DOI:
10.1039/D2FO02927H
(Paper)
Food Funct., 2023,
14, 489-499
Immunomodulatory effects of extracellular glyceraldehyde 3-phosphate dehydrogenase of exopolysaccharide-producing Lactiplantibacillus plantarum JCM 1149†
Received
30th September 2022
, Accepted 12th December 2022
First published on 15th December 2022
Abstract
Probiotic lactic acid bacteria evoke immunomodulatory effects in the host; however, the reasons for the different effects of various species and strains remain to be elucidated. To clarify the critical immunomodulatory components and impact of exopolysaccharide (EPS) in Lactiplantibacillus plantarum, 11 types of L. plantarum strains were compared for the production of EPS, inflammatory cytokines, interleukin-6 and -12, and the anti-inflammatory cytokine, interleukin-10, from THP-1 differentiated dendritic cells. EPS in the fermented medium correlated with cytokine-inducing activities. L. plantarum JCM 1149, with the highest production of EPS, also induced interleukin-6, -10, and -12 among the 11 tested strains. Notably, the cytokine-producing activities overlapped with the protein fraction in gel filtration chromatography but not with EPS, which has been reported to exert immunomodulatory effects. The 41 kDa protein that coexisted with EPS was purified as a major active component and identified as glyceraldehyde 3-phosphate dehydrogenase (GAPDH), a known moonlighting protein. GAPDH secretion was reduced when EPS synthesis inhibitors were added to the culture medium. RNA sequencing of GAPDH-treated THP-1 cells revealed an up-regulation in the expression of genes involved in transcriptional regulation, cell surface receptor signalling, immune response, and matrix components. Here, we report, to our knowledge for the first time, that the cell surface-associated L. plantarum GAPDH plays a crucial role in cytokine production in THP-1 cells, but EPS with less activity may help GAPDH secretion.
1. Introduction
Lactic acid bacteria (LAB) play a pivotal role in food fermentation and production of functional food components with health benefits for humans and animals. Useful LAB have been isolated from traditional fermented food products and environmental niches, including plant-derived substrates and fruits,1 to apply for starter cultures in dairy products. Probiotics are often used for the control of gastrointestinal disorders, immunomodulation, maintenance of intestinal microbial balance, and prevention of pathogenic bacterial infections.2–5
Stimulation of the host immune function by lactobacilli is explained via interactions with the gut immune system.6 Previous studies have demonstrated various host immune responses via host–bacterial interactions involving conserved bacterial ligands and pattern recognition receptors (PRRs), such as Toll-like receptors (TLRs), on dendritic cells (DCs).7 Probiotic LAB are strong inducers of pro-inflammatory cytokine activities, such as interleukin (IL)-12 and tumour necrosis factor (TNF)-α in the gut.7 The immunomodulatory effects of lactobacilli are closely linked to their uptake into gut-associated lymphoid tissue and modulation of mucosal immune responses. Microbial-associated molecular patterns (MAMPs) also modulate multiple host immune systems. Lipopolysaccharides (LPSs), lipoproteins, peptidoglycans, polysaccharide A, lipoteichoic acid (LTA), and microbial RNA, DNA, and CpG motif have been reported as MAMP components.8 Crosstalk between the MAMPs in lactobacilli and host PRRs allows for different host immune responses9 and modulates the signalling of some pathways after interaction with TLR, nuclear factor-kappa B (NF-κB), and mitogen-activated protein kinase signalling pathways, in a strain-dependent manner, including strains of the same species.10 For instance, lipoproteins in Lactobacillus plantarum WCFS1 stimulated TLR2 signalling and inflammatory responses.11 TLR3-mediated inflammation with LTA was reported with L. plantarum strain.12 CpG oligodeoxynucleotides significantly increased the IL-12, TNF-α, and TLR9 and enhanced NF-kB signalling in LPS-stimulated cells.13 However, the species and strains that are dependent on these probiotic effects and the contributions of these effects remain unknown.
One of the most important bioactive compounds produced by lactobacilli is exopolysaccharide (EPS), a long-chain polysaccharide consisting of glucose, galactose, fructose, mannose, or other monosaccharides.14 The EPS produced by Lactiplantibacillus plantarum is the most extensively studied with focus on its biological function, structure, and genes.15–17 Previous studies on L. plantarum EPS have focused mainly on the relationship between its biological activities and structural characterization, and the roles of specific genes,14,17,18 whereas little is known about the contribution of EPS to the immunomodulatory activities of whole cell components. Four gene clusters of EPS-related genes involved in the regulation of EPS production, chain length determination, biosynthesis of repeating units, and polymerization and export of repeating units,19–22 have been described in L. plantarum WCFS1,23 a probiotic and food-associated strain.
The immunomodulatory effects of EPS have been reported in various types of lactobacilli with or without EPS production. Therefore, in the present study, we attempted to evaluate the importance of EPS in immunomodulatory effects using various types of L. plantarum strains with different EPS production capacities and attempted to identify a key component that acts on cytokine production in THP-1 DCs. We also attempted to clarify the mechanism of action of the purified component in THP-1 DCs to understand its immunomodulatory activity.
2. Materials and methods
2.1 Regents and bacterial strains
Casein, bovine serum albumin, ovalbumin, and lysozyme (LTM) were purchased from Wako (Osaka, Japan). Tris (tris(hydroxymethyl)aminomethane) and trichloroacetic acid (TCA) were purchased from Nacalai Tesque Inc. (Kyoto, Japan). Dulbecco's modified Eagle's medium and penicillin–streptomycin (10
000 U mL−1) were obtained from Thermo Fisher Scientific (JP, Tokyo, JAPAN). Fetal bovine serum (FBS) was obtained from Biowest (MO, USA). Trypsin was obtained from Promega (Madison, WI, USA). All JCM LAB strains listed in Table 1 were obtained from the Japan Collection of Microorganisms (JCM). Other LAB strains were obtained from National Agriculture and Food Research Organization (NARO). The 1× phosphate-buffered saline (PBS) used in this study consisted of 137 mM of NaCl, 10 mM of Na2HPO4, 2.7 mM of KCl, and 1.8 mM of KH2PO4, and the pH was adjusted to 7.2 with HCl.
Table 1 Llist of Lactiplantibacillus plantarum strains used in this study
No. |
Strain |
Origin |
JCM: Japan Collection of Microorganisms. NARO: National Agriculture and Food Research Organization. |
1 |
Lactiplantibacillus platarum JCM 1149 |
JCM |
2 |
Lactiplantibacillus platarum JCM 1100 |
JCM |
3 |
Lactiplantibacillus platarum A73 |
NARO |
4 |
Lactiplantibacillus platarum D64 |
NARO |
5 |
Lactiplantibacillus platarum J21 |
NARO |
6 |
Lactiplantibacillus platarum OKI-51 |
NARO |
7 |
Lactiplantibacillus platarum OKI-58 |
NARO |
8 |
Lactiplantibacillus platarum OKI-59 |
NARO |
9 |
Lactiplantibacillus platarum 8-41 |
NARO |
10 |
Lactiplantibacillus platarum 12-38 |
NARO |
11 |
Lactiplantibacillus platarum 12-71 |
NARO |
2.2 Quantification of EPS and capsular polysaccharide (CPS) in the cultured medium
To evaluate the content of EPS in various LAB culture media, pre-existing EPS in MRS medium (Difco) with a molecular weight of over 10 kDa was removed by filtration through an Amicon Ultra-15 Centrifugal Filter Unit (Merck & Co., Inc., USA) (MRS-F). Then, each LAB strain was cultured in MRS-F medium for 20 h at 30 °C. The cells in the culture medium were removed via centrifugation at 10
000g for 5 min. Then, protein in the supernatant was precipitated by adding of 10% volume of TCA. Next, the supernatant was mixed with 10 times 100% EtOH and EPS was precipitated via centrifugation at 12
000g for 20 min at 4 °C, according to a previous method.24 The precipitate was dissolved in 1 mL of MilliQ water (EPS solution) and quantified to assess its immunomodulatory activity. Then, 100 μL of the EPS and solutions were mixed with 100 μL of 5% (v/v) phenol solution and vortexed, and 500 μL of sulfuric acid (v/v) was added. After incubation for 10 min at 25 °C, 100 μL of the mixture was transferred into each well of a microplate, and the absorbance at 490 nm was measured using an iMark Microplate Absorbance Reader (Bio-Rad Laboratories, Inc., USA). Glucose was used to prepare a standard curve. CPS, which is covalently combined with the bacterial cell wall, was extracted according to a previously described method.25 Briefly, bacterial cells were collected after fermentation in MRS-F medium via centrifugation at 5000g for 5 min at 4 °C. Then, bacterial cells were suspended in 0.2× PBS and treated with an ultrasonic homogenizer (Nikkyo Technos, Co., Ltd; HOM-100) for 30 s on ice. The supernatant was collected after centrifugation at 8000g for 5 min at 4 °C (CPS solution). To evaluate evalueate enough disruption of cell wall, protein concentration of the supernatants were monitored after several times treatments of the ultrasonic homogenizations. To inhibit EPS synthesis in L. plantarum JCM 1149, 0.15% of 2-(4-methoxyphenyl)ethylamine (Tokyo Chemical Industry, Tokyo, Japan), which was suggested to have an inhibitory effect on glycosylation by Ren et al.,26 was added to the MRS-F medium and incubated at 30 °C for 1.5 h.
2.3 Preparation of THP-1 DC and cytokine measurement
THP-1 cells were cultured in the Roswell Park Memorial Institute 1640 medium supplemented with 10% FBS, 100 U mL−1 penicillin, and 100 μg mL−1 streptomycin. For differentiation, the cells were incubated with 100 nM phorbol 2-myristate 13-acetate (Adipogen Life Science, Liestal, Switzerland) for three days in a 96-well culture plate at 5.0 × 104 cells per well (THP-1 DCs). Subsequently, EPS solution (see section 2.2) collected from MRS-F fermented medium was added to THP-1 DCs at 10% (v/v). Samples collected from gel filtration were dialysed with PBS before incubation with THP-1 DC. Equal volumes of MilliQ water or PBS were incubated with THP-1 DC as negative controls for the EPS solution and gel-filtrated samples, respectively. Subsequently, 100 ng mL−1 of IL-4 or IFN-γ was added for IL-10 or IL-6 and IL-12 p40 production, respectively. After incubation at 37 °C under 5% CO2 for 24 h, the supernatant was collected, and cytokines in the supernatant were measured using an enzyme-linked immunosorbent assay (ELISA) kit. IL-6 and IL-10 ELISA kits were obtained from BioLegend Inc. (San Diego, CA, USA), and IL-12 p40 ELISA kit was obtained from R&D Systems (Minneapolis, MN, USA). Fluorescence was measured in triplicate using a plate reader (Varioskan LUX SkanIt Software 4.0; Thermo Fisher Scientific). If the cytokine production in the supernatant was low, 20% (v/v) of the sample was added to THP-1 DC. For receptor analysis, anti-TLR2 (Sino Biological Inc., Peking, China) or anti-TLR4 (Sino Biological Inc., Peking, China) antibodies were pre-incubated with THP-1 DCs at 25 °C for 1 h after 1000 times dilution. Next, 0.1 μg mg−1 of glyceraldehyde 3-phosphate dehydrogenase (GAPDH) was added to THP-1 DCs because GAPDH showed dose dependent cytokine inductions for IL-6 and IL-10 at 0.01–0.1 μg mL−1, and 0.1 μg mL−1 was considered to be appropriate to detect the inhibitory effect of the antibody. IL-6 or IL-10 in the culture supernatant was measured after incubation at 37 °C for 24 h.
2.4 Gel filtration of the culture supernatant
To evaluate the immunomodulatory effect of EPS, 1 ml of supernatant of L. plantarum JCM 1149 fermented in MRS-F medium (containing 1.0 mg mL−1 of EPS) were fractionated using a gel filtration column (Φ 1.5 cm × 50 cm) containing Bio-gel P-100 and eluted with 10 mM phosphate and 135 mM NaCl (pH 7.0). About 1.4 mL of eluent was fractionated in each microtube. EPS and protein concentrations in each fraction were assessed. EPS was quantified according to the method described in section 2.2, and protein was quantified using the Coomassie Brilliant Blue (CBB) assay kit (5×) (Nakalai, Kyoto, Japan), according to the method described in the protocol. The fractions were then incubated with THP-1 DCs to measure IL-6, IL-10, and IL-12 levels.
2.5 Purification of the immunomodulatory component
L. plantarum JCM 1149 was cultured in MRS-F medium for 30 h at 30 °C, and the cells were harvested via centrifugation at 5000g for 5 min and then washed with 0.2× PBS. The cells were suspended in 0.5 M LiCl and incubated on ice for 15 min, and the supernatant was collected via centrifugation at 5000g for 5 min. Surface layer proteins (Slps) were concentrated, LiCl was removed using an Amicon Ultra-4 Centrifugal Filter Unit, and applied to a gel filtration column, as described in section 2.4. These fractions were assessed for their effects on IL-10, IL-6, and IL-12 production. The concentrations of EPS and protein were measured using the method described in section 2.4. Proteins in each fraction were analysed using sodium dodecyl sulfate-polyacrylamide gel electrophoresis (SDS-PAGE). Active fractions showing cytokine-inducing activity and containing major components were pooled and used as purified GAPDH.
2.6 SDS-PAGE and protein identification
Proteins in each fraction from the gel filtration were analyzed using 12.5% SDS-PAGE. The proteins were mixed with sample buffer (×6: 125 nM Tris-HCl, 4% SDS, 20% glycerol, 0.012% bromophenol blue, and 10% 2-mercaptoethanol), and heated for 5 min at 95 °C, and analyzed via SDS-PAGE, according to the method of Laemmli.27 Protein bands in the gel were visualized by staining the gels with CBB. Protein Ladder One Plus (Nacalai Tesque, Kyoto, Japan) was used as a size marker. Proteome analysis was performed to identify the proteins. Protein bands were excised from the SDS-PAGE gel after CBB staining. To remove the dye from the gel, de-staining solution (30% acetonitrile, 50 mM NH4HCO3) was added and incubated for 30 min. Then, 60% acetonitrile and 20 mM NH4HCO3 were added to remove the water from the gel. Next, 5% (w/w) of trypsin (Promega Japan, Tokyo) was added to the dried gel and incubated at 37 °C for 12 h. The peptides released from the gel were analyzed via mass spectrometry using UltrafleXtreme TOF/TOF MS (Bruker Daltonics GmbH, Bremen, Germany) operating in positive reflection ion mode between m/z 0 and 5000 Da. The proteins in the gel were transferred to a polyvinylidene fluoride membrane (Wako Pure Chemical Industries, Ltd, Japan) in Tris-glycine buffer at pH 8.3 (190 mM glycine, 5 mM Tris-Cl, and 20% methanol) for 1 h at 200 mA. Proteins were detected using an anti-GAPDH mouse antibody (Gene Tex, Inc., USA).
2.7 Interactions among EPS, GAPDH, and other components
To purify EPS, EPS was extracted from JCM 1149 after cultivation in MRS-F, according to the method described in section 2.2. Ribonuclease (Nippongene, Tokyo, Japan) (10 μg mL−1) and 12 units of DNase (NIPPON GENE. Co., Ltd, Tokyo, Japan) were added and the mixture was incubated for 30 min at 37 °C. Proteinase K (Merck & Co., Inc., USA) was added at 0.2 unit per mL and incubated at 55 °C for 30 h. EPS solution was dialyzed against distilled water using Visking Tubing (As One. Co., Ltd, Osaka, Japan). GAPDH purified by a gel filtration column, as described in section 2.5 was labeled with a Cy3-labeling kit (Amersham CyTM3 Mono-Reactive Dye Pack, GE Healthcare. Co., USA). To understand the interaction between GAPDH and EPS, 1 μg of Cy3GAPDH was used to analyze the molecular weight with or without pre-incubation with EPS at 25 °C for 30 min via gel filtration containing Bio gel P-100, according to the method described in 2.4. Samples were collected in microtubes, as described in section 2.4. GAPDH was monitored by measuring Cy3 originated fluorescence at 550 nm as the excitation wavelength and 522 nm as the emission wavelength. EPS was measured according to the method described in section 2.2.
2.8 Gene expression analysis of THP-1 DCs
THP-1 cells were seeded at 5.0 × 104 cells per well in 12 wells of a 96-well culture plate and differentiated as described in section 2.3. Purified GAPDH (10 ng) or PBS was added to each well of THP-1 DCs (1
:
4) and incubated for 5 h at 37 °C under 5% CO2. The cells were washed with PBS and total RNA was extracted using the Rneasy Mini Kit (QIAGEN, Hilden, Germany). After evaluating the quality and quantity of RNA using NanoDrop 2000 (Thermo Fisher Scientific, MA, USA), equal amounts of RNA samples extracted from triplicate samples from each group were mixed. Then, RNA sequencing was performed for two mixed groups from triplicated samples, GAPDH-treated and GAPDH untreated groups. THP-1 DC RNA sequencing was performed at the Bioengineering Lab (Kanagawa, Japan). A cDNA library was constructed using the MGIEasy RNA Directional Library Prep Set (MGI Tech Co., Ltd, Tokyo, Japan). The library preparations were sequenced on a DNBSEQ-G400 (MGI Tech Co., Ltd) by the Bioengineering Lab (Kanagawa, Japan). Differential expression analysis was performed using DESeq (ver.1.18.0). Genes with an adjusted (fold-change) >1.3, or (fold change) <−1.3 were selected for network analysis. Molecular interactions between the up-regulated and down-regulated genes were visualized using STRING (https://string-db.org/). Immune function-related genes with networks were selected and specific primers were prepared to quantify the gene expression in triplicate samples, as listed in Table S1.† RNA was reverse-transcribed (RT) into complementary DNA (cDNA) using quantitative polymerase chain reaction (qPCR) RT master mix, according to the manufacturer's instructions. The resulting cDNA was diluted 5-fold with nuclease-free water and mixed with specific primers (Table S1†) and the SYBR qPCR mix. The mixtures were incubated in a StepOne Real-Time PCR system (Applied Biosystems, MA, USA), according to the manufacturer's instructions. The data obtained were normalized to the mRNA expression level of β-actin, a housekeeping gene.
2.9 Statistical analysis
Statistical significance was analyzed by using One-way or Two-way ANOVA with GraphPad Prism software package version 9.1. Statistical significance was set at P < 0.05.
3. Results
3.1 EPS productions in various L. plantarum strains
To quantify EPS production in L. plantarum fermented medium, pre-existing polysaccharides in MRS medium with molecular mass over 10 kDa were filtered (MRS-F), as described in Materials and Methods. MRS-F medium, showing a significant reduction in EPS, was used to compare EPS production by various L. plantarum strains. Eleven types of L. plantarum strains listed in Table 1 were cultured in MRS-F at 30 °C for 20 h, and the EPS in the supernatants was analyzed according to the method described in Materials and Methods (n = 3). JCM 1149 showed the highest production of EPS (170 mg L−1) among the measured 11 strains, whereas the other types of L. plantarum strains showed relatively lower production of EPS (Fig. 1).
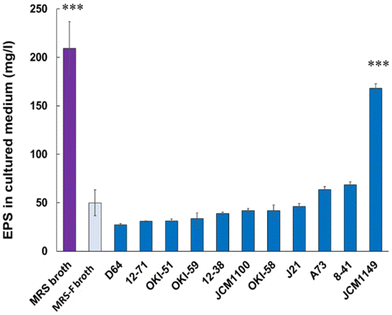 |
| Fig. 1 EPSs in MRS and MRS-F media, and MRS-F cultured with various L. actiplantibacillus plantarum strains. Data are represented as mean ± SD (n = 3). *p < 0.05 and ***p < 0.001 show significant difference from the control (MRS-F broth) analyzed by One-way ANOVA. | |
3.2. Cytokine production in various L. plantarum-fermented supernatants
To confirm the reported cytokine-inducing activities of EPS, various cytokine productions, pro-inflammatory cytokines, such as IL-12 and IL-6, and anti-inflammatory cytokine, IL-10, were measured for MRS-F fermented with 11 L. plantarum strains. As expected, among the 11 tested strains, JCM 1149, which had the highest EPS production, showed the highest production of IL-6, IL-10, and IL-12 (Fig. 2). Moreover, the cytokine-producing activities of various L. plantarum-fermented MRS-Fs were almost correlated with the production of EPS (Fig. 2). Therefore, JCM 1149, which showed the highest production of IL-6, IL-10, and IL-12, was selected for further analysis.
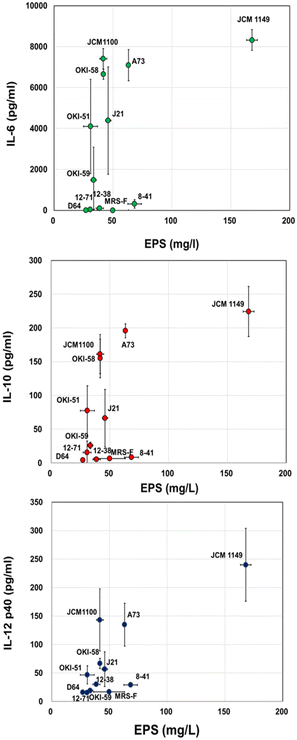 |
| Fig. 2 EPS in MRS-F cultured with various L. plantarum strains and cytokine-producing activity, IL-6, IL-10, and IL-12 of the cultured medium. Data are represented as mean ± SD (n = 3). | |
3.3. Gel filtration of JCM 1149 fermented MRS-F
To identify the active component in JCM 1149 fermented MRS-F, the supernatant was applied to a gel filtration column (1.4 cm Φ × 48 cm) containing Bio-gel P100. EPS quantified by the method of Nagai24 was mainly observed at 17–22 mL after elution (Fig. 3A) and surprisingly did not overlap with cytokine-producing activities. In contrast, proteins eluted at 15–17 mL almost overlapped with cytokine-producing activities, such as IL-6, IL-10, and IL-12 (Fig. 3B).
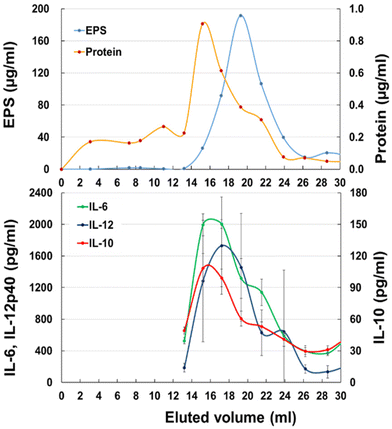 |
| Fig. 3 Gel chromatography of JCM 1149 cultured MRS-F on a Bio-Gel P-100 column (Φ 1.5 cm × 50 cm). (A) EPS and protein, (B) IL-6, IL-10 and IL-12 producing activities (n = 3, mean ± SD). | |
3.4. Gel filtration of JCM 1149 Slps
Most of the cytokine-producing activity in the fractions eluted from gel filtration overlapped with the protein fractions but contained a small amount of EPS (Fig. 3A). Therefore, to remove EPS from the active fraction, the surface layer proteins (Slps) collected from the JCM 1149 cell surface by washing with LiCl were fractionated by gel filtration. As expected, IL-6 producing activity was observed at the elution from 14–16 mL whereas no EPS was observed in any fraction (Fig. 4). The 41 kDa protein mainly observed in the active fractions, was correlated with IL-6 production. The 41 kDa protein purified by gel filtration was considered as the active component, and was identified as glyceraldehyde 3-phosphate dehydrogenase (GAPDH), a member of the moonlighting protein family, by proteome analysis after trypsin digestion. GAPDH in Fr. 14 and 15 were combined to yield purified GAPDH.
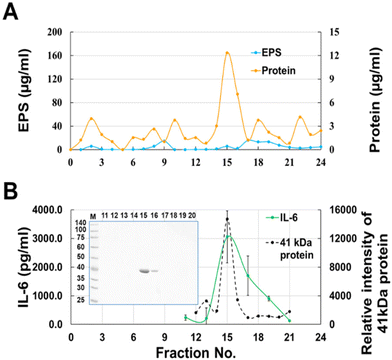 |
| Fig. 4 Gel chromatography of Slps released with 0.5 M LiCl from JCM 1149 on a Bio-Gel P-100 column (Φ 1.5 cm × 50 cm). (A) EPS and protein, (B) IL-6-producing activity (n = 3, mean ± SD) and GAPDH; 12.5% sodium dodecyl sulfate-polyacrylamide gel electrophoresis (SDS-PAGE) of proteins in each fraction (n = 1). | |
3.5 GAPDH interaction with EPS and secretion
To understand why the JCM 1149 strain with higher EPS produced higher amounts of extracellular GAPDH, we attempted to inhibit EPS synthesis in JCM 1149 by adding of 1.5% of 2-(4-methoxyphenyl) ethylamine (MPEA),25 an EPS synthesis inhibitor. If MPEA in the culture medium added was over 2%, the growth of JCM 1149 was completely inhibited (data not shown). However, JCM 1149 showed an increase in growth in 1.5% MPEA-supplemented medium (83% of growth) (Fig. 5). Under these conditions, EPS and CPS production were significantly reduced (68% and 74%, respectively) (Fig. 5). Notably, GAPDH on the cell surface of JCM 1149 was significantly reduced by 1.5% MPEA (Fig. 5, Fig. S1†).
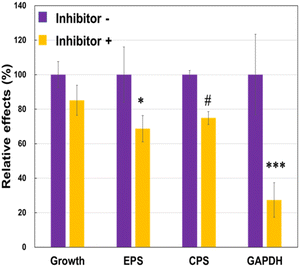 |
| Fig. 5 Cell growth and EPS, CPS and glyceraldehyde 3-phosphate dehydrogenase (GAPDH) production in L. plantarum JCM 1149 with or without EPS synthesis inhibitor. Data are represented as mean ± SD (n = 3). #p < 0.1, *p < 0.05 and ***p < 0.001 show significant difference between inhibitor − and + analyzed by Two-way ANOVA. | |
Next, the interaction of GAPDH with EPS was evaluated using gel filtration column chromatography. Briefly, the retention time of Cy3GAPDH was compared to that of Cy3GAPDH after pre-incubation with EPS. The purified Cy3GAPDH and EPS had molecular weights (MW) of approximately 50 kDa and 40 kDa, respectively (Fig. 6). However, Cy3 signals originating from GAPDH were observed in various fractions (130, 90, 65, and 50 kDa) when EPS was pre-incubated, suggesting an interaction between Cy3GAPDH and EPS (Fig. 6).
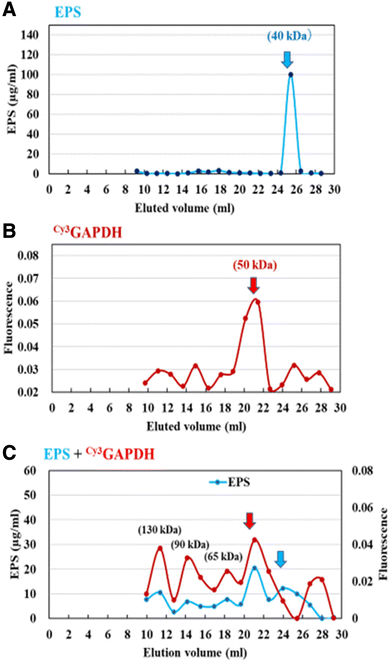 |
| Fig. 6 Gel chromatography of the purified EPS (A), Cy3GAPDH (B), and the mixture (C) on a Bio-Gel P-100 column (Φ 1.5 cm × 50 cm). | |
To confirm the presence of extracellular GAPDH in other L. plantarum strains, the expression of GAPDH in JCM 1149, D64, and A73 was detected using western blotting with an anti-GAPDH antibody. Higher production of GAPDH was observed in JCM 1149 than in the other L. plantarum strains, A73 and D64 (Fig. 7, S2†).
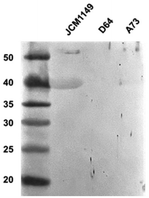 |
| Fig. 7 Western blotting analyses of Slps in L. plantarum JCM 1149, D64 and A73. | |
3.6 Differentially expressed genes in THP-1 DCs after GAPDH treatment
JCM 1149, which had the highest EPS, significantly induced all tested cytokines, IL-6, IL-10, and IL-12, in THP-1 DCs (Fig. 2). Furthermore, GAPDH was considered to contribute to the activation of the three types of cytokine production in THP-1 DCs. Hence, to understand the mechanism of action underlying the immunomodulatory effect of EPS production in JCM 1149, we performed RNA sequencing of THP-1 DCs to determine the differences in gene expression between GAPDH and PBS (control). The expression profiles of THP-1 DCs treated with GAPDH and the control groups were compared to screen for differentially expressed genes (DEGs). As a result, 315 genes in the GAPDH group were found to be upregulated (Table S2†) and 789 genes were downregulated (Table S3†), compared to those in the control group, when DEGs were selected using the criteria −1.3 < log fold change < 1.3, P < 0.05.
Molecular interactions among these genes were visualized using STRING (https://string-db.org/) (Fig. S3†). The possible interacting genes suggested to be involved in immune function are summarized in Fig. 8. The interaction of GAPDH with TLR2 on THP-DC may induce some transcriptional regulators, such as NFKB1, NFKB2, REL, RELB, and JUNB, receptors, such as TLR1, TLR2, TLR4, CD40 and CD44, signaling pathways, such as IRAK; immune responses, such as TNFAIP3, TNFAIP2, and IL1B responses; and cell matrices, such as ITGA2, ITGA4, ICAM, and VCAM (Fig. 8).
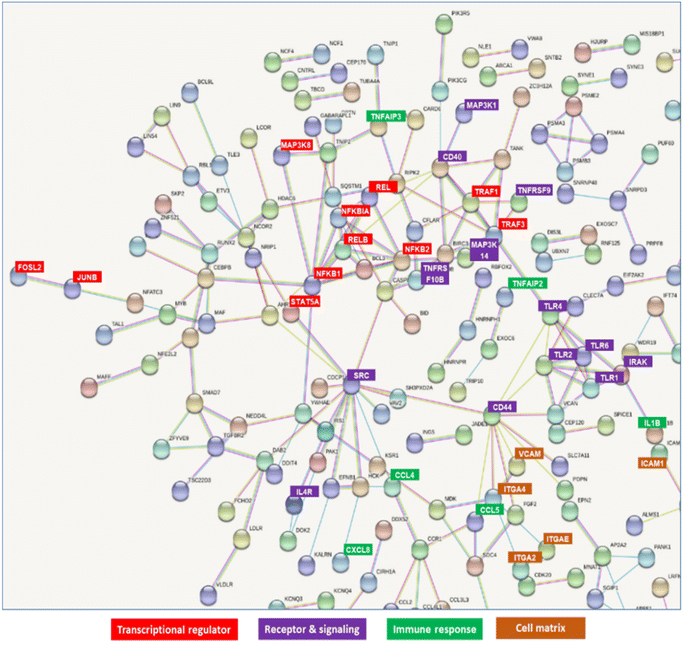 |
| Fig. 8 Network detected in upregulated and downregulated genes in THP-1 dendritic cells (DCs) after treatment with GAPDH. | |
Various genes involved in immunomodulation were selected, and gene expression was quantified by RT-PCR using specific primer sets (Table S1†). Among the selected genes, the levels of NFKBIA, CD40, IL10, IL1B, and CXCL1 were significantly upregulated after incubation with GAPDH (Fig. 9).
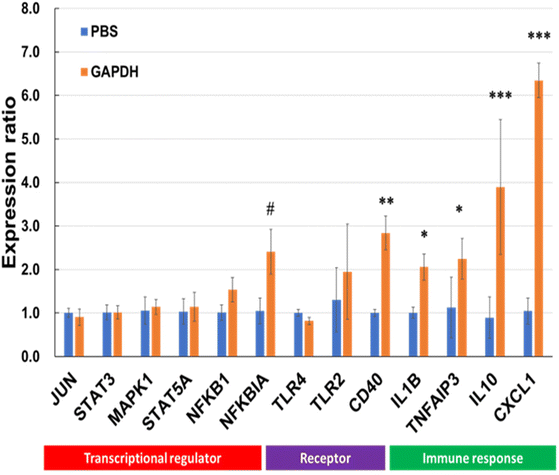 |
| Fig. 9 Reverse transcription-polymerase chain reaction (RT-PCR) for genes linked to transcriptional regulators, receptors and signals, and immune response in THP-1 DCs after treatment with GAPDH and phosphate-buffered saline (PBS; control). Data are represented as mean ± SD (n = 3 per group). Significant differences from control group analyzed by Two-way ANNOVA; #p < 0.1, *p < 0.05, **p < 0.01, ***p < 0.001. | |
3.7 Receptor for GAPDH on THP-1 DCs
Next, we evaluated whether anti-TLR2 or anti-TLR4 antibodies could prevent GAPDH from accessing these receptors. Anti-TLR2 antibody significantly reduced the induction of IL-6, and anti-TLR4 showed a decreasing trend (P = 0.095) (Fig. 10). However, neither antibody reduced IL-10 production. This result suggests that GAPDH may bind TLR2 to THP-1 DCs and induce IL-6 and IL-10 production.
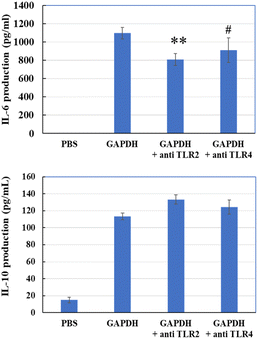 |
| Fig. 10 IL-6 and IL-10 production in THP-1 DCs via addition of GAPDH with or without anti-TLR2 or anti-TLR4 antibody. Significant differences from control group (GAPDH); #p < 0.1, **p < 0.01 (n = 3). | |
4. Discussion
4.1 Major immunomodulatory component in JCM 1149
Many previous studies have suggested the induction of cytokines in dendritic cells by EPS, especially by L. plantarum, which has four sets of CPS-related gene clusters, including EPS gene clusters. The complexity of the EPS structure is further exemplified by the observation that one bacterial genome possesses multiple polysaccharide biosynthesis-encoding gene clusters. For example, the L. plantarum WCFS1 genome encodes four CPS biosynthesis gene clusters.28,29 Therefore, in the present study, 11 types of L. plantarum strains were prepared to compare EPS production and IL-6, IL-10, and IL-12 inducing activities. The present study suggests that the EPS produced by L. plantarum is closely linked to the production of cytokines. Surprisingly, cytokine-inducing activity overlapped with extracellular GAPDH, but not with EPS. The immunomodulatory effect of WCSF1 derivatives was reduced if EPS cluster genes were lacking, suggesting reduced cytokine production with reduced EPS strains.18,30 The reason for the previous report showing cytokine-producing activity of EPS is not clear. However, in the present study, cytokine-producing activity of GAPDH in the culture medium was strongly suggested because cell surface GAPDH in JCM 1149 was reduced by the addition of the EPS synthesis inhibitor (Fig. 6), and GAPDH in other strains evaluated with specific antibodies was also correlated with cytokine production (Fig. 2 and 7). Moreover, from gel filtration column chromatography of JCM 1149 culture supernatant (Fig. 3), cytokine-producing activity based on protein (GAPDH was the primary protein in Fig. 4B) was estimated to be over 70%, as shown in Fig. S4.† Therefore, extracellular GAPDH, behind EPS, is considered a major active component in L. plantarum. Thus, extracellular GAPDH secreted by the EPS-producing L. plantarum strain plays a crucial role in cytokine production in THP-1 cells.
4.2 Immunomodulatory effects of GAPDH
GAPDH is known as a key enzyme in the glycolytic pathway but is also considered a multifunctional protein that interacts with intracellular and extracellular proteins (reviewed by Sirover31). GAPDH localized on the cell surface of Mycoplasma genitalium interacted with mucin, and this binding was significantly inhibited by the addition of a specific antibody.32 In E. coli, GAPDH interacts with metabolic enzymes and proteins involved in transcription or protein synthesis.33 In our study, GAPDH on the cell surface of Lactobacillus johnsonii interacted with tight junctions associated with JAM-2, and enhanced the integrity of matrix proteins in Caco-2 cells.34 GAPDH can generate NADH in the glycolytic pathway and promote the enhancement of IL-10 production and reduction of TNF-α in LPS induced macrophage.35 Pretreatment with recombinant GAPDH protected against Streptococcus pneumoniae infection in mice.36 Higher production of IL-12p70, IL-6, and TNF-α was observed after treatment with GAPDH but was reduced in TLR2-/- and TLR4-/- mice, indicating that GAPDH can interact with ligands for TLR2 but not with TLR4. GAPDH induced IL-1β, IL-6, TNF-α, and IL-10
37 expression The present study indicated a clear immunomodulatory effect of GAPDH secreted from L. plantarum JCM 1149 on the THP-1 DC-initiated interaction with TLR2.
4.3 Predicted signaling pathway
In the present study, GAPDH purified from L. plantarum JCM 1149 significantly induced IL-6, Il-10 and IL-12 production in THP-1 DCs (Fig. 2). Moreover, RNA sequencing of THP-1 DCs treated with GAPDH suggested that enhanced gene expression was strongly associated with transcriptional regulation, immunomodulation, cell response, and matrix components (Fig. 8). Furthermore, the major genes linked to immune function were quantified by RT-PCR (Fig. 9). Cytokine production was reduced by adding of an anti-TLR-2 antibody (Fig. 6). Taken together, these results suggest that possible host responses initiated by GAPDH-TLR-2 interactions were predicted and are summarized in Fig. 10. It has been suggested that GAPDH binding to TLR2 might activate various transcriptional regulators, TRAF, MARK, NFKB, JUNB, FOS, and RelB. Moreover, upregulated expression of both surface receptors, IL1R and IRAK, suggested cross signaling with upregulation of the TRAF-MARK pathway (Fig. 10). These transcriptional regulators activated the production of specific cytokines such as IL-1, IL-10, and TNF-α, and transmembrane receptors, such as CD40, CD44 and CD83 were upregulated during interactions with helper T cells. TLR-2 stimulated immune responses have been already reviewed.38
4.4 Secretion of GAPDH onto the cell surface
GAPDH, which is involved in glycolysis, functions as a moonlighting protein.39 Although GAPDH has no typical signature sequences for the secretion and anchoring feature at the carboxyl terminus to interact with the cell surface, it has been detected on the cell surface of various Gram-positive bacteria, including lactobacilli.40–43 The localization of GAPDH on the cell surface allows it to interact with some components of the host organism in the gut. For instance, GAPDH associated with the cell surface can adhere to gastric mucin in Caco-2 cells.34,44,45 However, its secretion is considered to depend on the species and strain, and the mechanism has not yet been elucidated. In L. plantarum 229, growth phase-dependent GAPDH secretion and localization on the cell surface due to altered plasma membrane permeability have been reported.46 The potential effect of GAPDH on the bacterial cell surface, which is closely linked to membrane permeability, was suggested in this study. In the present study, GAPDH interacted with EPS and CPS on the cell surface, but not with the CPS negative strain. Moreover, not only EPS, but also GAPDH in JCM 1149 was significantly reduced by the addition of the inhibitor, MPEA, during fermentation (Fig. 6), suggesting that other components interacting with GAPDH may have potential to secrete it. This strongly suggests that EPS synthesis may be a crucial event for the release of intracellular GAPDH on the cell surface and in the culture medium.
5. Conclusions
JCM 1149, with the highest EPS production, showed the highest induction of IL-6, IL-10, and IL-12 among the tested 11 strains of L. plantarum. However, cytokine-producing activity was mainly contributed by the moonlighting protein, GAPDH, which existed behind EPS in JCM 1149. GAPDH interacted with EPS and was significantly repressed by the inhibition of EPS synthesis. GAPDH could upregulate the immunomodulation-linked gene expression involving transcriptional regulators, surface receptors, signaling pathways, and cytokine production. These results may aid in understanding the roles of extracellular GAPDH in the host as well as the mechanism of its secretion in specific strains.
Author contributions
H. K., K. M., and N. Y. conceived this study and designed the experiments. H. K. performed the experiments and analyzed the data. H. K., K. M., and N. Y. wrote the manuscript.
Abbreviations
LAB | Lactic acid bacteria |
EPS | Exopolysaccharide |
CPS | Capsular polysaccharide |
DCs | Dendritic cells |
Slps | Surface layer proteins |
GAPDH | Glyceraldehyde 3-phosphate dehydrogenase |
IL | Interleukin |
TNF-α | Tumor necrosis factor |
Conflicts of interest
There are no conflicts of interest to declare.
Acknowledgements
We would like to thank Dr Hiromi Kimoto-Nira (Food Research Institute, NARO) and Dr Naoko Moriya (NARO Institute of Livestock and Grassland Science) for providing the stock culture of Lactiplantibacillus plantarum used in this study.
References
- R. J. Siezen and V. E. Evan Hylckama Vlieg, Genomic diversity and versatility of Lactobacillus plantarum, a natural metabolic engineer, Microb. Cell Fact., 2011, 10, S1 CrossRef PubMed.
- A. Pärtty, S. Rautava and M. Kalliomäki, Probiotics on pediatric functional gastrointestinal disorders, Nutrients, 2018, 9, 1836 CrossRef PubMed.
- M. Schultz and R. B. Sartor, Probiotics and inflammatory bowel diseases, Am. J. Gastroenterol., 2000, 95, S19 CrossRef CAS PubMed.
- S. Salminen, E. Isolauri and T. Onnela, Gut flora in normal and disordered states, Chemotherapy, 1995, 41, 5 CrossRef PubMed.
- G. T. Macfarlane and J. H. Cummings, Probiotics, infection and immunity, Curr. Opin. Infect. Dis., 2002, 15, 501 CrossRef CAS PubMed.
- A. Llewellyn and A. Foey, Probiotic modulation of innate cell pathogen sensing and signaling events, Nutrients, 2017, 9, 1156 CrossRef PubMed.
- M. Rescigno, A. Y. Huang and R. N. Germain, Dynamic imaging of dendritic cell extension into the small bowel lumen in response to epithelial cell TLR engagement, J. Exp. Med., 2006, 203, 2841 CrossRef PubMed.
- K. Atarashi, T. Tanoue, T. Shima, A. Imaoka, T. Kuwahara, Y. Momose, G. Cheng, S. Yamasaki, T. Saito, Y. Ohba, T. Taniguchi, K. Takeda, S. Hori, I. I. Ivanov, Y. Umesaki, K. Itoh and K. Honda, Induction of colonic regulatory T cells by indigenous Clostridium species, Science, 2011, 331, 337 CrossRef CAS PubMed.
- B. C. Offor, I. A. Dubery and L. A. Piater, Prospects of gene knockouts in the functional study of MAMP-triggered immunity: a review, Int. J. Mol. Sci., 2020, 21, 2540 CrossRef CAS PubMed.
- C. M. Thomas and J. Versalovic, Probiotics-host communication: modulation of signaling pathways in the intestine, Gut Microbes, 2010, 1, 148 CrossRef PubMed.
- I.-C. Lee, I. I. van Swam, S. Boeren, J. Vervoort, M. Meijerink, N. Taverne, M. Starrenburg, P. A. Bron and M. Kleerebezem, Lipoproteins contribute to the anti-inflammatory capacity of Lactobacillus plantarum WCFS1, Front. Microbiol., 2020, 29, 1822 CrossRef PubMed.
- H. Mizuno, L. Arce, K. Tomotsune, L. Albarracin, R. Funabashi, D. Vera, M. A. Islam, M. G. Vizoso-Pinto, H. Takahashi, Y. Sasaki, H. Kitazawa and J. Villena, Lipoteichoic acid is involved in the ability of the immunobiotic strain Lactobacillus plantarum CRL1506 to modulate the intestinal antiviral innate immunity triggered by TLR3 activation, J. Front. Immunol., 2020, 11, 571 CrossRef CAS PubMed.
- X. K. Gao, C. Wang, L. Liu, X. Dou, J. Liu, L. Yuan, W. Zhang and H. Wang, Immunomodulation and signaling mechanism of Lactobacillus rhamnosus GG and its components on porcine intestinal epithelial cells stimulated by lipopolysaccharide, J. Microbiol., Immunol. Infect., 2017, 50, 700e713 Search PubMed.
- B. Ismail and K. M. Nampoothiri, Production, purification and structural characterization of an exopolysaccharide produced by a probiotic Lactobacillus plantarum MTCC 9510, Arch. Microbiol., 2010, 19, 1049 CrossRef PubMed.
- J. Y. Li, M. M. Jin, J. Meng, S. M. Gao and R. R. Lu, Exopolysaccharide from Lactobacillus planterum LP6: antioxidation and the effect on oxidative stress, Carbohydr. Polym., 2013, 98, 1147 CrossRef CAS PubMed.
- D. M. Remus, R. van Kranenburg, I. I. van Swam, N. Taverne, R. S. Bongers, M. Wels, J. M. Wells, P. A. Bron and M. Kleerebezem, Impact of 4 Lactobacillus plantarum capsular polysaccharide clusters on surface glycan composition and host cell signaling, Microb. Cell Fact., 2012, 11, 149 CrossRef CAS PubMed.
- K. Wang, W. Li, X. Rui, X. Chen, M. Jiang and M. Dong, Structural characterization and bioactivity of released exopolysaccharides from Lactobacillus plantarum, 70810, Int. J. Biol. Macromol., 2014, 67, 71 CrossRef CAS PubMed.
- I. C. Lee, G. Caggianiello, I. I. van Swam, N. Taverne, M. Meijerink, P. A. Bron, G. Spano and M. Kleerebezem, Strain-specific features of extracellular polysaccharides and their impact on Lactobacillus plantarum-host interactions, Appl. Environ. Microbiol., 2016, 82, 3959 CrossRef CAS PubMed.
- T. Dan, K. Fukuda, M. Sugai-Bannai, N. Takakuwa, H. Motoshima and T. Urashima, Characterization and expression analysis of the exopolysaccharide gene cluster in Lactobacillus fermentum TDS030603, Biosci. Biotechnol. Biochem., 2009, 73, 2656 CrossRef CAS PubMed.
- S. Lebeer, T. L. Verhoeven, G. Francius, G. Schoofs, I. Lambrichts, Y. Dufrêne, J. Vanderleyden and S. C. De Keersmaecker, Identification of a gene cluster for the biosynthesis of a long, galactose-rich exopolysaccharide in Lactobacillus rhamnosus GG and functional analysis of the Priming glycosyltransferase, Appl. Environ. Microbiol., 2009, 75, 3554 CrossRef CAS PubMed.
- B. Péant, G. LaPointe, C. Gilbert, D. Atlan, P. Ward and D. Roy, Comparative analysis of the exopolysaccharide biosynthesis gene clusters from four strains of Lactobacillus rhamnosus, 2005, Microbiology, 2005, 151, 1839 CrossRef PubMed.
- C. Suzuki, M. Kobayashi and H. Kimoto-Nira, Novel exopolysaccharides produced by Lactococcus lactis, subsp. lactis, and the diversity of epsE genes in the exopolysaccharide biosynthesis gene clusters, Biosci. Biotechnol. Biochem., 2005, 77, 2013 CrossRef PubMed.
- M. Kleerebezem, J. Boekhorst, R. van Kranenburg, D. Molenaar, O. P. Kuipers, R. Leer, R. Tarchini, S. A. Peters, H. M. Sandbrink, M. W. Fiers, W. Stiekema, R. M. Lankhorst, P. A. Bron, S. M. Hoffer, M. N. Groot, R. Kerkhoven, M. de Vries, B. Ursing, W. M. de Vos and R. J. Siezen, Complete genome sequence of Lactobacillus plantarum, WCFS1, Proc. Natl. Acad. Sci. U. S. A., 2003, 100, 1990 CrossRef CAS PubMed.
- T. Nagai, S. Makino, S. Ikegami, H. Itoh and H. Yamada, Effects of oral administration of yogurt fermented with Lactobacillus, delbrueckii ssp. bulgaricus OLL1073R-1 and its exopolysaccharides against influenza virus infection in mice, Int. Immunopharmacol., 2011, 11, 2246 CrossRef CAS PubMed.
-
C. Nachtigall, C. Vogel, H. Rohm and D. Jaros, How Capsular Exopolysaccharides Affect Cell Surface Properties of Lactic Acid Bacteria. Microorganisms, 2020, 8(12), 1904 Search PubMed.
- Z. Ren, T. Cui, J. Zeng, L. Chen, W. Zhang, X. Xu, L. Cheng, M. Li, J. Li, X. Zhou and Y. Li, Molecule targeting glucosyltransferase inhibits Streptococcus mutans biofilm formation and virulence, Antimicrob. Agents Chemother., 2016, 60, 126 CrossRef CAS PubMed.
- U. K. Laemmli, Cleavage of structural proteins during the assembly of the head of bacteriophage T4, Nature, 1970, 227, 680 CrossRef CAS PubMed.
- B. Gareth, W. Jie, Z. Wei, Y. Wenhong, S. Anthony, W. Peter, F. G. Jack, E. Andrew and B. Z. Deborah, Interactions of the Escherichia coli hydrogenase biosynthetic proteins: HybG complex formation, FEBS Lett., 2006, 580, 677 CrossRef PubMed.
- G. Corinne, N. Sophie, P. Emmanuelle, M. Siegfried, H. Corinna, D. Joelle, P. Bruno, H. Thomas, H. Pascal and M. Annick, Enhanced antiinflammatory capacity of a Lactobacillus plantarum mutant synthesizing modified teichoic acids, Proc. Natl. Acad. Sci. U. S. A., 2005, 102, 10321 CrossRef PubMed.
- S. van Hemert, M. Meijerink, D. Molenaar, P. A. Bron, P. de Vos, M. Kleerebezem, J. M. Wells and M. L. Marco, Identification of Lactobacillus plantarum genes modulating the cytokine response of human peripheral blood mononuclear cells, BMC Microbiol., 2010, 10, 293 CrossRef PubMed.
- M. A. Sirover, Moonlighting glyceraldehyde-3-phosphate dehydrogenase: posttranslational modification, protein and nucleic acid interactions in normal cells and in human pathology, Crit. Rev. Biochem. Mol. Biol., 2020, 55, 354 CrossRef CAS PubMed.
- R. A. Alvarez, M. W. Blaylock and J. B. Baseman, Surface localized glyceraldehyde-3-phosphate dehydrogenase of Mycoplasma genitalium binds mucin, Mol. Microbiol., 2003, 48, 1417 CrossRef CAS PubMed.
- E. Ferreira, R. Giménez, L. Aguilera, K. Guzmán, J. Aguilar, J. Badia and L. Baldomà, Protein interaction studies point to new functions for Escherichia coli, glyceraldehyde-3-phosphate dehydrogenase, Res. Microbiol., 2013, 164, 145 CrossRef CAS PubMed.
- Y. Bai, M. Lyu, K. Miyanaga and N. Yamamoto, Tight junction associating Lactobacillus johnsonii, promotes anti-inflammatory effect on DSS treated mouse, Food Funct., 2022, 13, 11021 RSC.
- T. Nakano, S. Goto, Y. Takaoka, H.-P. Tseng, T. Fujimura, S. Kawamoto, K. Ono and C.-L. Chen, A novel moonlight function of glyceraldehyde-3-phosphate dehydrogenase (GAPDH) for immunomodulation, Biofactors, 2018, 44, 597 CrossRef CAS PubMed.
- X. Sun, J. Wang, J. Zhou, H. Wang, X. Wang, J. Wu, Y. He, Y. Yin, X. Zhang and W. Xu, 2017. Subcutaneous immunization with Streptococcus pneumoniae GAPDH confers effective protection in mice via TLR2 and TLR4, Mol. Immunol., 2017, 83, 1 CrossRef CAS PubMed.
- M. J. Sullivan, K. G. K. Goh, R. Thapaa, D. Chattopadhyay, D. S. Ipe, B. L. L. Duella, L. Katupitiyaa, D. Goslinga, D. Acharyaa and G. C. Uletta,
Streptococcus agalactiae glyceraldehyde-3-phosphate dehydrogenase (GAPDH) elicits multiple cytokines from human cells and has a minor effect on bacterial persistence in the murine female reproductive tract, Virulence, 2021, 12, 3015 CrossRef CAS PubMed.
- T. Kaisho and S. Akira, Toll-like receptors as adjuvant receptors, Biochim. Biophys. Acta, 2002, 1589, 1–13 CrossRef CAS PubMed.
- M. Kopeckova, I. Pavkova and J. Stulik, Diverse localization and protein binding abilities of glyceraldehyde-3-Phosphate dehydrogenase in pathogenic bacteria: The key to its multifunctionality?, J. Front. Cell Infect. Microbiol., 2020, 10, 89 CrossRef CAS PubMed.
- V. Hurmalainen, S. Edelman, J. Antikainen, M. Baumann, K. Lahteenmaki and R. K. Korhonen, Extracellular proteins of Lactobacillus crispatus enhance activation of human plasminogen, Microbiology, 2007, 153, 1112 CrossRef CAS PubMed.
- P. Kelly, P. B. Maguire, M. Bennett, D. Fitzgerald, R. J. Edwards, B. Thiede, A. Treumann, J. K. Collins, G. C. O'sullivan, F. Shanahan and C. Dunne, Correlation of probiotic Lactobacillus salivarius, growth phase with its cell wall associated proteome, FEMS Microbiol. Lett., 2005, 252, 153 CrossRef CAS PubMed.
- J. M. Lamonica, M. Wagner, M. Eschenbrenner, L. E. Williams, T. L. Miller, G. Patra and V. G. Del-Vecchio, 2005. Comparative secretome analyses of three Bacillus anthracis strains with variant plasmid contents, Infect. Immun., 2005, 73, 3646 CrossRef CAS PubMed.
- V. Pancholi and V. A. Fischetti, 1992. Major surface protein on group A streptococci is a glyceraldehyde-3-phosphate dehydrogenase with multiple binding activity, J. Exp. Med., 1992, 176, 415 CrossRef CAS PubMed.
- H. Kinoshita, H. Uchida, Y. Kawai, T. Kawasaki, N. Wakahara, H. Matsuo, Y. Kawai, H. Kitazawa, S. Ohnuma, K. Miura, A. Horii and T. Saito, Cell surface Lactobacillus plantarum LA 318 glyceraldehyde-3-phosphate dehydrogenase (GAPDH) adheres to human colonic mucin, J. Appl. Microbiol., 2008, 104, 1667 CrossRef CAS PubMed.
- K. Ramiah, C. A. Van Reenen and M. T. Dicks, Surface-bound proteins of Lactobacillus plantarum, 423 that contribute to adhesion of Caco-2 cells and their role in competitive exclusion and displacement of Clostridium sporogenes and Enterococcus faecalis, Res. Microbiol., 2008, 159, 470 CrossRef CAS PubMed.
- N. Saad, N. Urdaci, C. Vignoles, S. Chaignepain, R. Tallon, J. M. Schmitter and P. Bressollier,
Lactobacillus plantarum, 299v surface-bound GAPDH: a new insight into enzyme cell walls location, J. Microbiol. Biotechnol., 2009, 19, 1635 CrossRef CAS PubMed.
Footnote |
† Electronic supplementary information (ESI) available: Table S1. Sets of primers for RT-PCR amplifications. Table S2. Significantly upregulated gene expressions in THP-1 DCs by the treatment with GAPDH. Table S3. Significantly downregulated gene expressions in THP-1 DCs by treatment with GAPDH. Fig. S1. Productions of GAPDH on cell surface with or without EPS inhibitor during cultivation of L. plantarum JCM 1194. Fig. S2. Western blotting analyses of Slps in L. plantarum JCM 1149, 064 and A73. Fig. S3. Whole network detected in upregulated and downregulated gene expressions in THP-1 DC by the treatment with GAPDH. Fig. S4. Estimated contributions of protein and EPS on IL-10 inducing activities of fractions of gel filtration (Bio-gel P-100) and combined activity. See DOI: https://doi.org/10.1039/d2fo02927h |
|
This journal is © The Royal Society of Chemistry 2023 |