvested to perform the cytokinesis-block micronucleus (CBMN) assay. | |
Before the DR experiments, all mice were single housed and fed a standard maintenance diet AL for one week to get used to the food. During this period, the body weight and the daily consumed food of each mouse at the baseline (AL conditions) were recorded. These data provided a baseline to establish the daily amount of food that should be provided in TRE and CR cohorts. While AL, TRE and ADF mice received food in a hopper, CR mice were fed on the floor of the cage because CR made them too weak to get food from the hopper. Throughout the experiments, mice of all cohorts were single housed and the cages of each cohort were changed each day to exclude the accumulation of food particles from the preceding day. While single housing enables us to precisely measure the food intake of each mouse, one disadvantage is that it causes unnatural stress derived from isolation.32
2.4 Body weight and food intake
In each cohort, pre-weighed food was provided within the same time of day (21:00 pm for the TRE cohort and 21:00 pm for AL, ADF and CR cohorts) and the uneaten food and the body weight of each mouse were weighed at the same time (9:00 am). The daily food intake was calculated by subtracting the amount of uneaten food from the amount of supplied food. It should be pointed out that in the CR cohort, the daily food provided was not based on the food consumed in the AL cohort in the previous day, but was based on their own daily food intake under AL conditions continuously measured 7 days before the start of diet intervention. Because a long-term change from the AL diet to the 30% CR diet was not well tolerated by mice in the CR cohort at day 10, CR mice were fed the same amount of food as the AL mice for 4 days (day 11 to 15) before switching to the 30% CR diet. Thus, while our initial target was to achieve 30% CR, the eventual CR level attained was 21% in males and 24% in females.
2.5 The bone marrow micronucleus test
At the end of DR interventions (day 28), mice of each cohort were divided into two groups (3–4 mice per group). The mice of one group were intraperitoneally injected with 2 mg per kg b.w. cisplatin, whereas the mice of the other group received the same volume of saline. In the following 24 h, all DR-intervened mice were switched to the AL regimen. Then, all mice were killed by cervical dislocation. The bone marrow from both femurs was flushed with 3 mL saline as a fine suspension into a centrifuge tube containing 10% new-born calf serum (Sigma). This cell suspension was centrifuged at 2000 rpm for 5 min and the supernatant was removed. The pellet was resuspended in 0.5–1.5 ml of saline (with 10% new-born calf serum, depending on the amount of collected bone marrow cells) before being used for preparing slides by cytospin centrifugation. The air-dried slides were stained with 10% Giemsa in PBS (pH 6.8). For each mouse, 6 slides were prepared and each slide was randomly assigned to a trained research staff member to perform cell counts under an optical microscope with 1000× magnification (Olympus, Tokyo, Japan). At least 1000 polychromatic erythrocytes (PCEs) were screened per slide for the presence of MN and the number of micronucleated (MNed) PCEs per 1000 PCEs in each mouse was calculated.
2.6 Cell line and cell culture
Human umbilical vein endothelial cells (HUVECs), a cell line that was immortalized by hTERT, were kindly provided by Prof. Guixue Wang of Chongqing University (Chongqing, China). According to the ATCC, HUVECs (ATCC #CRL-1730) are a hypodiploid human cell line with a female karyotype (45, XX). HUVECs at a passage less than 15 were used in all our experiments. HUVECs were maintained as a monolayer in Dulbecco's Modified Eagle's Medium (DMEM; Gibco, NY, USA) supplemented with 10% fetal bovine serum (FBS, Gibco, NY, USA), 1% penicillin (5000 IU mL−1)/streptomycin (5 mg mL−1) solution (Gibco, NY, USA), and 1% L-glutamine (2 mM) (Gibco, NY, USA). All cell culture experiments were carried out at 37 °C under a 5% CO2 atmosphere in a humidified incubator. HUVECs were tested for mycoplasma contamination by PCR before being used.
2.7 Cell interventions
HUVECs were cultured in 6-well culture plates (Costar, Cambridge, MA, USA) at a density of 1 × 105 viable cells per well. In the control group, the culture medium was DMEM with 10% FBS and the culture medium was changed every 24 h. In addition, seven intervention regimens were designed to mimic TRE, ADF and CR in cultured cells (Fig. 1B). Depending on the regimens, the culture medium was changed every 12 or 24 h. Cell intervention was designed to be either short-term (9 days) or long-term (45 days). Cells in each group were passaged every 3 days and each well was maintained at a seeding density of 1 × 105 viable cells per well.
2.8 Cytokinesis-block micronucleus (CBMN) assay
At each sampling time (days 9 and 45), duplicate 400 μl subcultures were established in a 24-well plate (Costar) from the main cultures, at a concentration of 1 × 105 cells per well. Following a 2-hour incubation period, cytochalasin B (1.5 μg ml−1; Sigma) was added to block cytokinesis and rinsed with PBS after a further 24 hours. The cells were mounted onto glass slides and centrifuged using a cyto-centrifuge for 5 minutes at 1000 rpm. The final cell density per slide was kept between 0.5 × 105 and 1 × 105 cells. After drying briefly in air, the slides were fixed with 100% cold methanol at −20 °C for 15 minutes and stained with 10% Giemsa. The slides were washed twice with ddH2O and allowed to air dry and then cover slips were placed over the slides. In cisplatin-challenge assays, cells from the main cultures at day 9 or 45 were seeded in a 24-well plate at a concentration of 1 × 105 cells per well and were treated with 0.5 μg ml−1 cisplatin for 48 h. Then, the cells were harvested for the CBMN assay as described above. All biomarkers of the CBMN assay, including MN, nucleoplasmic bridge (NPB) and nuclear bud (NB) were scored under 1000× magnification with an optical microscope (Olympus, Tokyo, Japan). 300–1000 binucleated cells (BNCs) were scored per replicate and at least three independent experiments were carried out.
2.9 Quantification and statistical analysis
Values are mean ± standard error of the mean (s.e.m.) if not otherwise stated. All datasets were tested for normal distribution using the Shapiro–Wilk tests. Skewed data were log-transformed. For mice and cell experiments that have more than two treatments, each parameter was separately analyzed by one-way ANOVA followed by Tukey's multiple comparison test. For experiments that have two treatments, differences were evaluated using the unpaired (2-side) t test. All statistical analyses were performed using GraphPad 7.0.
3 Results
3.1 Food intake and body weight dynamics of mice intervened by different DR regimens
The effects of dietary intervention are often studied in the inbred C57BL/6 mice, which may compromise the generality and translational use of the experimental discoveries.33 Considering this, we used CF-1 in this study. A cohort of 54 CF-1 female and male mice at 3–5 months of age was established and the mice were randomly divided into four diet groups (AL, TRE, ADF and CR) and maintained for 4 weeks (Fig. 1A). We followed these mice longitudinally, with daily assessments of food consumed (Fig. 2A and B) and body weight (Fig. 3A and B). Both the female and male ADF mice learned quickly that food would not be continuously available and thus tended to eat more food in the feed days (Fig. 2A and B). However, most of the time, TRE mice did not recognize that food would not be continuously available because a small part of the given food usually used to be left uneaten at the end of daily TRE (Fig. 2A and B).
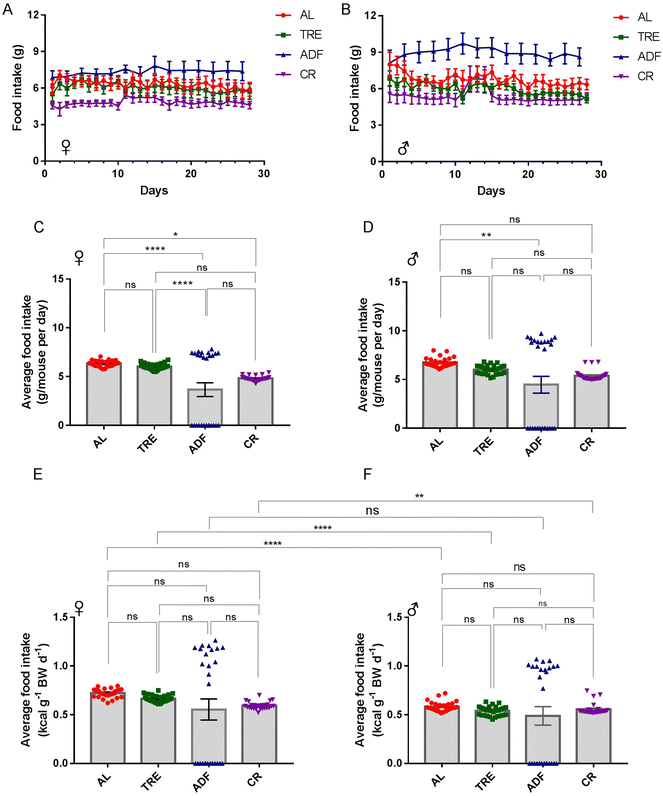 |
| Fig. 2 Estimated food consumption per mouse over the 4-week DR interval. (A and B) The food-intake trajectories of female and male mice from each of the four feeding regimens (n = 7–8 mice per cohort). Food intake of ADF mice in fasting days (0 g) was omitted for clarity. (C and D) Average daily food intake per female and male mouse (n = 7–8 mice per group). (E and F) Average daily food intake per female and male mouse relative to their body weight (BW) (n = 7–8 mice per group). Data are the mean ± s.e.m. Data are analyzed by one-way ANOVA with Tukey's multiple comparison tests (more than two treatments) or Student's t test (two treatments). ns = P > 0.05; *P < 0.05, **P < 0.01, ***P < 0.001, ****P < 0.0001. | |
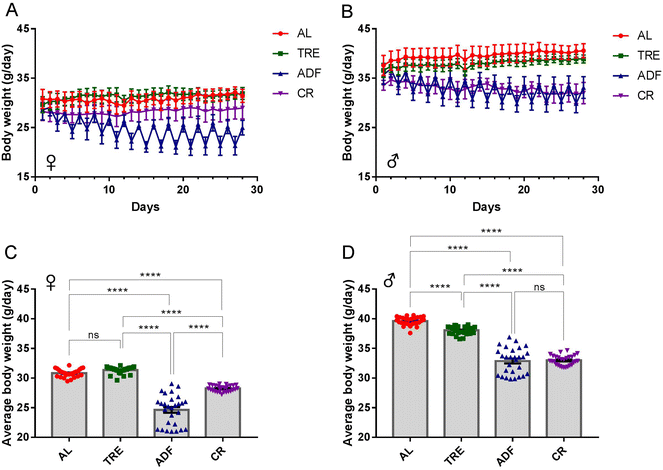 |
| Fig. 3 Estimated body weight per mouse over the 4-week DR interval. (A and B) The body-weight trajectories of female and male mice from each of the four feeding regimens (n = 7–8 mice per cohort). (C and D) Average daily body weight per female and male mouse (n = 7–8 mice per cohort). Data are the mean ± s.e.m. Data are analyzed by one-way ANOVA with Tukey's multiple comparison tests (more than two treatments). ns = P > 0.05; ****P < 0.0001. | |
Over the course of 4-week intervention, the average food intake of female AL mice was 6.34 ± 0.06 g per day (21.5 kcal per day; Fig. 2C). Compared to AL controls, the average food intake of female TRE mice was reduced by 4.9% (6.03 ± 0.06 g per day or 20.5 kcal per day; Fig. 2C). The average food intake at the feeding days of female ADF mice was 7.38 ± 0.07 g per day (25.1 kcal per day; Fig. 2C), an average increase of 16.4% compared to their AL counterparts. The average food intake of female CR mice was 4.82 ± 0.05 g per day (16.39 kcal per day; Fig. 2C), an average reduction of 24% (24% CR) compared to their AL counterparts. For males, the average food intake of AL mice was 6.76 ± 0.09 g per day (23.0 kcal per day; Fig. 2D), 12.1% higher than AL females. Compared to AL males, the average food intake of male TRE mice was reduced by 11.1% (6.01 ± 0.09 g per day or 20.4 kcal per day; Fig. 2D). The average food intake at the feeding days of male ADF mice was 8.95 ± 0.11 g per day (30.43 kcal per day; Fig. 2D), an average increase of 32.4% compared to their AL counterparts. The average food intake of male CR mice was 5.38 ± 0.10 g per day (18.29 kcal per day; Fig. 2D), an average reduction of 21.4% (21.4% CR) compared to their AL counterparts. The total amount of food consumed by ADF females and males was correspondingly 38.8% and 33.8% lower than their AL counterparts, suggesting that ADF was easier than CR to achieve high levels of food restriction in CF-1 mice.
Over the 4-week DR interval, the body-weight trajectories of each of the four feeding regimens were recorded (Fig. 3A and B). The body weight of AL males, but not AL females, gradually increased over the 4-week time course (∼0.1% weight gain for females and ∼6.9% weight gain for males; Fig. 3A and B). As expected, the body weight of both female and male ADF mice decreased rapidly at the fasting days and regained at the feeding days (Fig. 3A and B). The body-weight trajectories between 4-week TRE females (31.36 ± 0.11 g) and AL controls (30.83 ± 0.12 g) were not significantly different (Fig. 3A and C). While the body weight of female ADF mice (24.64 ± 0.48 g) was reduced by an average of 21.1% compared to that of AL control mice, the body weight of male CR mice (28.24 ± 0.10 g) was reduced by an average of 9.4% compared to that of AL control mice during the 4-week time course (Fig. 3C). For males, the difference in the body-weight trajectories between TRE mice and AL controls was steady over the 4-week time course (Fig. 3B). Overall, TRE caused a minor reduction of body weight compared to the AL diet (38.02 ± 0.13 g vs. 39.62 ± 0.13 g; Fig. 3D) in males. ADF and CR males gradually lost their weight, reaching a corresponding ∼7.2% and ∼4.7% of body weight at the end of the 4-week interval. ADF and CR males had a comparable average body weight (32.87 ± 0.41 g vs. 32.98 ± 0.16 g), which was significantly lower than AL mice (Fig. 3D).
In addition, we noted that there was a sex difference of weight loss in response to DR. For instance, under the ADF regimen, females (Fig. 3C) achieved a higher level of weight loss than males (Fig. 3D), compared to their corresponding AL counterparts. In contrast, males achieved a higher level of weight loss than females in the course of 4-week TRE. Although these three DR regimens were effective for weight loss, ADF produced superior changes in body weight to CR and TRE.
To avoid the confounding effects of weight change on food intake,32 we calculated the food consumption relative to the body weight of each mouse (Fig. 2E and F). The patterns of daily food consumption relative to the body weight across four diet regimens (Fig. 2E and F) were consistent with those of daily food consumption (Fig. 2C and D). While daily food consumption relative to the body weight was similar across the four feeding groups, one interesting finding was that daily food consumption relative to the body weight was significantly higher in females than in males under each diet regimen, except for ADF (Fig. 2E and F). These data indicate that the energy requirement per unit body weight is much greater in female CF-1 mice than in male CF-1 mice.
3.2 Sex and regimen-dependent effect of DR on spontaneous and induced MN
MN is a commonly observed biomarker of GIN and usually results from the mis-segregated chromosomal fragments or entire chromosomes.34 Micronucleation has much severe consequences on the affected chromosome and/or the host cells. Recent evidence supported that MN is also a strong mutator phenotype that can drive genetic heterogeneity through catastrophic events such as chromothripsis (a novel form of GIN in cancer cells) in the MNed chromosome.35 Besides, the presence of MN is linked to cellular senescence and apoptosis.34 For example, MN promotes inflammatory signaling by introducing double-stranded DNA into the cytosol, engaging the cGAS-STING anti-viral pathway, which is a driving force for cellular senescence and cancer.36 In chromosomally unstable tumor cells, the chronic activation of the cGAS-STING pathway in response to MN leads to noncanonical NF-κB signaling, thereby promoting cancer metastasis.36 Thus, there is renewed interest in studying MN. The bone marrow MN assay is an in vivo method for MN screening. Measuring the number of MN in mammalian red blood cells is relatively straightforward because PCEs (erythrocyte precursor cells that reside in the bone marrow) expel their nuclei during red blood cell development, leaving behind any MN that have formed (Fig. 4A).37
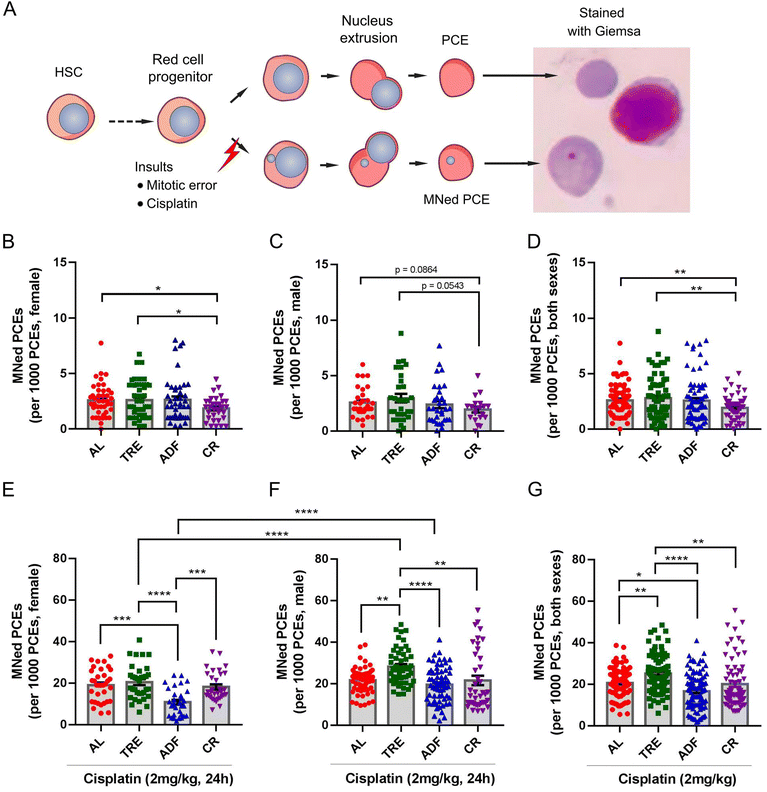 |
| Fig. 4 Shared and sex- and regimen-specific effects of DR on genome stability in CF-1 mice. (A) Scheme depicting the formation of micronucleated polychromatic erythrocytes (MNed-PCEs). Micronuclei (MN) generated by fragmentation or mis-segregation of chromosomes during erythrocyte maturation that remained in the erythrocyte after the extrusion of the main nucleus. These fragments can be stained with Giemsa. (B–D) Effects of different DR regimens on the spontaneous rate of MNed PCEs in females, males and both sexes (n = 3–4 mice per regimen). (E–G) Effects of different DR regimens on the cisplatin-induced rate of MNed PCEs in females, males and both sexes (n = 3–4 mice per regimen). Data are the mean ± s.e.m. Data are analyzed by one-way ANOVA with Tukey's multiple comparison tests (more than two treatments) or Student's t test (two treatments). *P < 0.05; **P < 0.01, ***P < 0.001, ****P < 0.0001. | |
We investigated the effects of DR on genome stability at the end of 4-week DR by the bone marrow MN assay. We analyzed female and male bone marrow in each regimen separately to allow for identification of sexually dimorphic phenotypes, given the disproportionate changes of food intake and body weight among females and males. The spontaneous MN frequency in the AL cohort did not exhibit a sex difference (females: 2.58 ± 0.22‰ vs. males: 2.57 ± 0.24‰). In female groups, we did not observe a difference between the AL and TRE regimens, or between the AL and ADF regimens. However, the CR regimen showed a 28.3% decrease in the frequency of MNed PCEs compared to the AL controls (1.85 ± 0.19‰ vs. 2.58 ± 0.22‰; P = 0.02, Fig. 4B). The effect of ADF on spontaneous MN in males was similar to that of control-fed mice (Fig. 4B). Although the male mice under TRE showed some increase in spontaneous MN relative to their AL counterparts, the increase did not reach significance (Fig. 4B). In contrast to females, CR did not significantly decrease the frequency of MNed PCEs compared to the AL controls (1.93 ± 0.26‰ vs. 2.57 ± 0.24‰; P = 0.086, Fig. 4C). These results suggested that CR has female-specific benefits in improving genome stability relative to controls. Considering both males and females together, a statistically significant effect of CR in decreasing the spontaneous MN was observed (P = 0.004, Fig. 4D). With the exception of CR, neither TRE nor ADF exerted beneficial effects on preventing spontaneous MN in both sexes.
We next assessed the effects of DR on MN induced by cisplatin, a well-recognized mutagen and chemotherapy drug able to induce sister-chromatid exchanges and DNA crosslinks38 and therefore MN.21 Exposing mice to a single 2 mg kg−1 dose of cisplatin for 24 h was sufficient to increase the bone marrow MN levels. We found that 2 mg kg−1 cisplatin induced a 7.3- and 8.4-fold increase in the MN frequency in female (Fig. 4E) and male mice (Fig. 4F), suggesting that there is no apparent sex different response to cisplatin-induced MN. Indeed, MN induced from cisplatin also did not exhibit a significant sex difference (females: 18.96 ± 1.34‰ vs. males: 21.62 ± 0.83‰; P = 0.078). In females, although CR reduced the spontaneous MN, it failed to protect against cisplatin-induced MN compared to AL controls (18.19 ± 1.36‰ vs. 18.96 ± 1.34‰; P = 0.675, Fig. 4E). In contrast, while ADF had no effect on spontaneous MN (Fig. 4A), it exhibited remarkable potential to decrease cisplatin-induced MN compared to AL controls (10.8 ± 1.17‰ vs. 18.96 ± 1.34‰; P < 0.001, Fig. 4E). In males, both ADF and CR had no effect on cisplatin-induced MN (Fig. 4F). Unexpectedly, after exposure to cisplatin, the frequency of MN in male TRE mice was 30.6% higher than that of male AL controls (28.23 ± 1.18‰ vs. 21.62 ± 0.83‰; P < 0.0001, Fig. 4F), suggesting that the TRE feeding exacerbates cisplatin-induced GIN. There was no negative effect of TRE feeding on cisplatin-induced MN in females (20.46 ± 1.42‰ vs. 18.96 ± 1.34‰; P = 0.845, Fig. 4E). Considering both males and females together, a statistically significant effect of ADF in decreasing cisplatin-induced MN (P < 0.01) and a statistically significant effect of TRE in enhancing cisplatin-induced MN (P < 0.05) were observed (P = 0.004, Fig. 4G).
We also compared the effects of TRE, ADF and CR on MN. In both females and males, the spontaneous MN was not significantly different between the TRE groups and the ADF groups or between the ADF groups and CR groups (Fig. 4B and C). Among the three intervention groups, CR feeding was superior to TRE with regard to providing protection against spontaneous MN, particularly in females (Fig. 4B–D). There was no significant difference in cisplatin-induced MN between the TRE and CR females (Fig. 4E). However, this is not the case for males as CR males had superior ability to protect against cisplatin-induced MN to TRE males (Fig. 4F). In the female cohort, ADF exhibited a superior effect to protect against cisplatin-induced MN to TRE and CR (Fig. 4E). In the male cohort, ADF and CR provided greater protection against cisplatin-induced MN than TRE (Fig. 4F). When males and females were considered separately or together, the difference of cisplatin-induced MN between TRE and ADF groups was constantly significant (Fig. 4E–G). In addition, ADF and CR exhibited a similar effect with respect to cisplatin-induced MN.
In addition, we assessed whether there is a sex difference in spontaneous and cisplatin-induced MN within each regimen. Within each group, there was no significant difference in spontaneous MN between females and males (Fig. 4B and C). Regarding cisplatin-induced MN, the sex-dependent difference was pronounced. Although the frequency of cisplatin-induced MN in male AL mice was only 14% higher than that of their female counterparts (P = 0.078; Fig. 4E and F), we found that the frequency of cisplatin-induced MN in male TRE mice and ADF mice was respectively 37.98% and 80.3% higher than that of their female counterparts (both P < 0.0001; Fig. 4E and F). The results of cisplatin-induced MN showed no difference between CR females and males. These data suggested that a 4-week course of TRE and ADF makes the male mice more sensitive to the genotoxicity of external mutagens.
Together, these results demonstrated that the effects of DR on spontaneous and cisplatin-induced MN depend on the sex and regimen. Importantly, the sex dimorphic effect of DR intervention, especially ADF, was more pronounced in regard to cisplatin-induced MN. Despite differences in the aspects of the feeding regimen and food intake, CR conferred an overall benefit to genome stability that is similar to ADF. Interestingly, our results also demonstrated that a reduction of spontaneous MN by one DR regimen would not be expected to correlate with the reduced rate of cisplatin-induced MN, or vice versa. Importantly, we challenged the paradigm that TRE is always beneficial for health by showing that cisplatin-induced MN was exacerbated in TRE males.
3.3 Development of in vitro DR mimetics
DR interventions in mice are limited by the fact that mice do not faithfully recapitulate human pathophysiology due to the extensive species-specific differences and the possible artifacts introduced by the laboratory environment. Given that some DR regimens exhibited excellent potential in reducing spontaneous or induced MN in CF-1 mice, we wanted to test whether this effect can be reproduced in human cells cultured in serum-based media. Since the concentrations of many nutrients in commonly used serum-based media are several fold higher than those in human and rodent plasma,39 we hypothesized that restricting the concentrations of serum and/or total nutrients, rather than one or more specific nutrients, is a feasible way to mimic in vivo DR regimens. Based on the feeding paradigms of the three in vivo DR regimens tested above, we developed a set of culture paradigms (Fig. 1B) to mimic TRE (#2 and #3), ADF (#4, #5 and #6) and CR (#7 and #8). We used the in vitro MN test to examine whether they could modify the rate of spontaneous or induced MN in HUVECs. In this assay, MN are formed in the interphase when acentric chromosomal fragments or whole chromosomes have failed to be incorporated into the nucleus at the completion of mitosis (Fig. 5A). In addition, this assay provides an opportunity to detect other forms of GIN like NB based on the nuclear morphology (Fig. 5A).
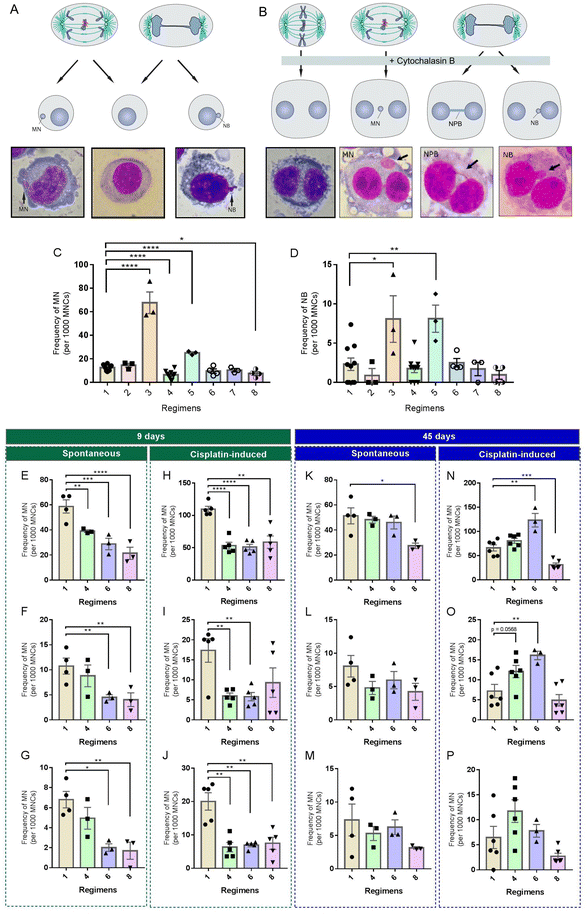 |
| Fig. 5 Effects of different in vitro DR mimetics on the genome stability of HUVECs. (A) Scheme depicting the fates of mitotic mis-segregated chromosomes at the end of cytokinesis. After mitosis, most of the ana-telophase lagging chromosomes would end up as micronuclei (MN). The ana-telophase bridged chromosomes will be broken by the mechanical stress and the broken ends will be identified as nuclear buds (NB). (B) Scheme depicting the fates of mitotic mis-segregated chromosomes in the cytokinesis-block micronucleus (CBMN) assay. In this assay, cytochalasin-B is added to block cytokinesis and induce binucleated cells. Lagging chromosomes formed during ana-telophase can be identified as MN, bridged chromosomes formed during ana-telophase can be identified as nucleoplasmic bridges (NPB) and mis-amplified DNA can be identified as NB in binucleated daughter cells. (C and D) Effects of in vitro DR mimetics on the spontaneous rate of MN and NB in HUVECs determined by the in vitro MN assay. (E–J) Effects of short-term in vitro DR mimetics on the frequencies of spontaneous (E–G) and cisplatin-induced (H–J) MN, NPB and NB in HUVECs determined by the CBMN assay. (K–P) Effects of long-term in vitro DR mimetics on the frequencies of spontaneous (K–M) and cisplatin-induced (N–P) MN, NPB and NB in HUVECs determined by the CBMN assay. Data are the mean ± s.e.m. Data are analyzed by one-way ANOVA with Tukey's multiple comparison tests (more than two treatments). *P < 0.05; **P < 0.01, ***P < 0.001, ****P < 0.0001. | |
Among these culture paradigms, 12.5% control medium, PBS (nutrition-free) or 12.5% control medium was used to mimic fasting, and 70% control medium or medium with 7% FBS was used to mimic 30% CR (Fig. 1B). HUVECs were used in our in vitro interventions. Firstly, HUVECs were cultured for a short term (9 days) in different media. Then, cells from each culture paradigm were collected to determine the frequency of morphological alterations in nuclei. We found that, compared to the control (paradigm #1), paradigms #3 and #5 significantly increased the frequency of MN (Fig. 5C) and NB (Fig. 5D), but paradigms #4 and #8 significantly decreased the frequency of MN (Fig. 5C). In addition, we found that paradigm #6 also displayed potential to decrease MN, although no significance was achieved (Fig. 5C).
Considering the potential of paradigms #4, #6 and #8 in decreasing nuclear alterations, we selected them as the key paradigms to determine their effects on spontaneous and cisplatin-induced GIN using the CBMN assay. In the CBMN assay, cytochalasin-B is added to block the cytokinesis of once-divided cells and induce binucleated cells. Therefore, ana-telophase lagging chromosomes can be identified as MN, ana-telophase bridged chromosomes can be identified as NPB and mis-amplified DNA can be identified as NB in binucleated daughter cells (Fig. 5B).40 Unlike the in vitro MN assay, which scores GIN in cells with different cell division kinetics, the CBMN assay restricts GIN scoring in once-divided cells (identified as binucleated cells) and can prevent the confounding effects caused by suboptimal or altered cell division kinetics.40
Upon a short-term (9 days) intervention, paradigms #6 and #8 displayed remarkable potential to reduce spontaneous MN (Fig. 5E), NPB (Fig. 5F) and NB (Fig. 5G) in HUVECs. Meanwhile, only paradigm #4 had potential to significantly decrease spontaneous MN (Fig. 5E). Also, we observed that, compared to the control, a short-term nutritional intervention of paradigms #4 and #6 had comparable potential to significantly reduce cisplatin-induced MN (Fig. 5H), NPB (Fig. 5I) and NB (Fig. 5J). Although paradigm #8 had potential to reduce cisplatin-induced MN (Fig. 5H) and NB (Fig. 5J), it had no apparent effect on cisplatin-induced NPB (Fig. 5I).
To further investigate a long-term effect of these culture paradigms on genome stability, HUVECs were intervened for 45 days. We found that paradigms #4 and #6 had lost their potential to reduce spontaneous MN (Fig. 5K), NPB (Fig. 5L) and NB (Fig. 5M) in HUVECs. Only paradigm #8 maintained its potential to significantly reduce MN (Fig. 5K). Unexpectedly, paradigms #4 or #6 had potential to increase cisplatin-induced MN (Fig. 5N), NPB (Fig. 5O) and NB (Fig. 5P) to a different extent in HUVECs upon a long-term intervention. Notably, there was a decreased level of MN induced by cisplatin in HUVECs intervened by paradigm #8 when compared to the control (Fig. 5N). We found that paradigm #8 also displayed potential to decrease cisplatin-induced NPB (Fig. 5O) and NB (Fig. 5P), although no significance was achieved.
Overall, these results show that the GIN-modulating effect of DR can be reproduced in vitro by culturing cells with modified concentrations of serum and/or diluted nutrients. Importantly, the effects of some in vitro DR mimetics on GIN become opposite under short-term and long-term conditions.
4 Discussion
A diverse body of data across the field of dietary intervention has established that DR has extensive benefits in health. Poor diet is a known contributor to the accelerated onset of aging and multiple diseases. Importantly, GIN is a hallmark and driver of aging and cancer. A logical next phase of the DR field is establishing whether and how DR regimens that have health benefits modulate GIN. A caveat relevant to most DR studies is that a variation in the experimental conditions, such as DR duration, time onset or diet composition limits the direct comparison of the results. Qualitative studies that directly compare the effects of different DR regimens in the single study will help to ascertain whether one regimen is superior to the others in maintaining genome stability. In the present study, we used both male and female CF-1 mice to assess whether three DR regimens known to exert beneficial effects on health, TRE, ADF and CR, influence the spontaneous and cisplatin-induced MN in the bone marrow of male and female CF-1 mice.
Since most previous studies using mice fail to consider sex as a variable, female and male mice were included in the present study. For the first time, we have questioned whether sex influences the response of genome stability to DR. Our results suggest that DR effects on genome stability are not universal. We found that TRE, ADF and CR influence the spontaneous and cisplatin-induced MN in distinct ways and the effects are sex-dependent. First, we found that CR significantly decreases the spontaneous MN in female mice compared to their AL counterparts, while the spontaneous MN in CR males are slightly decreased compared to their AL counterparts. Second, we found that ADF significantly decreases cisplatin-induced MN in female mice compared to their AL counterparts, while the cisplatin-induced MN in CR males remain the same. Third, we observed a significant increase of MN in TRE males following cisplatin exposure compared to their AL counterparts, while TRE females showed no significant increase in MN following cisplatin exposure. Overall, it can be argued that female mice from either DR regimen exhibit more pronounced ability to maintain genome stability than males. These data add to a growing body of evidence that molecular responses to DR are sex specific,16 which has important implications for clinical translation of the DR regimens into medical treatment for humans.
The mechanisms through which females sustain their genome more stable than males under DR intervention, however, remain largely unknown. Since the baseline MN in CF-1 mice did not exhibit any sex disparity, the female-biased ability to maintain genome stability under DR conditions may not be due to the constituted disparity in genome preserving mechanisms between females and males. For example, activation of sirtuin family proteins is a universally attributed mechanism to exert the beneficial effects of DR.1 The members of sirtuin family proteins have been considered as guardians of the genome. For instance, SIRT1 and SIRT6 are the master regulators of the chromatin structure, DNA repair and chromosome segregation.41,42 Thus, we speculate that sirtuins may be key players underlying the sex-varied effects of DR on genome stability. In line with this speculation, it was recently reported that 20% CR or a low-protein diet significantly induces the expression of SIRT1 specifically in the hippocampus of female mice but not in male mice.43 To the best of our knowledge, to date, no study has reported convincing evidence to support that SIRT6 displays a sex-specific response to DR and this is in need of further investigation. Taken together, the disproportionate impact of different DR regimens on the female and male genome stability provides a rich opportunity for future investigation into the influence of sex on GIN-related pathophysiological consequences.
While the argument of multiple DNA repair pathways under CR conditions44 may lower the acquisition of DNA mutation, CR has recently found to decrease mutation retention in mice intestine by increasing the number of competitive wildtype stem cells to displace the mutant stem cells.45 All cycling cells in the hematopoietic system are originated from the differentiation of hematopoietic stem cells (HSCs) residing in bone marrow. Competition by HSCs for occupation of bone marrow is mediated by p53.46 If mutations confer high fitness, these affected HSCs will outcompete the less fit wildtype HSCs and produce a clone of mutated progeny.11 It has been shown that CR and prolonged fasting improve HSCs’ self-renewal and prevent myeloid-biased differentiation in a manner depending on the length of CR and the age of CR onset.47,48 Drawing from our observation of decreased MN upon CR, we argue that CR may compromise the ability of MN-prone HSCs to compete with wildtype HSCs, thereby reducing the level of MN positive PCEs derived from these mutant HSCs. Further ongoing studies are needed to address whether such mechanism does exist in the CR-treated hematopoietic system, and if so, whether there is a sex dimorphism underlying this issue.
While the detailed mechanisms underlying this female-biased ability to maintain genome stability under DR conditions remain to be addressed, we noted that, in CR groups, the female biased decrease of spontaneous MN is consistent with a more pronounced reduction in daily food intake in females (24% reduction in females vs. 21% reduction in males). Similarly, in ADF groups, the female biased decrease of cisplatin-induced MN is consistent with a more pronounced reduction in daily food intake in females (38.8% reduction in females vs. 33.8% reduction in males). This relationship clarifies the importance to investigate whether the degree of energy reduction is one determinate of the anti-GIN effect of CR and ADF. In support of this, a previous study has found that the metabolic and lifespan-prolonging benefits of 20% CR in C57BL/6 mice did not become further pronounced under 40% CR.49 Moreover, we found that CR has a marginally significant effect on reducing spontaneous MN in males. Therefore, additional research will be required to define the optimal degree of energy reduction for preserving genome stability in each sex.
Our results showed that the effects on spontaneous and cisplatin-induced MN in each intervention group are not correlated, indicating that the molecular determinants of genome stability under these two conditions differ. Among the three tested DR regimens, ADF is the only regimen able to protect against MN induced by cisplatin. Cisplatin is one of the most widely used anticancer drugs and it mainly impairs DNA function by generating monoadducts and DNA crosslinks.38 Like many other DNA-damaging agents, cisplatin not only kills cancer cells but also induces massive damage to normal cells. As strategies to treat cancer have focused primarily on the killing of cancer cells, we have previously found that several phytochemicals such as geraniin and the geraniin-containing herbal drug—Phyllanthus emblica—are able to protect normal but not cancer cells against spontaneous and chemotherapy-induced GIN,50–54 suggesting a possibility that changes in diet could improve a chemotherapy drug's side effects on normal cells. Indeed, short term starvation has potential to protect normal cells against the cytotoxicity of high-dose chemotherapy55,56 and multiple cycles of fasting augment the anticancer efficacy of certain chemotherapy drugs via synergistically promoting DNA breaks in cancer cells.57 Drawing from our observation of decreased cisplatin-induced MN upon the CR regimen, we speculate that ADF is a readily accessible way to reduce the genotoxicity of chemotherapy drugs in blood cells. In agreement with our results, prolonged fasting (lasting 48 hours) leads to a decrease of DNA damage caused by cyclophosphamide in mice leukocytes and bone marrow cells.48
By strengthening the circadian rhythms of energy intake and metabolism, TRE has the potential to reduce the risk of metabolic diseases.29 Although ADF extends the lifespan of male C57BL/6J mice, only a few molecular, cellular, physiological and histopathological aging features can be delayed by ADF,58 suggesting that the benefits of ADF are not as large as full CR. Our results demonstrated that TRE is effective at reducing body weight of male but not female CF-1 mice and TRE has no apparent effect on the spontaneous rate of MN in both sexes. An unexpected finding in the current study is that TRE increases the predisposition of males, but not females, to the genotoxicity of cisplatin. This result stands in contrast to multiple studies having unambiguously documented beneficial effects of TRE and suggests that TRE can have a negative effect on the genome stability of male mice. Since TRE is also associated with reduced calorie intake and minor weight loss in males rather than females, we cannot tease apart the effects of reduced calorie intake or weight loss versus TRE per se on cisplatin's genotoxicity observed in males. As no mechanistic explanation for this negative effect is apparent at present, a logical extension of our observation is aimed at understanding how TRE exacerbates cisplatin's genotoxicity in male mice. In light of this finding, it is important to consider the genomic safety of TRE in human studies. While sex is currently not taken into consideration in DR trials,1 our findings also emphasize the need to consider the sex difference in the experimental design of future trials. Since our results reveal that 12 h once-a-day TRE exacerbates cisplatin-induced MN, future studies are needed to determine the impact of other forms of TRE on genome stability, such as TRE with two or more isocaloric feeding intervals per day or with different feeding windows (4–12 h).
Although animal models are valuable for exploring the health benefits of DR, dissecting the underlying mechanisms in animal models is challenging. To overcome this limitation, in vitro models should be developed to mimic different DR regimens, which was another goal of our present study. One well-established in vitro CR mimetic using serum was obtained from CR-fed rodents or monkeys to culture cell lines.59 Moreover, the impact of CR on the budding yeast is typically investigated in glucose-restricted cultures.60 To investigate the responses of human stem cells to CR, stem cells are cultured under 70–90% glucose reduction conditions, which have been found to simulate the moderately stressful conditions induced by CR in vivo.61 Previously, we have shown that culturing HUVECs under 75% glucose reduction conditions for 24 hours significantly reduces the spontaneous rate of MN.20 Coupling this finding to our current observation that CR decreases spontaneous MN in CF-1 mice, we propose that glucose reduction recapitulates the features of CR in maintaining genome stability.
Since glucose is just one of the numerous macronutrients restricted by CR, we aimed to develop some alternatives that can mimic different DR regimens in the present study. We found that an in vitro TRE-mimetic paradigm (#3) and an in vitro ADF-mimetic paradigm (#5) have potential to increase the spontaneous rate of MN and NB in HUVECs, suggesting that PBS is not feasible to be considered as an in vitro fasting mimetic. The other two ADF-mimetic paradigms (#4 and #6) exhibit an ability to reduce the spontaneous or cisplatin-induced MN, NPB and/or NB after a short-term intervention (9 days). After a long-term intervention (45 days), however, they not only fail to reduce the spontaneous GIN events, but also tend to promote cisplatin-induced GIN. Notably, a CR-mimetic paradigm (#8) exhibits a strong ability to protect against the spontaneous or cisplatin-induced GIN events under both short- and long-term interventions, suggesting that a medium with 30% reduction of serum can be very effective at recapitulating the anti-GIN feature of CR in cultured cells. While the specific mechanisms responsible for the anti-GIN effect of serum reduction remain elusive, it is worth noting that cells cultured under paradigm #8 had a decreased rate of cell division (data not shown). Since cell division is required for a GIN-positive cell to form MN in the CBMN assay, we cannot rule out that paradigm #8 reduced the rate of MN by inhibiting the division of GIN-positive cells. Reducing the level of serum in cell cultures has been shown to effectively recapitulate the in vivo features of DR. For example, it has been reported that serum reduction (90% reduction) alone or in combination with glucose restriction protects normal but not cancer cells against the cytotoxicity of high-dose chemotherapy, as what has been observed in mice upon short-term starvation.55
These in vitro findings have obvious future applications. Since cell lines have virtually limitless replicative potential, they have been widely used in routine biomedical studies with different purposes. For example, human normal cell lines are a valuable component of genotoxicity testing62 and human tumor-derived cells have a very important role in the fundamental discoveries of cancer biology and development of new cancer therapeutics.63 One limitation in using cell lines under these conditions, however, is the gradual acquisition of GIN during the in vitro passaging in synthetic culture media.62 Genetic heterogeneity deriving from GIN could potentially influence the results and reproducibility of genotoxicity testing62 and be linked to a differential anti-cancer drug response.64 One deep reason underpinning the acquisition of GIN may be that the concentrations of some key metabolic nutrients in traditional synthetic culture media are non-physiologically high, which could profoundly affect cellular metabolism.39 Given that efforts have been made to adjust the concentrations of media components to imitate real physiological conditions,65,66 our findings suggest that reducing the level of serum from the standard 10% to 7% could be considered as a useful modification to mitigate the acquisition of GIN in cell lines. In addition, long-term cultured human pluripotent stem cells frequently acquire GIN, which has raised concerns for their safety in cellular therapies and regenerative medicine.67 Our findings can provide novel insight into designing new culture paradigms to preserve genome stability or improving mitotic fidelity in human pluripotent stem cells.
Although our results were reproduced in vitro and in vivo, their potential relevance to humans is unknown. In humans, only a few studies have assessed the impact of DR on genetic materials. Nonetheless, clinical evidence supports the idea that DR may stabilize human DNA. For example, a study of 48 overweight individuals showed that DNA damage in blood cells measured by the comet assay is significantly reduced from the baseline in 25% CR, 12.5% CR with structured exercise, and very a low-calorie diet group after 6-month intervention.68 Compared with controls, these interventions caused 10–13.9% weight loss from the baseline to month 6. There is no question that overweight is associated with increased DNA damage that can be reduced after weight loss in obese individuals.69 Thus, we cannot yet differentiate whether the decrease of DNA damage in DR-intervened individuals68 results from modulating DNA repair pathways or from the secondary effects induced by weight loss.
Strengths of this study design for evaluating the effect of DR on genome stability are two-fold: (1) directly compared the effects of different DR regimens in the single study in mice of both sexes, which would help to ascertain whether one regimen is superior to the others in maintaining genome stability and identify new factors contributing to the preservation a genome more stable in one sex than the other; (2) developed a set of cell culture paradigms to faithfully recapitulate the key effects of these DR regimens on genome stability and these in vitro DR mimetics could be adopted to study the underlying molecular mechanisms. Our study has several apparent limitations. We only used one mouse strain and these findings may not be representative of other mouse strains. There could be strain-specific responses to dietary intervention33,70 worth studying further. We only reported the differences in genome stability at one time point and these might vary with a longer intervention period. Further work should examine whether further changes would arise during a longer intervention. We only examined the DR effects on genome stability in bone marrow. Since the extent of sex difference depends on the tissue involved,71 further studies are needed to explore whether these effects have tissue-specific variations.
In conclusion, our present study adds the first evidence, to our knowledge, to shed light on the sex-biased effect of different DR regimens on genome stability, an important experimental phenotype of DR that deserves some detailed mechanistic follow-up. Additional integrated multi-omics studies will be required to fully elucidate the underlying mechanisms, which are likely to promote coordinated effects on multiple anti-GIN pathways in a sex- and regimen-specific manner. While the weight loss or correcting an existing metabolic impairment is an active area of investigation in human clinical trials,10 our results clarify the importance of combining basic and human studies to begin to examine the effect of DR on genome stability, a metric that determines the rate of human aging and risk of multiple diseases. Translationally, our findings emphasize the need to consider sex as a potential variable in pre-clinical and clinical trials in the field of DR. Our results also point out that the DR field should not only focus on the effects of DR on metabolic health, but also should pay attention to how DR regulates genomic health. Finally, we develop several culture paradigms to closely recapitulate the positive and negative effects of different DR regimens on genome stability, suggesting that the beneficial and detrimental effects of DR on genome stability may be conserved in mice and humans. Further studies are needed to delineate whether these culture paradigms recapitulate other in vivo features of DR, so that these in vitro DR mimetics can be widely adopted to study the molecular mechanisms underlying the beneficial and adverse effects of DR.
Author contributions
Xihan Guo: conception; methodology; data curation; validation; writing – original draft; writing – review and editing; funding acquisition; supervision. Fuping Su: methodology; data curation; validation. Yue Gao: methodology; data curation; validation. Liyan Tang: methodology; data curation; validation. Xixi Yu: methodology; data curation; validation. Jiangli Zi: methodology; data curation; validation. Yingshui Zhou: methodology; data curation; validation. Han Wang: writing – review and editing. Jinglun Xue: conception; resource. Xu Wang: conception; resource; funding acquisition; supervision; writing – review and editing.
Conflicts of interest
There are no conflicts to declare.
Acknowledgements
The authors thank Jianfei Li and Cheng Zhang for technical assistance and thank Xueqin Hu, Yanan Huang, Yizhen Jia and Lingzhi Li for their help in taking care of the mice. This work is funded by the National Natural Science Foundation of China (no. 31900410 and 32260148 to X. G. and no. 31860301 to X. W.), the Yunnan Fundamental Research Projects (no. 202001AU070055 and 202101AT070112 to X. G.) and the Outstanding Young Academics Award of Yunnan Normal University (2019, to X. G.).
References
- Q. Wu, Z.-J. Gao, X. Yu and P. Wang, Dietary regulation in health and disease, Signal Transduction Targeted Ther., 2022, 7, 252 CrossRef PubMed.
- L. Valdés, A. Cuervo, N. Salazar, P. Ruas-Madiedo, M. Gueimonde and S. González, The relationship between phenolic compounds from diet and microbiota: impact on human health, Food Funct., 2015, 6, 2424–2439 RSC.
- F. Rul, C. Béra-Maillet, M. C. Champomier-Vergès, K. E. El-Mecherfi, B. Foligné, M. C. Michalski, D. Milenkovic and I. Savary-Auzeloux, Underlying evidence for the health benefits of fermented foods in humans, Food Funct., 2022, 13, 4804–4824 RSC.
- G. Wu, Dietary protein intake and human health, Food Funct., 2016, 7, 1251–1265 RSC.
- N. Kanarek, B. Petrova and D. M. Sabatini, Dietary modifications for enhanced cancer therapy, Nature, 2020, 579, 507–517 CrossRef CAS PubMed.
- M. Dehghan, A. Mente, X. Zhang, S. Swaminathan, W. Li, V. Mohan, R. Iqbal, R. Kumar, E. Wentzel-Viljoen, A. Rosengren, L. I. Amma, A. Avezum, J. Chifamba, R. Diaz, R. Khatib, S. Lear, P. Lopez-Jaramillo, X. Liu, R. Gupta, N. Mohammadifard, N. Gao, A. Oguz, A. S. Ramli, P. Seron, Y. Sun, A. Szuba, L. Tsolekile, A. Wielgosz, R. Yusuf, A. Hussein Yusufali, K. K. Teo, S. Rangarajan, G. Dagenais, S. I. Bangdiwala, S. Islam, S. S. Anand, S. Yusuf, R. Diaz, A. Orlandini, B. Linetsky, S. Toscanelli, G. Casaccia, J. M. M. Cuneo, O. Rahman, R. Yusuf, A. K. Azad, K. A. Rabbani, H. M. Cherry, A. Mannan, I. Hassan, A. T. Talukdar, R. B. Tooheen, M. U. Khan, M. Sintaha, T. Choudhury, R. Haque, S. Parvin, A. Avezum, G. B. Oliveira, C. S. Marcilio, A. C. Mattos, K. Teo, S. Yusuf, J. Dejesus, D. Agapay, T. Tongana, R. Solano, I. Kay, S. Trottier, J. Rimac, W. Elsheikh, L. Heldman, E. Ramezani, G. Dagenais, P. Poirier, G. Turbide, D. Auger, A. L. De Bluts, M. C. Proulx, M. Cayer, N. Bonneville, S. Lear, D. Gasevic, E. Corber, V. de Jong, I. Vukmirovich, A. Wielgosz, G. Fodor, A. Pipe, A. Shane, F. Lanas, P. Seron, S. Martinez, A. Valdebenito, M. Oliveros, L. Wei, L. Lisheng, C. Chunming, W. Xingyu, Z. Wenhua, Z. Hongye, J. Xuan, H. Bo, S. Yi, B. Jian, Z. Xiuwen, C. Xiaohong, C. Tao, C. Hui, D. Qing, C. Xiaoru, H. Xinye, L. Jian, L. Juan, L. Xu, R. Bing, W. Wei, W. Yang, Y. Jun, Z. Yi, Z. Manlu, L. Fanghong, W. Jianfang, L. Yindong, H. Yan, Z. Liangqing, G. Baoxia, L. Xiaoyang, Z. Shiying, B. Rongwen, T. Xiuzhen, L. Dong, C. Di, W. Jianguo, X. Yize, L. Tianlu, Z. Peng, D. Changlin, L. Ning, M. Xiaolan, Y. Yuqing, L. Rensheng, F. Minfan, H. Jing, L. Yu, X. Xiaojie, Z. Qiang, P. Lopez-Jaramillo, P. A. C. Lopez, R. Garcia, L. J. A. Jurado, D. Gómez-Arbeláez, J. F. Arguello, R. Dueñas, S. Silva, L. P. Pradilla, F. Ramirez, D. I. Molina, C. Cure-Cure, M. Perez, E. Hernandez, E. Arcos, S. Fernandez, C. Narvaez, J. Paez, A. Sotomayor, H. Garcia, G. Sanchez, T. David, A. Rico, P. Mony, M. Vaz, A. V. Bharathi, S. Swaminathan, K. S. A. V. Kurpad, K. G. Jayachitra, N. Kumar, H. A. L. Hospital, V. Mohan, M. Deepa, K. Parthiban, M. Anitha, S. Hemavathy, T. Rahulashankiruthiyayan, D. Anitha, K. Sridevi, R. Gupta, R. B. Panwar, I. Mohan, P. Rastogi, S. Rastogi, R. Bhargava, R. Kumar, J. S. Thakur, B. Patro, P. V. M. Lakshmi, R. Mahajan, P. Chaudary, V. R. Kutty, K. Vijayakumar, K. Ajayan, G. Rajasree, A. R. Renjini, A. Deepu, B. Sandhya, S. Asha, H. S. Soumya, R. Kelishadi, A. Bahonar, N. Mohammadifard, H. Heidari, K. Yusoff, T. S. T. Ismail, K. K. Ng, A. Devi, N. M. Nasir, M. M. Yasin, M. Miskan, E. A. Rahman, M. K. M. Arsad, F. Ariffin, S. A. Razak, F. A. Majid, N. A. Bakar, M. Y. Yacob, N. Zainon, R. Salleh, M. K. A. Ramli, N. A. Halim, S. R. Norlizan, N. M. Ghazali, M. N. Arshad, R. Razali, S. Ali, H. R. Othman, C. Hafar, A. Pit, N. Danuri, F. Basir, S. N. A. Zahari, H. Abdullah, M. A. Arippin, N. A. Zakaria, I. Noorhassim, M. J. Hasni, M. T. Azmi, M. I. Zaleha, K. Y. Hazdi, A. R. Rizam, W. Sazman, A. Azman, R. Khatib, U. Khammash, A. Khatib, R. Giacaman, R. Iqbal, A. Afridi, R. Khawaja, A. Raza, K. Kazmi, W. Zatonski, A. Szuba, K. Zatonska, R. Ilow, M. Ferus, B. Regulska-Ilow, D. Rózanska, M. Wolyniec, Alkamel, M. Ali, M. A. Kruger, H. H. Voster, A. E. Schutte, E. Wentzel-Viljoen, F. C. Eloff, H. de Ridder, H. Moss, J. Potgieter, A. A. Roux, M. Watson, G. de Wet, A. Olckers, J. C. Jerling, M. Pieters, T. Hoekstra, T. Puoane, E. Igumbor, L. Tsolekile, D. Sanders, P. Naidoo, N. Steyn, N. Peer, B. Mayosi, B. Rayner, V. Lambert, N. Levitt, T. Kolbe-Alexander, L. Ntyintyane, G. Hughes, R. Swart, J. Fourie, M. Muzigaba, S. Xapa, N. Gobile, K. Ndayi, B. Jwili, K. Ndibaza, B. Egbujie, A. Rosengren, K. B. Boström, A. Gustavsson, M. Andreasson, M. Snällman, L. Wirdemann, A. Oguz, N. Imeryuz, Y. Altuntas, S. Gulec, A. Temizhan, K. Karsidag, K. B. T. Calik, A. A. K. Akalin, O. T. Caklili, M. V. Keskinler, A. N. Erbakan, A. M. Yusufali, W. Almahmeed, H. Swidan, E. A. Darwish, A. R. A. Hashemi, N. Al-Khaja, J. M. Muscat-Baron, S. H. Ahmed, T. M. Mamdouh, W. M. Darwish, M. H. S. Abdelmotagali, S. A. O. Awed, G. A. Movahedi, F. Hussain, H. Al Shaibani, R. I. M. Gharabou, D. F. Youssef, A. Z. S. Nawati, Z. A. R. A. Salah, R. F. E. Abdalla, S. M. Al Shuwaihi, M. A. Al Omairi, O. D. Cadigal, R. S. Alejandrino, J. Chifamba, L. Gwaunza, G. Terera, C. Mahachi, P. Murambiwa, T. Machiweni and R. Mapanga, Associations of fats and carbohydrate intake with cardiovascular disease and mortality in 18 countries from five continents (PURE): a prospective cohort study, Lancet, 2017, 390, 2050–2062 CrossRef CAS PubMed.
- C. L. Green, D. W. Lamming and L. Fontana, Molecular mechanisms of dietary restriction promoting health and longevity, Nat. Rev. Mol. Cell Biol., 2022, 23, 56–73 CrossRef CAS PubMed.
- C. M. McCay, L. A. Marynard, G. Sperling and L. L. Bames, Retarded growth, life span, ultimate body size and age changes in the albino rat after feeding diets restricted in calories, J. Nutr., 1939, 18, 1–13 CrossRef CAS.
- V. D. Longo, M. Di Tano, M. P. Mattson and N. Guidi, Intermittent and periodic fasting, longevity and disease, Nat. Aging, 2021, 1, 47–59 CrossRef PubMed.
- K. A. Varady, S. Cienfuegos, M. Ezpeleta and K. Gabel, Clinical application of intermittent fasting for weight loss: progress and future directions, Nat. Rev. Endocrinol., 2022, 18, 309–321 CrossRef PubMed.
- X. Dai and X. Guo, Decoding and rejuvenating human ageing genomes: Lessons from mosaic chromosomal alterations, Ageing Res. Rev., 2021, 68, 101342 CrossRef CAS PubMed.
- D. Hanahan and R. A. Weinberg, Hallmarks of cancer: The next generation, Cell, 2011, 144, 646–674 CrossRef CAS PubMed.
- C. López-Otín, M. A. Blasco, L. Partridge, M. Serrano and G. Kroemer, The hallmarks of aging, Cell, 2013, 153, 1194–1217 CrossRef PubMed.
- B. Laffon, S. Bonassi, S. Costa and V. Valdiglesias, Genomic instability as a main driving factor of unsuccessful ageing: potential for translating the use of micronuclei into clinical practice, Mutat. Res., Rev. Mutat. Res., 2021, 787, 108359 CrossRef CAS PubMed.
- F. Mauvais-Jarvis, A. P. Arnold and K. Reue, A guide for the design of pre-clinical studies on sex differences in metabolism, Cell Metab., 2017, 25, 1216–1230 CrossRef CAS PubMed.
- A. E. Kane, D. A. Sinclair, J. R. Mitchell and S. J. Mitchell, Sex differences in the response to dietary restriction in rodents, Curr. Opin. Physiol., 2018, 6, 28–34 CrossRef PubMed.
- J. A. Clayton and F. S. Collins, Policy: NIH balance sex in cell and animal studies, Nature, 2014, 509, 282–283 CrossRef PubMed.
- X. Guo, J. Ni, Y. Zhu, T. Zhou, X. Ma, J. Xue and X. Wang, Folate deficiency induces mitotic aberrations and chromosomal instability by compromising the spindle assembly checkpoint in cultured human colon cells, Mutagenesis, 2017, 32, 547–560 CrossRef CAS PubMed.
- X. Guo, X. Dai, J. Ni, N. Cao, G. Yang, J. Xue and X. Wang, High concentration of sugars is genotoxic to folate-deficient cells, Mutat. Res., Fundam. Mol. Mech. Mutagen., 2019, 814, 15–22 CrossRef CAS PubMed.
- T. Lyu, H. Zhou, Y. Wang, M. Jiang, Q. Tao, J. Chen, Y. Guo, Q. Zhang, X. Wang and X. Guo, High-dose metformin induces a low-glucose dependent genotoxic stress, Food Chem. Toxicol., 2022, 165, 113129 CrossRef CAS PubMed.
- L. Yan, Z. Zhao, X. Wang, T. Lyu, J. Li, Y. Qi, X. Wang and X. Guo, Short-term in vitro glutamine restriction differentially impacts the chromosomal stability of transformed and non-transformed cells, Mutagenesis, 2020, 35, 425–435 CrossRef CAS PubMed.
- X. Hu, X. Guo, J. Ni, H. Wang, N. Cao, Z. Liang and X. Wang, High homocysteine promotes telomere dysfunction and chromosomal instability in human neuroblastoma SH-SY5Y cells, Mutat. Res., Genet. Toxicol. Environ. Mutagen., 2020, 854–855, 503197 CrossRef CAS PubMed.
- X. Guo, Y. Qi, J. Li, H. Fan, L. Yang, X. Wu, J. Ni, H. Wang and X. Wang, A comprehensive study of the genotoxic and anti-genotoxic effects of homocysteine in HUVECs and mouse bone marrow cells, Food Chem. Toxicol., 2021, 156, 112518 CrossRef CAS PubMed.
- J. Li, C. Zhang, L. Li, X. Hu, Y. Jia, Y. Huang, T. Lyu, X. Wang and X. Guo, Folate deficiency enhances the in vitro genotoxicity of bile acids in human colon and liver cells, Mutagenesis, 2022, 37, 34–43 CrossRef CAS PubMed.
- C. Hine, E. Harputlugil, Y. Zhang, C. Ruckenstuhl, B. C. Lee, L. Brace, A. Longchamp, J. H. Treviño-Villarreal, P. Mejia, C. K. Ozaki, R. Wang, V. N. Gladyshev, F. Madeo, W. B. Mair and J. R. Mitchell, Endogenous hydrogen sulfide production is essential for dietary restriction benefits, Cell, 2015, 160, 132–144 CrossRef CAS PubMed.
- X. Guo, J. Ni, X. Dai, T. Zhou, G. Yang, J. Xue and X. Wang, Biphasic regulation of spindle assembly checkpoint by low and high concentrations of resveratrol leads to the opposite effect on chromosomal instability, Mutat. Res., Genet. Toxicol. Environ. Mutagen., 2018, 825, 19–30 CrossRef CAS PubMed.
- R. de Cabo and M. P. Mattson, Effects of intermittent fasting on health, aging, and disease, N. Engl. J. Med., 2019, 381, 2541–2551 CrossRef CAS PubMed.
- H. Sudo and A. Kubo, The aneugenicity of ketone bodies in colon epithelial cells is mediated by microtubule hyperacetylation and is blocked by resveratrol, Int. J. Mol. Sci., 2021, 22, 9397 CrossRef CAS PubMed.
- M.-D. Li, Clock-modulated checkpoints in time-restricted eating, Trends Mol. Med., 2022, 28, 25–35 CrossRef CAS PubMed.
- S. J. Mitchell, M. Bernier, J. A. Mattison, M. A. Aon, T. A. Kaiser, R. M. Anson, Y. Ikeno, R. M. Anderson, D. K. Ingram and R. de Cabo, Daily fasting improves health and survival in male mice independent of diet
composition and calories, Cell Metab., 2019, 29, 221–228 CrossRef CAS PubMed.
- V. D. Longo and R. M. Anderson, Nutrition, longevity and disease: From molecular mechanisms to interventions, Cell, 2022, 185, 1455–1470 CrossRef CAS PubMed.
- C. Allard, P. Zizzari, C. Quarta and D. Cota, Food intake and body weight in rodent studies: the devil is in the details, Nat. Metab., 2022, 4, 1424–1426 CrossRef PubMed.
- S. Roy, M. B. Sleiman, P. Jha, J. F. Ingels, C. J. Chapman, M. S. McCarty, J. D. Ziebarth, M. Hook, A. Sun, W. Zhao, J. Huang, S. M. Neuner, L. A. Wilmott, T. M. Shapaker, A. G. Centeno, D. G. Ashbrook, M. K. Mulligan, C. C. Kaczorowski, L. Makowski, Y. Cui, R. W. Read, R. A. Miller, K. Mozhui, E. G. Williams, S. Sen, L. Lu, J. Auwerx and R. W. Williams, Gene-by-environment modulation of lifespan and weight gain in the murine BXD family, Nat. Metab., 2021, 3, 1217–1227 CrossRef CAS PubMed.
- X. Guo, J. Ni, Z. Liang, J. Xue, M. F. Fenech and X. Wang, The molecular origins and pathophysiological consequences of micronuclei: New insights into an age-old problem, Mutat. Res., Rev. Mutat. Res., 2019, 779, 1–35 CrossRef CAS PubMed.
- X. Guo, X. Dai, X. Wu, N. Cao and X. Wang, Small but strong: Mutational and functional landscapes of micronuclei in cancer genomes, Int. J. Cancer, 2021, 148, 812–814 CrossRef CAS PubMed.
- X. Guo, H. Hintzsche, W. Xu, J. Ni, J. Xue and X. Wang, Interplay of cGAS with micronuclei: Regulation and diseases, Mutat. Res., Rev. Mutat. Res., 2022, 790, 108440 CrossRef CAS PubMed.
- G. Balmus, N. A. Karp, B. L. Ng, S. P. Jackson, D. J. Adams and R. E. McIntyre, A high-throughput in vivo micronucleus assay for genome instability screening in mice, Nat. Protoc., 2015, 10, 205–215 CrossRef PubMed.
- S. Rottenberg, C. Disler and P. Perego, The rediscovery of platinum-based cancer therapy, Nat. Rev. Cancer, 2021, 21, 37–50 CrossRef CAS PubMed.
- S. Lagziel, E. Gottlieb and T. Shlomi, Mind your media, Nat. Metab., 2020, 2, 1369–1372 CrossRef PubMed.
- M. Fenech, Cytokinesis-block micronucleus cytome assay, Nat. Protoc., 2007, 2, 1084–1104 CrossRef CAS PubMed.
- A. Korotkov, A. Seluanov and V. Gorbunova, Sirtuin 6: linking longevity with genome and epigenome stability, Trends Cell Biol., 2021, 31, 994–1006 CrossRef CAS PubMed.
- P. Oberdoerffer, S. Michan, M. McVay, R. Mostoslavsky, J. Vann, S.-K. Park, A. Hartlerode, J. Stegmuller, A. Hafner, P. Loerch, S. M. Wright, K. D. Mills, A. Bonni, B. A. Yankner, R. Scully, T. A. Prolla, F. W. Alt and D. A. Sinclair, SIRT1 redistribution on chromatin promotes genomic stability but alters gene expression during aging, Cell, 2008, 135, 907–918 CrossRef CAS PubMed.
- D. Wahl, S. M. Solon-Biet, Q.-P. Wang, J. A. Wali, T. Pulpitel, X. Clark, D. Raubenheimer, A. M. Senior, D. A. Sinclair, G. J. Cooney, R. de Cabo, V. C. Cogger, S. J. Simpson and D. G. Le Couteur, Comparing the effects of low-protein and high-carbohydrate diets and caloric restriction on brain aging in mice, Cell Rep., 2018, 25, 2234–2243 CrossRef CAS PubMed .e2236.
- A. R. Heydari, A. Unnikrishnan, L. V. Lucente and A. Richardson, Caloric restriction and genomic stability, Nucleic Acids Res., 2007, 35, 7485–7496 CrossRef CAS PubMed.
- L. Bruens, S. I. J. Ellenbroek, S. J. E. Suijkerbuijk, M. Azkanaz, A. J. Hale, P. Toonen, D. J. Flanagan, O. J. Sansom, H. J. Snippert and J. van Rheenen, Calorie restriction increases the number of competing stem cells and decreases mutation retention in the intestine, Cell Rep., 2020, 32, 107937 CrossRef CAS PubMed.
- T. Bondar and R. Medzhitov, p53-mediated hematopoietic stem and progenitor cell competition, Cell Stem Cell, 2010, 6, 309–322 CrossRef CAS PubMed.
- D. Tang, S. Tao, Z. Chen, I. O. Koliesnik, P. G. Calmes, V. Hoerr, B. Han, N. Gebert, M. Zörnig, B. Löffler, Y. Morita and K. L. Rudolph, Dietary restriction improves repopulation but impairs lymphoid differentiation capacity of hematopoietic stem cells in early aging, J. Exp. Med., 2016, 213, 535–553 CrossRef CAS PubMed.
- C.-W. Cheng, G. B. Adams, L. Perin, M. Wei, X. Zhou, B. S. Lam, S. Da Sacco, M. Mirisola, D. I. Quinn, T. B. Dorff, J. J. Kopchick and V. D. Longo, Prolonged fasting reduces IGF-1/PKA to promote hematopoietic-stem-cell-based regeneration and reverse immunosuppression, Cell Stem Cell, 2014, 14, 810–823 CrossRef CAS PubMed.
- S. J. Mitchell, J. Madrigal-Matute, M. Scheibye-Knudsen, E. Fang, M. Aon, J. A. González-Reyes, S. Cortassa, S. Kaushik, M. Gonzalez-Freire, B. Patel, D. Wahl, A. Ali, M. Calvo-Rubio, M. I. Burón, V. Guiterrez, T. M. Ward, H. H. Palacios, H. Cai, D. W. Frederick, C. Hine, F. Broeskamp, L. Habering, J. Dawson, T. M. Beasley, J. Wan, Y. Ikeno, G. Hubbard, K. G. Becker, Y. Zhang, V. A. Bohr, D. L. Longo, P. Navas, L. Ferrucci, D. A. Sinclair, P. Cohen, J. M. Egan, J. R. Mitchell, J. A. Baur, D. B. Allison, R. M. Anson, J. M. Villalba, F. Madeo, A. M. Cuervo, K. J. Pearson, D. K. Ingram, M. Bernier and R. de Cabo, Effects of sex, strain, and energy intake on hallmarks of aging in mice, Cell Metab., 2016, 23, 1093–1112 CrossRef CAS PubMed.
- X. Guo, J. Ni, X. Liu, J. Xue and X. Wang,
Phyllanthus emblica L. fruit extract induces chromosomal instability and suppresses necrosis in human colon cancer cells, Int. J. Vitam. Nutr. Res., 2013, 83, 271–280 CrossRef CAS PubMed.
- X. Guo and X. Wang,
Phyllanthus emblica, fruit extract activates spindle assembly checkpoint, prevents mitotic aberrations and genomic instability in human colon epithelial NCM460 cells, Int. J. Mol. Sci., 2016, 17, 1437 CrossRef PubMed.
- X. Guo, H. Wang, J. Ni, Z. Liang, X. Wu, J. Xue and X. Wang, Geraniin selectively promotes cytostasis and apoptosis in human colorectal cancer cells by inducing catastrophic chromosomal instability, Mutagenesis, 2018, 33, 271–281 CrossRef CAS PubMed.
- X. Guo, X. Dai, J. Ni, X. Ma, J. Xue and X. Wang, Geraniin differentially modulates chromosome stability of colon cancer and noncancerous cells by oppositely regulating their spindle assembly checkpoint, Environ. Mol. Mutagen., 2019, 60, 254–268 CrossRef CAS PubMed.
- X. Guo, J. Ni, J. Xue and X. Wang,
Phyllanthus emblica Linn, fruit extract potentiates the anticancer efficacy
of mitomycin C and cisplatin and reduces their genotoxicity to normal cells in vitro, J. Zhejiang Univ., Sci., B, 2017, 18, 1031–1045 CrossRef CAS PubMed.
- L. Raffaghello, C. Lee, F. M. Safdie, M. Wei, F. Madia, G. Bianchi and V. D. Longo, Starvation-dependent differential stress resistance protects normal but not cancer cells against high-dose chemotherapy, Proc. Natl. Acad. Sci. U. S. A., 2008, 105, 8215–8220 CrossRef CAS PubMed.
- K. L. Tinkum, K. M. Stemler, L. S. White, A. J. Loza, S. Jeter-Jones, B. M. Michalski, C. Kuzmicki, R. Pless, T. S. Stappenbeck, D. Piwnica-Worms and H. Piwnica-Worms, Fasting protects mice from lethal DNA damage by promoting small intestinal epithelial stem cell survival, Proc. Natl. Acad. Sci. U. S. A., 2015, 112, E7148–E7154 CrossRef CAS PubMed.
- C. Lee, L. Raffaghello, S. Brandhorst, F. M. Safdie, G. Bianchi, A. Martin-Montalvo, V. Pistoia, M. Wei, S. Hwang, A. Merlino, L. Emionite, R. de Cabo and V. D. Longo, Fasting cycles retard growth of tumors and sensitize a range of cancer cell types to chemotherapy, Sci. Transl. Med., 2012, 4, 124ra127 Search PubMed.
- K. Xie, F. Neff, A. Markert, J. Rozman, J. A. Aguilar-Pimentel, O. V. Amarie, L. Becker, R. Brommage, L. Garrett, K. S. Henzel, S. M. Hölter, D. Janik, I. Lehmann, K. Moreth, B. L. Pearson, I. Racz, B. Rathkolb, D. P. Ryan, S. Schröder, I. Treise, R. Bekeredjian, D. H. Busch, J. Graw, G. Ehninger, M. Klingenspor, T. Klopstock, M. Ollert, M. Sandholzer, C. Schmidt-Weber, M. Weiergräber, E. Wolf, W. Wurst, A. Zimmer, V. Gailus-Durner, H. Fuchs, M. Hrabě de Angelis and D. Ehninger, Every-other-day feeding extends lifespan but fails to delay many symptoms of aging in mice, Nat. Commun., 2017, 8, 155 CrossRef PubMed.
- R. de Cabo, S. Fürer-Galbán, R. M. Anson, C. Gilman, M. Gorospe and M. A. Lane, An in vitro model of caloric restriction, Exp. Gerontol., 2003, 38, 631–639 CrossRef CAS PubMed.
- L. Dolz-Edo, M. van der Deen, S. Brul and G. J. Smits, Caloric restriction controls stationary phase survival through Protein Kinase A (PKA) and cytosolic pH, Aging Cell, 2019, 18, e12921 CrossRef PubMed.
- T. Lo, J. H. Ho, M.-H. Yang and O. K. Lee, Glucose reduction prevents replicative senescence and increases mitochondrial respiration in human mesenchymal stem cells, Cell Transplant., 2011, 20, 813–826 Search PubMed.
- J. Li, R. S. Settivari and M. J. LeBaron, Genetic instability of in vitro cell lines: Implications for genetic toxicity testing, Environ. Mol. Mutagen., 2019, 60, 559–562 CAS.
- S. V. Sharma, D. A. Haber and J. Settleman, Cell line-based platforms to evaluate the therapeutic efficacy of candidate anticancer agents, Nat. Rev. Cancer, 2010, 10, 241–253 CrossRef CAS PubMed.
- U. Ben-David, B. Siranosian, G. Ha, H. Tang, Y. Oren, K. Hinohara, C. A. Strathdee, J. Dempster, N. J. Lyons, R. Burns, A. Nag, G. Kugener, B. Cimini, P. Tsvetkov, Y. E. Maruvka, R. O'Rourke, A. Garrity, A. A. Tubelli, P. Bandopadhayay, A. Tsherniak, F. Vazquez, B. Wong, C. Birger, M. Ghandi, A. R. Thorner, J. A. Bittker, M. Meyerson, G. Getz, R. Beroukhim and T. R. Golub, Genetic and transcriptional evolution alters cancer cell line drug response, Nature, 2018, 560, 325–330 CrossRef CAS PubMed.
- J. R. Cantor, M. Abu-Remaileh, N. Kanarek, E. Freinkman, X. Gao, A. Louissaint, C. A. Lewis and D. M. Sabatini, Physiologic medium rewires cellular metabolism and reveals uric acid as an endogenous inhibitor of UMP synthase, Cell, 2017, 169, 258–272 CrossRef CAS PubMed.
- J. Vande Voorde, T. Ackermann, N. Pfetzer, D. Sumpton, G. Mackay, G. Kalna, C. Nixon, K. Blyth, E. Gottlieb and S. Tardito, Improving the metabolic fidelity of cancer models with a physiological cell culture medium, Sci. Adv., 2019, 5, eaau7314 CrossRef PubMed.
- J. Halliwell, I. Barbaric and P. W. Andrews, Acquired genetic changes in human pluripotent stem cells: origins and consequences, Nat. Rev. Mol. Cell Biol., 2020, 21, 715–728 CrossRef CAS PubMed.
- L. K. Heilbronn, L. de Jonge, M. I. Frisard, J. P. DeLany, D. E. Larson-Meyer, J. Rood, T. Nguyen, C. K. Martin, J. Volaufova, M. M. Most, F. L. Greenway, S. R. Smith, W. A. Deutsch, D. A. Williamson, E. Ravussin and F. T. Pennington Calerie Team, Effect of 6-month calorie restriction on biomarkers of longevity, metabolic adaptation, and oxidative stress in overweight individuals: A randomized controlled trial, J. Am. Med. Assoc., 2006, 295, 1539–1548 CrossRef CAS PubMed.
- E. E. Bankoglu, C. Arnold, I. Hering, M. Hankir, F. Seyfried and H. Stopper, Decreased chromosomal damage in lymphocytes of obese patients after bariatric surgery, Sci. Rep., 2018, 8, 11195 CrossRef PubMed.
- C. Y. Liao, B. A. Rikke, T. E. Johnson, V. Diaz and J. F. Nelson, Genetic variation in the murine lifespan response to dietary restriction: from life extension to life shortening, Aging Cell, 2010, 9, 92–95 CrossRef CAS PubMed.
- I. Kassam, Y. Wu, J. Yang, P. M. Visscher and A. F. McRae, Tissue-specific sex differences in human gene expression, Hum. Mol. Genet., 2019, 28, 2976–2986 CrossRef CAS PubMed.
Footnotes |
† Electronic supplementary information (ESI) available. See DOI: https://doi.org/10.1039/d2fo03138h |
‡ This paper is dedicated to the memory of the well-respected Prof. Jinglun Xue, who passed away suddenly on December 3, 2022. |
|
This journal is © The Royal Society of Chemistry 2023 |
Click here to see how this site uses Cookies. View our privacy policy here.