DOI:
10.1039/D2FO03028D
(Paper)
Food Funct., 2023,
14, 500-515
Tetrastigma hemsleyanum leaf extracts ameliorate NAFLD in mice with low-grade colitis via the gut–liver axis†
Received
10th October 2022
, Accepted 8th December 2022
First published on 15th December 2022
Abstract
Nonalcoholic fatty liver disease (NAFLD) is a complex metabolic disorder, manifested as oxidative stress, lipid accumulation, and inflammation of the liver. Tetrastigma hemsleyanum leaves (THL), which are rich in flavonoids and phenolic acids, have good anti-inflammatory, antioxidant, and hepatoprotective effects. However, it is unknown whether THL extracts can improve NAFLD and the underlying mechanisms are unknown. Hence, this study was designed to investigate the effects of THL extracts on NAFLD and perform a preliminary inquiry into the underlying mechanism based on the gut–liver axis. The results showed that THL extracts could reverse NAFLD-related oxidative stress, lipid accumulation, and inflammation. Additionally, the protective effect of THL extracts on the gut includes the maintenance of the intestinal barrier and the regulation of gut microbiota, which may be one of the mechanisms by which THL improves NAFLD. To be specific, in our study, THL extracts alleviated hepatic lipid accumulation and oxidative stress by regulating the expression of lipid synthesis/catabolism and the oxidative stress genes (SREBP-1c/ACC-1/PPAR-α/PPAR-γ/Keap1/Nrf2). In addition, THL extracts reduced damage to the intestinal barrier (ZO-1/Mucin2/occludin) and increased the relative abundance of Lactobacillales, Ruminococcaceae, and Bifidobacteriales in NAFLD mice. In short, THL extracts alleviated NAFLD-related oxidative stress, lipid accumulation, and inflammation in NAFLD mice which may be via the gut–liver axis (gut barrier integrity and gut microbiota).
1. Introduction
Unhealthy diets such as high-sugar and high-fat diets are causative factors for the development of metabolic disorders. Non-alcoholic fatty liver disease (NAFLD) is a metabolic disease manifested in the liver, and includes a series of pathological processes. It varies from simple steatosis (non-alcoholic fatty liver disease, NAL) to non-alcoholic steatohepatitis (NASH). NASH eventually leads to liver fibrosis, which further develops into liver cirrhosis and hepatocellular carcinoma (HCC).1 NAFLD has been a threat to global health, affecting about 25% of people worldwide according to a recent meta-analysis.2 Among people suffering from NAFLD, about 20% may develop into NASH and among those 20% ultimately develop into HCC.3 For this reason, early nutritional therapy is an important means of intervening in NAFLD. However, the complex pathogenesis poses a major challenge for NAFLD treatment. The “two-hit hypothesis” theory was often used to explain the pathological process of NAFLD and NASH in earlier studies. Yet, it is now believed that “multiple hits”, rather than “two hits”, are a more reasonable interpretation of NAFLD. The “first hit” mainly appears as lipid accumulation, hepatic triglyceride accumulation, and insulin resistance which may be one of the culprits of diseases like diabetes, hypertension, and fatty liver. The “second hit” is accompanied by a cascade of inflammation, mitochondrial dysfunction, and oxidative stress.4–6 Accordingly, the “multiple hit” theory has attracted the attention of researchers due to the emphasis on the involvement of multiple associated organs and tissues as well as gut microbiota.4,7 Plenty of evidence has demonstrated that gut microbiota plays a key role in the prevention and treatment of NAFLD in a gut–liver axis-dependent manner. Therefore, therapies for NAFLD are not only concentrated on the pathological manifestations in the liver but also on the involvement of the gut microbiome.
The gut–liver axis is a crosstalk between the gut and liver through the portal circulation, bile duct, and systematic circulation. A lot of data implicated the gut microbiome and its metabolites in the development of NAFLD. The prevalence of NAFLD among inflammatory bowel disease (IBD) patients was 32%, which is higher than the prevalence of NAFLD in the general population from a meta-analysis.8 Evidence of NAFLD in humans indicated that the impaired gut barrier exacerbates hepatic fat accumulation and hepatic steatosis.9 Therefore, the maintenance of gut health plays a critical role in NAFLD intervention strategies. The intestinal mucosal epithelium is a natural barrier that absorbs nutrients and prevents harmful substances in the gut from entering the portal circulation and harming the liver. The integrity of the intestinal barrier is maintained by tight junction (TJ) proteins, including Mucin2, ZO-1, and occludin.10 The increased intestinal permeability increases some gut-derived products such as lipopolysaccharide (LPS), bile acids (BAs), and cytokines to enter enterohepatic circulation, which results in liver exposure to these derived products. Subsequently, LPS activates the toll-like receptor (TLR) mediated inflammatory pathway, which increases the risk of developing NAFLD.11 Overall, the consequences of a leaky gut can lead to liver damage, inflammation, and even NASH. Additionally, intestinal inflammation and disturbance of the intestinal microenvironment are other risk factors for NAFLD. Existing clinical data and animal experiments have shown that intestinal inflammation increased liver damage and hepatic fat accumulation.12,13 Previous studies had confirmed that gut inflammation exacerbates liver injury and fibrosis in C57BL/6 mice which were fed a high-fat diet (HFD) and dextran sulfate sodium salt (DSS).14 Therefore, normal intestinal homeostasis including gut barrier integrity and gut microbiota balance will contribute to the improvement of NAFLD. However, the “gut–liver” axis theory is complex and not simply described here. Herein, we have focused on “gut barrier integrity” and “gut microbiota” in the gut–liver axis.
In recent years, phytochemicals rich in bioactives such as phenolics and flavonoids have been shown to be ideal for the prevention of NAFLD. Tetrastigma hemsleyanum Diels et Gilg, a kind of edible and renascent herb rich in flavonoids and phenolic acid compounds, is widely used in folk medicine. It is known as one of the functional foods due to its antioxidant, anti-inflammatory, and hepatoprotective effects.15 In our previous research, the antioxidant, antiproliferative, and antitumor activities of THL have been demonstrated. It was found that 80% methanol extract of THL exhibited excellent antioxidant activity.16 We also carried out qualitative and quantitative analyses of the bioactives in THL and their metabolic changes in vitro and in vivo in our previous research.17,18 13 phenolic compounds (3-caffeoylquinic acid, 5-caffeoylquinic acid, 1-caffeoylquinic acid, 5-p-coumaroylquinic acid, isoorientin-2′′-O-rhamnoside, isoorientin, orientin-2′′-O-rhamnoside, orientin, 1-p-coumaroylquinic acid, vitexin-2′′-O-rhamnoside, isovitexin-2′′-O-rhamnoside, vitexin, and isovitexin) in THL were identified in our preliminary study.16 Our recent research observed that THL extracts could relieve DSS-induced colitis by protecting the intestinal epithelial barrier and regulating gut microbiota.19 As for liver disease, many studies have observed their antitumor effect in vitro, and their effect on liver injury in vivo.17,20 However, most of these studies focused on the whole Tetrastigma hemsleyanum plant or the roots, and the liver protective effect of THL was recorded in ancient books. The effects of THL on NAFLD remain unknown. Especially in the case of low-grade gut inflammation exacerbating NAFLD, it was unknown whether THL extracts and their metabolites can reduce intestinal inflammation while alleviating NAFLD.
With regard to the above, we explored whether THL extracts and their metabolites inhibit the development of NAFLD and further elucidated the underlying mechanisms. Thus, C57BL/6 mice were treated with HFD and DSS to induce NAFLD with low-grade colitis. Treatment groups were supplemented with THL extracts. Relevant physiological and molecular parameters in the liver, colon, and serum were assessed to determine the therapeutic potential of THL, respectively. Overall, this study aims to explore whether THL extracts ameliorate NAFLD through the gut–liver axis, especially their effects on gut integrity and liver immune homeostasis.
2. Materials and methods
2.1 Equipment and reagents
A total of 48 specific pathogen-free (SPF) male C57BL/6 mice were purchased from Hunan Slack Jingda Experimental Animal Co., Ltd; Tetrastigma hemsleyanum leaves came from Shangrao Red Sun Agricultural Development Co., Ltd (Jiangxi, China). DSS was purchased from MP Biomedicals, USA. Isoflurane was purchased from Rick Ward, China. Malondialdehyde (MDA), superoxide dismutase (SOD), total triglycerides (TG), total cholesterol (TC), high-density lipoprotein cholesterol (HDL-c), low-density lipoprotein cholesterol (LDL-c), total bile acid (TBA), aspartate aminotransferase (AST) and alanine aminotransferase (ALT) kits were bought from the Nanjing Jiancheng Biological Engineering Research Institute (Nanjing, China). Interleukin-1β (IL-1β), interleukin-6 (IL-6), and mice tumor necrosis factor-α (TNF-α) ELISA kits were obtained from Beyotime Biotechnology Co., Ltd (Shanghai, China). RNA isolation and purification kits were from Beyotime (Shanghai, China). The cDNA reverse transcription kit and Q-PCR fluorescence quantitative kit were purchased from Takara Biomedical Technology (Beijing, China). ZO-1 (Lot: #5406) and Mucin2 (Lot: #88686) antibodies were purchased from Cell Signaling Technology (Danvers, USA). Instruments: Microplate reader (Thermo Fisher Technology Co., Ltd), fluorescence quantitative PCR machine (BIO-RAD, USA), high-speed tissue grinder (Wuhan Saiweier Technology Co., Ltd, China), and Nikon inverted fluorescence microscope (Tokyo, Japan).
2.2 Animal experiments
2.2.1 Sample preparation.
THL extracts extracted from 80% methanol were dissolved to form high-dose (500 mg kg−1) and low-dose (100 mg kg−1) THL extract solution for intragastric administration into mice. The specific sample extraction method can refer to Sun Yong et al.16 The dried powder (0.2 kg) of THL was extracted with 80% methanol (1
:
20) for 90 min at 60 °C by reflux extraction. The organic extracts were centrifuged at 5000 rmp for 10 min. The supernatant was collected and evaporated at 45 °C by a rotary vacuum evaporator (Eyela N-100, Japan). Then, the concentrated solution was dried by a vacuum drying oven (StableTemp XQ-05053-10, USA) to obtain the final extract.
2.2.2 Animal treatment.
48 SPF male C57BL/6 mice with an initial weight of 20–22 g were provided by the Experimental Animal Center of Jiangxi University of Traditional Chinese Medicine. The animals were uniformly labeled and housed in an animal room under standard conditions (indoor temperature: 20 ± 2 °C, humidity: 60 ± 5%, 12 h day/night cycle, no specific pathogens). This experiment has been approved by the Animal Ethics Committee of Jiangxi University of Traditional Chinese Medicine (No. 2020-0018).
The animal experimental design is shown in Fig. 1A. After adaptive feeding for one week, a total of 48 mice were randomly divided into 4 groups (12 mice/group) using a random number table and four mice were kept in one cage: normal control group (NC group), high-fat diet + DSS group (HFD + DSS), low-dose 100 mg per kg body weight per day THL extract group (L-THL) and high-dose 500 mg per kg body weight per day THL extract group (H-THL). All the mice were sacrificed after anesthesia with 2.5% isoflurane on the 16th week. In addition, to assess the occurrence of NAFLD under a high-fat diet, a group of mice was established on a high fat diet without DSS and some parameters were measured, which can be found in the ESI.†
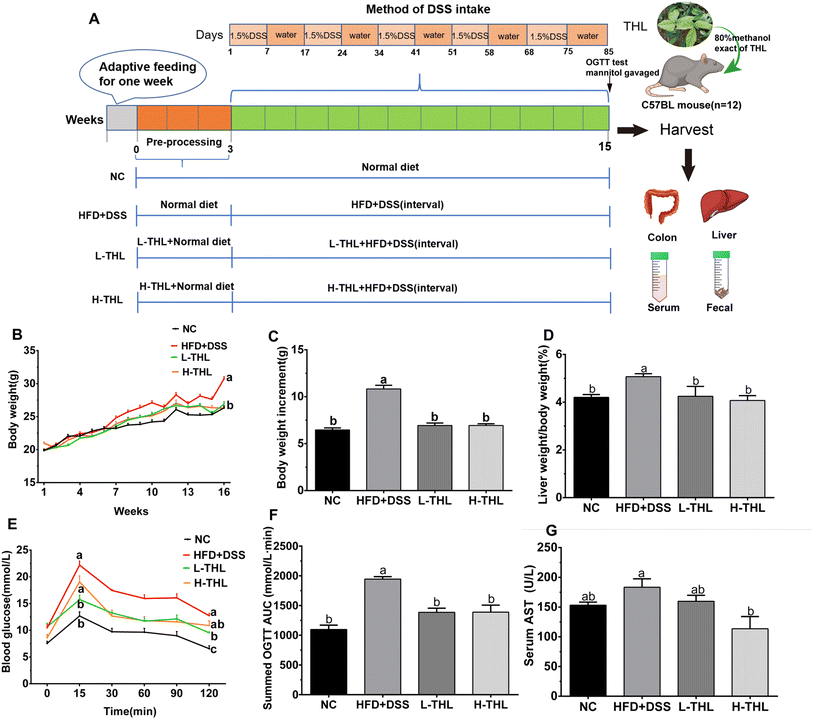 |
| Fig. 1 Effects of THL extracts on weight gain, liver damage and glucose tolerance caused by HFD and DSS. (A) Animal experiment design. (B–D) Body weight, body weight increment, relative liver weight (n = 11). (E and F) Blood glucose of OGTT, AUC of OGTT, n = 6. (G) Serum AST, n = 6. Significant differences (P ≤ 0.05) expressed with different letters (a–c). One-way ANOVA with Tukey–Kramer's test was used to compare the data among different groups. | |
The methods of administration or feeding were as follows: (1) the NC group was provided with a normal diet and free drinking water for 15 weeks. (2) The HFD + DSS group was provided with a normal diet during the 1st–4th weeks and high-fat feed during the 4th–16th weeks, and DSS was administered at intervals after the 4th week. The specific formula of the high-fat feed was 15% lard, 2% cholesterol, 0.5% bile salts, 15% sucrose, and 67.5% normal feed. The information about the diet of mice can be referred to in ESI Table S1.† (3) The L-THL extract group was given a high-fat diet and low-dose (100 mg kg−1) of THL extracts and the H-THL extract group was administered a high-fat diet and high-dose (500 mg kg−1) of THL extracts respectively for 15 weeks. Similarly, 1.5% w/w DSS was administered at intervals after the 4th week. The DSS interval administration method was as follows:
Except for the NC group, all the other groups were administered with 1.5% w/w DSS at intervals (DSS solution for 7 days followed by normal drinking water for 10 days). During the experiment, the daily mental and behavioral state, food intake (Fig. S1†), and defecation of the mice were observed, and the weight of mice was monitored and recorded once a week. The serum as well as liver, colon and other tissues were collected and frozen at −80 °C for subsequent experiments after the mice were sacrificed on the 16th week.
2.3 Glucose tolerance test and intestinal permeability test
Six experimental mice in each group were selected randomly after fasting for 6 hours, and they underwent 2 hours of OGTT test. The mice were given a dose of 2 g kg−1 glucose dissolved in distilled water (glucose solution concentration: 0.2 g ml−1) by gavage, and the caudal vein blood samples were collected for measurement at 0 min (before gavage), 15 min, 30 min, 60 min, 90 min and 120 min after gavage respectively. The data at 0 min could be recorded as fasting blood glucose. The results were expressed as the area under the curve (AUC).
Similarly, 6 mice in each group fasted for 6 hours before the experiment and were given mannitol (0.6 g kg−1) by gavage. Then, after 2 hours of fasting and water restriction, blood was collected from the mice, and serum was separated to determine the concentration of D-mannitol.
2.4 Pathological analysis of tissue sections
2.4.1 H&E staining of colon tissue.
Fresh mouse colon tissue was washed with PBS and fixed in 4% paraformaldehyde. The materials were taken for paraffin embedding; they were then sliced (4 μm) and baked. After dewaxing, they were stained with hematoxylin and eosin in sequence. After gum mounting, the degree of inflammatory damage in colon tissue was observed under an optical microscope, including mucosal damage, crypt damage and overall cell morphology.
2.4.2 Oil red staining of liver tissue.
Fresh liver tissue was collected and fixed into paraffin sections with 4% paraformaldehyde. After dewaxing, rewarming, immersion, staining with Oil Red staining solution, and hematoxylin counterstaining and rinsing, the slides were mounted for microscopic examination. Finally, the lipid deposition on liver slice tissue was observed.
2.5 Immunofluorescence analysis of colon tissue
The colon tissue was analyzed by immunofluorescence, and the slice preparation method was the same as before. After the sections were deparaffinized and hydrated, antigen retrieval was performed in citrate buffer, blocked with 5% BSA for 60 minutes, and then blocked with ZO-1 and Mucin2 antibodies. Sections were observed under a Nikon inverted fluorescence microscope.
2.6 Inflammatory cytokine detection
20 mg of liver tissue was added to PBS at a ratio of 1
:
9 for homogenization, centrifuged to get the supernatant, and the IL-6, TNF-α, and IL-1β of the liver homogenate were detected by enzyme linked immunosorbent assay (ELISA) kit according to the instructions. Similarly, the serum obtained after centrifugation was tested for the levels of IL-6, TNF-α, IL-1β, and LPS by the ELISA kit according to the instructions.
2.7 Biochemical measurements
The collected blood was allowed to stand for 2 hours, centrifuged at 2000 rpm for 20 min, and the supernatant was taken as the serum to be detected. Serum levels of TBA, TG, TC, HDL-c, and LDL-c were detected with an automatic biochemical analyzer.
2.8 Real-time quantitative PCR analysis
Total RNA from colon and liver tissue was extracted using an RNA isolation and purification kit to determine RNA concentration. The extracted total RNA was reverse-transcribed into cDNA using a cDNA reverse transcription kit. The reverse transcribed cDNA was added to TB Green™ Premix Ex Taq™ II (Tli RNaseH Plus) (TaKaRa, Japan) and quantified on an RT-PCR fluorometer (BIO-RAD, USA). The reaction conditions were as follows: 95 °C for 30 s, 95 °C for 5 s, and 60 °C for 20 s for 40 cycles. GAPDH was used as a reference gene to detect the relative expression level of the target gene. The primer sequence list is shown in Table 1. The dissolution curve was plotted and the final data were expressed using the 2−ΔΔct method.
Table 1 Gene primers used for real-time quantitative qPCR in this study
Primer |
Gene ID |
Forward primer |
Reverse primer |
SREBP-1c |
78968 |
GCTTCTCTTCTGCTTCTCTGG |
CAGGCTGTAGGATGGTGAGTG |
ACC-1 |
107476 |
AGAATTTGTTACTCGTTTTGG |
TTCTGCATTGGCTTTAAGGTC |
PPAR-α |
19013 |
CACAATGCAATTCGCTTTGG |
TCTTTCCCGCGAGTATGA |
PPAR-γ |
19016 |
ACCAAAGTGCGATCAAAG |
GGATCCGGCAGTTAAGAT |
Keap1 |
50868 |
TGCACCCAAGGCTTTTGAAC |
TAGCAATCCAGCAGCACATG |
Nrf2 |
18024 |
AGCATTGCACATGGAAGCAG |
AACCGCAACGAGAGCAATTG |
HO-1 |
15368 |
TTCCTCACCTGCAAAAGCAC |
ACTGTGCATTGGTGAGTTCC |
NQO1 |
18104 |
AGCTTTGTGGTGCAAACTGG |
AAGCAACGCACTTCCCAAAC |
GCLC |
14629 |
ACACACTCGTTTGGCAGTTC |
AAGGACGCCACAATTTGCTG |
GAPDH |
14433 |
AGACCCACACTTCTCCATTTCC |
TGAAATGTGCACGCACCAAG |
Occludin |
18260 |
GCTGTTGTCGCACCTGTTTT |
TGCGGCTTCGAAGACATTCT |
ZO-1 (TJP-1) |
21872 |
AGGACACCAAAGCATGTGAG |
GGCATTCCTGCTGGTTACA |
2.9 Short-chain fatty acid (SCFA) analysis
(1) 500 μl of sterile PBS buffer (pH 7.2–7.4) was added to each 250 mg of stool sample, then vortexed to mix and sonicated for 10 min (100 w). (2) Then 20 μl of 10% sulfuric acid was added to the sample and vortexed for 30 s. (3) Subsequently, 800 μl of ether was added to the sample and vortexed for 30 s. (4) Finally, centrifugation at 10
000 rpm for 20 min was performed and the upper ether organic phase was collected. (5) Steps (3) and (4) were repeated, then the upper organic phases were merged and concentrated to 500 μl with nitrogen blowing. (6) The sample was filtered through 0.22 μm organic membrane and the content of short-chain fatty acids was quantified using a GC-FID detector. (7) The procedure for increasing the gradient temperature was as follows: the initial column oven temperature was 75 °C for 0.5 min and then the temperature was increased to 140 °C for 3 min at a rate of 6 °C min−1. And finally, the temperature was increased to 190 °C at a heating rate of 6 °C min−1. The total run time was 25 min. A HP-5 ms (5%-phenyl-methylpolysiloxane) capillary GC column (30 m × 0.320 mm i.d. 0.25 μm film thickness, Agilent Technologies) was applied running at 1 μl of sample injection volume and a rate of the carrier gas at 6.5 mL min−1.
2.10 16S rRNA gene sequencing
Cecal contents were used for bacterial DNA extraction using an E.Z.N.A.R stool DNA kit (Omega Bio tek Inc., USA). The hypervariable regions v3–v4 of 16sRNA were applied for amplification using universal primers. After library preparation, quality inspection, quantification, and sample differentiation using the set TAG sequence, the Illumina Hiseq 2500 high-throughput sequencing platform was used to sequence the qualified library. Finally, OTU cluster analysis and taxonomy annotation were performed on the obtained optimized sequences. Gut microbiota analysis service was provided by Beijing Microread Genetics Co., Ltd.
2.11 Statistical analysis
The graphics were obtained by the GraphPad Prism 8.0 software and all values were expressed as the mean ± standard error of mean (SEM). One-way ANOVA with Tukey–Kramer's test was used to compare the data among different groups by the SPSS 21.0 software. Pearson's correlation coefficient (r) was used to quantify the correlation between pairs of parameters. p ≤ 0.05 expressed with different letters (a–c) was considered to be statistically significant.
3. Results
3.1 THL extracts attenuated NAFLD and NAFLD-related inflammation, lipid accumulation and oxidative stress in the liver
3.1.1 Effects of THL extracts on weight gain, liver damage and glucose tolerance caused by HFD and DSS.
Since NAFLD is closely related to obesity and the metabolic state in vivo, we recorded body weight, relative liver weight (expressed by the liver weight to body weight ratio), and glucose tolerance in mice. As shown in Fig. 1B, the average body weight of the mice in the HFD + DSS group started to be higher than that of mice in the other three groups on the 7th week, and the results were consistent in the last week (p ≤ 0.05). Significant increases in body weight increment and relative liver weight were observed in the HFD + DSS group after 15 weeks compared with the THL extract administration groups (Fig. 1C and D). Research has shown that glucose level was directly linked to liver fibrosis in NAFLD and the glucose tolerance status reflects the metabolism status in vivo.21 Therefore, we measured the oral glucose tolerance (OGTT) of the mice before they were sacrificed. As shown in Fig. 1E, the blood glucose level rose to a maximum at 15 minutes, and the blood glucose level in the HFD + DSS group was significantly higher than in the THL extract intervention groups. After 15 min, blood glucose decreased in each group. However, compared with the HFD + DSS group, the THL extracts significantly improved the glucose clearance rate in mice. The area under the curve of OGTT (AUC) showed that glucose intolerance was improved obviously in the two THL extract groups vs. the HFD + DSS group (Fig. 1F). We measured the level of aspartate aminotransferase (AST) in serum, which could preliminarily determine whether the liver is in a pathological state (Fig. 1G). The result showed that serum AST was decreased in the H-THL group, significantly. As shown in Fig. S2,† the body weight, and liver weight were increased in the HFD group but the level of blood glucose did not rise significantly. It may be that only mild NAFLD had occurred in the HFD group compared with the HFD + DSS group.
The above results showed that THL extracts can improve HFD and DSS-induced weight gain, fat accumulation in the liver, hepatic injury, and glucose tolerance.
3.1.2 Effects of THL extracts on inflammatory status in the liver.
Since inflammatory status is one of the manifestations of obesity and NAFLD, we evaluated the ability of THL extracts to reduce the level of inflammation in mice, including IL-6, TNF-α and IL-1β levels in serum and the liver (Fig. 2A and C). The results showed that the level of inflammation decreased after THL extract intervention in NAFLD mice with intestinal injury. As shown in Fig. 2B, there was a lower MCP-1 level in the two doses of THL extract treatment groups and NC group compared with that in the HFD + DSS group, indicating an anti-inflammatory effect of THL extracts on NAFLD.
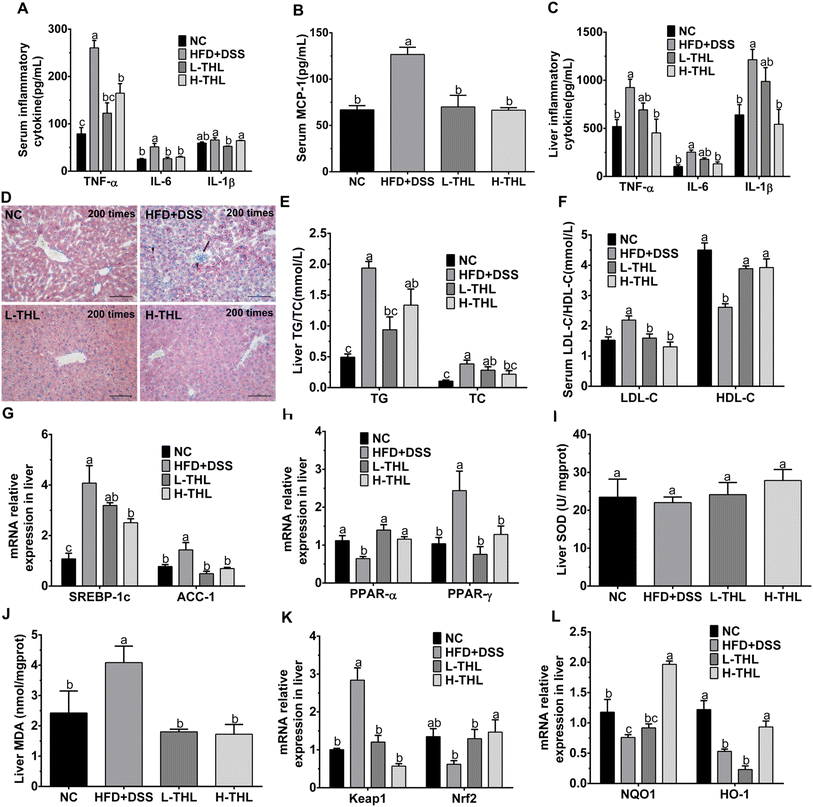 |
| Fig. 2 Effects of THL extracts on inflammation, lipid metabolism and oxidative stress in NAFLD mice. (A) Inflammatory cytokine in serum, n = 6. (B) Serum MCP-1, n = 6. (C) Inflammatory cytokine in the live, n = 6. (D) Oil Red O staining of liver sections at 200 times (scale bars = 100 μm). (E) Liver TG/TC, n = 6 (F) LDL-c, HDL-c in serum. n = 6 (G and H) relative mRNA expression levels of lipid oxidation and synthesis (PPAR-α, PPAR-γ, SREBP-1c, ACC-1), n = 4. (E and J) Liver SOD and MDA, n = 6. (K and L) Relative mRNA expression levels of oxidative stress (Keap1, Nrf2, NQO1, HO1), n = 4. Significant differences (P ≤ 0.05) expressed with different letters (a–c). One-way ANOVA with Tukey–Kramer's test was used to compare the data among different groups. | |
3.1.3 Effects of THL extracts on hepatic lipid metabolism and hepatic morphology.
To further investigate whether the liver injury and lipid accumulation induced by HFD and DSS were improved after THL extract intervention, we performed Oil Red O staining of liver tissue sections (Fig. 2D). Obvious lipid aggregation and cell extrusion were visible. After the intervention with THL extracts, lipid droplets were reduced and the cell morphology was similar to that in the NC group (Fig. 2D).
The initial manifestation of NAFLD is the accumulation of lipids, mainly triglycerides and total cholesterol, in the liver. Therefore, we also measured TG and TC in the liver. The results showed that THL extracts could alleviate the accumulation of TG and TC in liver tissue under a high-fat diet with DSS (Fig. 2E). Similarly, the HDL-c which is considered to be “good cholesterol” and the LDL-c which is considered to be “bad cholesterol” were both detected to assess lipid metabolism. There was a higher level of LDL-c and a lower level of HDL-c in the HFD + DSS group. In contrast, THL extracts increased the production of HDL-c and decreased the production of LDL-c (Fig. 2F). These results revealed that THL extracts could reduce hepatic damage and hepatic lipid accumulation, which will contribute to improving NAFLD. As shown in Fig. S3,† some degree of liver damage and lipid accumulation can be found in the HFD group but less than that in the HFD + DSS group. It may be that the intestinal damage caused by DSS aggravated NAFLD.
To further explore the effect of THL extracts on lipid metabolism in NAFLD mice, we determined the expression of some key genes affecting lipid oxidation, lipid synthesis and steatosis in liver tissues, including peroxisome proliferator-activated receptor alpha/gamma (PPARα/γ), acetyl-CoA carboxylase-1 (ACC-1) and sterol response element-binding protein-1c (SREBP-1c). Compared with the HFD + DSS group, expression levels of ACC-1 and SREBP-1c genes were decreased in the THL extract intervention group, and significance (p ≤ 0.05) was observed in the H-THL group (Fig. 2G). PPAR-γ is essential for lipid synthesis. ACC-1 and SREBP-1c are responsible for hepatic de novo fatty acid synthesis. On the contrary, PPAR-α is a ligand-activated transcription factor associated with fatty acid oxidation and breakdown which reduces lipid accumulation in the liver. As the results displayed in Fig. 2H, relative expression of PPAR-α was significantly increased while relative expression of PPAR-γ was decreased in the L-THL and H-THL extract groups (P ≤ 0.05), vs. HFD + DSS group. These data suggested that THL extracts prevented NAFLD-related lipid accumulation by regulating the expression of lipid oxidation and metabolism genes.
3.1.4 Effects of THL extracts on oxidative stress in the liver.
Oxidative stress is a key factor in the development of NAFLD. To evaluate the oxidative stress status in the liver of experimental mice in the study, we detected the levels of superoxide dismutase (SOD) and alondialdehyde (MDA) in liver tissue by biochemical assays, which can reflect liver antioxidant and peroxidation levels, respectively (Fig. 2I and J). Supplementation with THL extracts increases the SOD invisibly but decreases the MDA levels significantly (P ≤ 0.05), vs. The HFD + DSS group.
In addition, we selected the Keap1/Nrf2 signaling pathways which are classical antioxidant signaling pathways to further detect expression of oxidation-related genes, including Kelch ECH associating protein 1 (Keap1), and nuclear factor erythroid 2-related factor 2 (Nrf2) and its downstream antioxidant enzyme genes such as NQO1 and HO-1. As shown in Fig. 2K, the level of Keap1 in the HFD + DSS group was dramatically higher than in the other three groups, which indicated that normal expression of Keap1 could be maintained under the intervention of THL extracts. In contrast, expression of Nrf2 was decreased in the HFD + DSS group but higher in the THL extract intervention group, suggesting that the THL extracts may activate the Keap1/Nrf2 signaling pathways. Therefore, we determined the genes related to antioxidant enzymes downstream of this pathway. A significant upregulation of NQO1 and HO-1 can be observed in the H-THL group, compared with the HFD + DSS group (Fig. 2L). These results suggested that THL can alleviate the hepatic oxidative stress in NAFLD mice.
3.2 THL extracts attenuate NAFLD may via protecting gut barrier integrity and improving colitis
3.2.1 Effects of THL extracts on intestinal barrier integrity.
It has been proved that gut inflammation exacerbated NAFLD. In addition, we have demonstrated that THL extracts could alleviate DSS-induced colitis in previous studies. Based on these, we speculated whether THL can interfere with NAFLD by ameliorating intestinal damage. Therefore, we determined the relevant pathological indicators of the gut.
To determine whether THL extracts alleviated NAFLD via the gut–liver axis, we observed and detected colon damage, intestinal permeability, and intestinal tight junction protein-related indicators. Colon tissue was collected for length measurements and length of the colon was significantly shorter in the HFD + DSS group than in the THL extract and NC groups (Fig. 3A and B). Besides, mice were given mannitol by gavage before sacrifice, and the serum mannitol level of the mice was measured after sacrifice. As the results show, the level of mannitol in serum had increased, indicating damage and a decreased permeability of the colon in the HFD + DSS group (Fig. 1C). However, with both high- and low-dose THL intervention, these impairments were significantly alleviated, manifested in a more normal colon and decreased mannitol levels in serum. H&E staining of the colon showed that the colon section structure was relatively complete, and there was no inflammatory cell infiltration in the mucosal layer in the NC group (Fig. 3D). Compared with the NC group, the structure of the colon was damaged, manifested as thinning of the intestinal wall, infiltration of the inflammatory cells, and exfoliation and necrosis of epithelial cells. However, these symptoms were relieved in the H-THL and L-THL extract groups. Furthermore, from immunofluorescence staining of the tight junction proteins Mucin2 and ZO-1 (Fig. 3E and F), it could be found that expression of ZO-1 and Mucin2 was decreased in the HFD + DSS group but was increased in the NC group, high-dose and low-dose THL extract groups. Therefore, we guessed that THL could maintain normal expression of Mucin2 and ZO-1. To further test the effects of THL extracts on the intestinal barrier, we also measured the gene relative expression of two tight junction proteins, occludin and ZO-1 (TJP-1), by PCR (Fig. 3G). The results were consistent with the fluorescence staining results, and expression of occludin and ZO-1 (TJP-1) was upregulated in the H-THL group but was not effective in the L-THL group. It was an indication that a certain dose of THL extracts could maintain intestinal barrier damage through these results, combined with the previous results of serum mannitol levels. As shown in Fig. S4,† the mice had no significant intestinal damage with the high-fat diet alone.
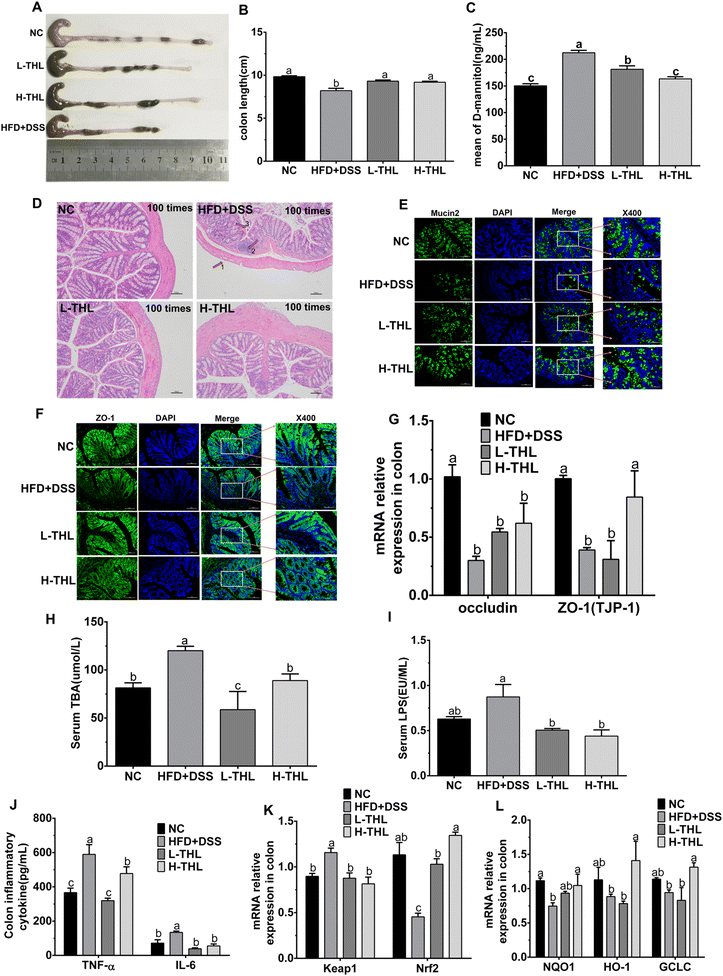 |
| Fig. 3 THL extracts attenuate NAFLD may via protecting gut barrier integrity and improving colitis. (A and B) The length of the colon, n = 6 (E) mean of D-mannitol, n = 6 (D) H&E staining of colon sections at 100 times (scale bars = 100 μm). (E) Fluorescence image of Mucin2 at 200 times (scale bars = 100 μm) and 400 times (scale bars = 50 μm), the target protein was expressed as green fluorescence. (F) Fluorescence image of ZO-1 at 200 times (scale bars = 100 μm) and 400 times (scale bars = 50 μm), the target protein was expressed as green fluorescence. (G) Relative mRNA expression levels of Tight junction protein, n = 4 (H) serum LPS, n = 6 (I) serum TBA, n = 6. (J) Inflammatory cytokine in the colon, n = 6. (K and L) Relative mRNA expression levels of oxidative stress (Keap1, Nrf2, NQO1, HO1, GCLC), n = 4. Significant differences (P ≤ 0.05) expressed with different letters (a–c). One-way ANOVAs with the Tukey–Kramer's test was used to compare the data among different groups. | |
Total bile acid (TBA) is a hallmark metabolite of liver and gut crosstalk, and serum TBA levels can reflect the integrity of the intestinal barrier. The accumulation of TBA can lead to liver damage and further aggravate inflammation.22 Serum TBA levels were increased in HFD + DSS treated mice while they were decreased in the THL extract treated mice (Fig. 3H). Serum LPS, another indicator of gut barrier integrity, is a key bacterial derivative in the colon. We evaluated serum LPS levels and the results showed that HFD and DSS contributed to the massive production of LPS (Fig. 3I). LPS levels decreased both in high-dose and low-dose THL intervention groups. These results suggested that THL extracts reduce the serum TBA and LPS levels.
3.2.2 Effects of THL extracts on inflammatory status in the gut.
To determine the effects of THL extracts on gut inflammation, we evaluated the IL-6 and TNF-α levels in colonic tissue. It demonstrated that the levels of IL-6 and TNF-α were decreased in the THL extract treatment group but were increased in the HFD + DSS group (Fig. 3J). These results combined with the result of LPS illustrated that THL extracts reduce inflammation in the gut.
3.2.3 Effects of THL extracts on inhibiting oxidative stress in the gut.
In our previous study, THL extracts were found to protect against oxidative damage in the gut caused by DSS. In this experiment, we also selected the Keap1/Nrf2 pathway for validation at the gene level (Fig. 3K). Consistent with previous findings, gene expression of Keap1 was upregulated and gene expression of Nrf2 was downregulated in the HFD + DSS group. After THL extract intervention, expression of Keap1 and Nrf2 was opposite to that of HFD + DSS group, which will help Nrf2 activate the downstream antioxidant enzymes. Likewise, we determined gene expression of antioxidant enzymes downstream of the Keap1/Nrf2 pathway in colon tissue including NQO1, GCLC, and HO-1 (Fig. 3L). Expression of these genes was downregulated in HFD + DSS and upregulated in the THL intervention group.
The above data suggested that THL extracts maintained gut barrier integrity and reduced inflammation and oxidative stress in the colon.
3.3 Regulation of THL extracts on gut microbiota composition
3.3.1 Effects of THL extracts on modulating gut microbiota and increasing the SCFA level in NAFLD mice.
Since many of the previous indicators were related to a balanced gut microecology, it was speculated that THL extracts improve NAFLD may be by regulating gut microbiota to a certain extent. To this end, we determined short-chain fatty acids (SCFAs) in feces as well as microbiota composition in cecal contents based on 16 S rRNA gene sequencing. As shown in Fig. 4A, THL extracts increased the production of acetic acid, propionic acid, butyric acid, and total SCFAs, compared with the HFD + DSS group. The results showed that THL extract mainly increased acetic acid production. SCFAs are the product of the gut microbiome, and it is likely that THL extracts promoted the increase of SCFA levels by regulating the gut microbiota. Furthermore, THL extract intervention in NAFLD is may be related to the gut microbiome. Therefore, we further explored the effect of THL extracts on the gut microbial structure.
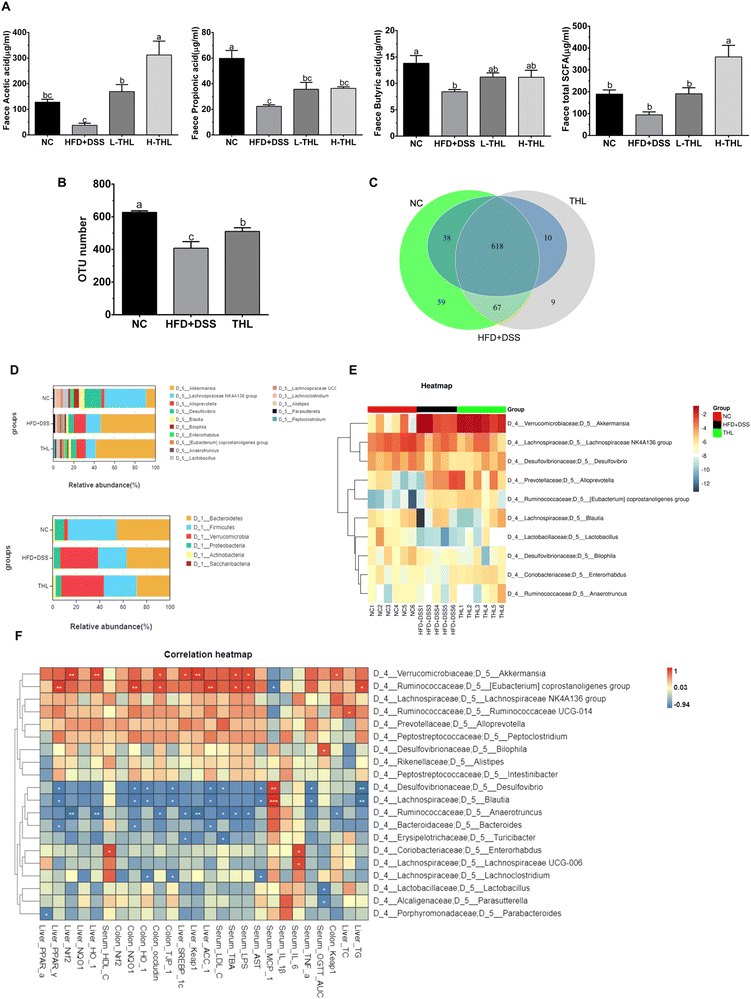 |
| Fig. 4 Effects of THL extracts on abundance and composition of microbiota in NAFLD mice. (A) Total SCFAs of feces and the major SCFAs (B) the total OTUs number in groups. (C) Venn diagram of OTUs (D-top) Relative abundance at phylum level (D-bottom) Relative abundance at genus the level. (E) A heatmap of relative abundance of the top 10 genera. (F) Spearman correlation analysis between the top 20 genera with parameters related to NAFLD in the THL extract group, Pearson's correlation coefficient (r) was used to quantify the correlation between pairs of parameters. n = 6, Significant differences (P ≤ 0.05) expressed with different letters (a–c). One-way ANOVA with Tukey–Kramer's test was used to compare the data among different groups. | |
Since a better effect of high-dose THL extracts was visible based on the results above, we selected the NC group, HFD + DSS group, and H-THL group for 16 S rRNA gene sequencing. In the results of species relative abundance analysis, it can be seen that the number of OTUs in the THL extract treatment group and the NC group was significantly higher than that in the HFD + DSS group (P ≤ 0.05). There were 76 unique OTUs in the THL extract group and 126 unique OTUs in the NC group, compared with the HFD + DSS group (Fig. 4b and c). This result preliminarily indicated that the THL extract group increased the abundance of gut microbiota. Bacteroidetes, Firmicutes and Verrucomicrobia were the main dominant bacteria at the phylum level (Fig. 4D top). At the genus level, Akkermansia, Lanchnospiraceae, and Alloprevotella were the predominant bacteria (Fig. 4D bottom). A heatmap of the relative abundance of the top 10 genera demonstrates that Akkermansia and Lanchnospiraceae were enriched in the THL group (Fig. 4E). Additionally, Spearman correlation analysis was performed to assess the correlation between the top 20 genera with parameters related to NAFLD in the THL extract group (Fig. 4F). The relative abundance of Akkermansia was positively correlated with hepatic Nrf2 (R2 = 0.94, p = 0.005), HO-1 (R2 = 0.92, p = 0.008) and intestinal occludin mRNA expression (R2 = 0.88, p = 0.02). Eubacterium coprostanoligenes group which belongs to Ruminococcaceae was positively correlated with hepatic PPAR-α (R2 = 0.94, p = 0.005) and intestinal occludin (R2 = 0.88, p = 0.02) and was negatively correlated with MCP-1 in serum (R2 = −0.88, p = 0.01). Blautia had negative correlation with hepatic TG (R2 = −0.94, p = 0.005), ACC-1 (R2 = −0.88, p = 0.02) and TNF-α (R2 = −0.88, p = 0.02), AST (R2 = −0.84, p = 0.03) in serum. Notably, these bacteria can be considered to be probiotics in the gut.
In addition, we further observed the difference and diversity of the gut microbiota. Beta diversity was performed with principal coordinates analysis (PCoA) and non-metric multidimensional scaling (NMDS) analysis based on the unweighted unifrac distance. As shown in Fig. 5A, PCoA and NMDS diagrams showed a difference in microbiota structures of the three groups. Alpha diversity analysis including the OTU number, chao1, and Shannon index of the observed species showed higher levels in both NC and THL groups vs. the HFD + DSS group (Fig. 5B). These results indicated that THL interferes with changes in gut microbiota structure and diversity levels. Lefse analysis between the THL and HFD + DSS groups was further performed to search for species that differ significantly between groups. The taxonomic cladogram presented the predominant bacteria with large relative contributions and their relative abundance (Fig. 5C). Similarly, analysis by LDA (linear regression analysis) displayed the biomarkers with a statistical difference (LDA score >2) (Fig. 5D). There was an enrichment of 37 taxa in the THL group while only 3 taxa were enriched in the HFD + DSS group. It is worth noting that there were many probiotics in these 37 differential taxa such as Ruminococcaceae, Lactobacillales, RuminococcaceaeUGG-014, Bifidobacteriales, Lactobacillus, and Lactobacillaceae. In particular, the relative abundance of Ruminococcaceae, Lactobacillales, and Bifidobacteriales showed a significant difference in the THL group vs. the HFD + DSS group (p ≤ 0.05) (Fig. 5E). Based on the above results, most of the probiotics enriched in the THL group belong to the genus Firmicutes. Therefore, we inferred that the effect of THL on gut microbiota structure was mainly due to the regulation of the probiotics in Firmicutes.
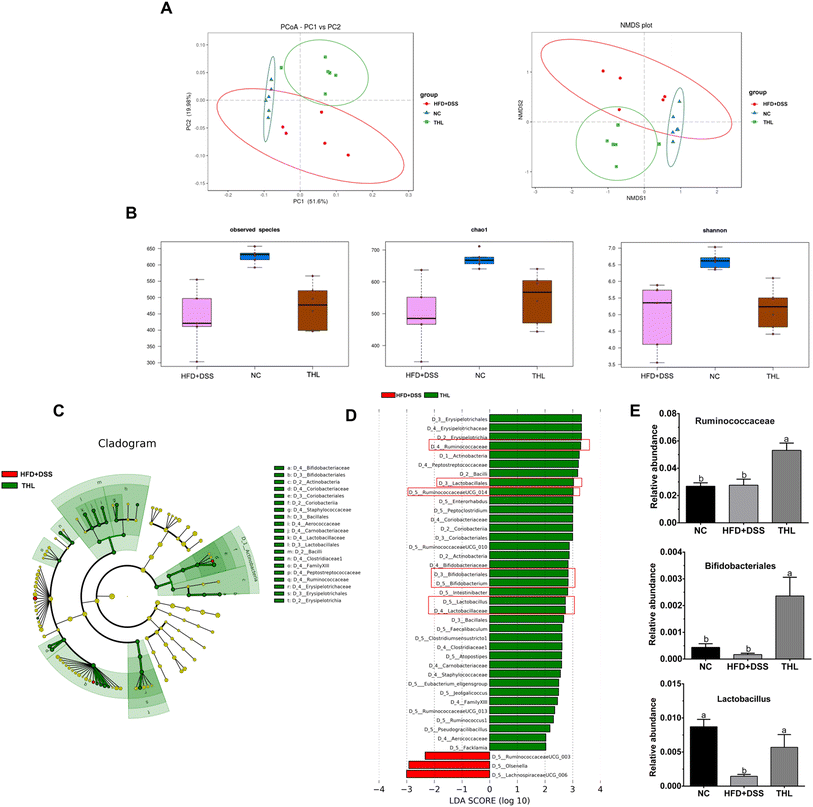 |
| Fig. 5 Effects of THL extracts on diversity and difference analysis of microbiota in NAFLD mice. (A) Beta diversity analysis (B) alpha diversity analysis. (C) Taxonomic cladogram. (D) LDA scores by Lefse analysis between the THL extract group and HFD + DSS group (LDA score >2), which can show the impact extent of species. (E) The relative abundance of some bacteria in the THL extract group from Lefse analysis, may be the key bacteria for THL extracts affecting NAFLD. n = 6, significant differences (P ≤ 0.05) expressed with different letters (a–c). One-way ANOVA with Tukey–Kramer's test was used to compare the data among different groups. | |
4. Discussion
NAFLD, a complex metabolic disease, is closely associated with diabetes, hyperlipidemia, obesity, and other metabolic diseases. A long-term high-fat diet is a common trigger factor for NAFLD and the gut microenvironment influences the development of NAFLD. In this research, we selected THL as the research object and explored the mitigatory effect of THL extracts on NAFLD mice with colitis induced by HFD + DSS for the first time. As the results show above, THL extracts ameliorate NAFLD-related oxidative stress, lipid metabolism and inflammation in the local liver. Notably, our research found that the gut was an important target for the treatment of NAFLD. The effects of THL extracts on gut barrier integrity and the regulation of gut microbiota facilitated the attenuation of NAFLD. It is consistent with the “multiple hits” mentioned above in NAFLD.
From some apparent parameters (body weight, liver weight, and blood glucose), we speculated that THL extracts may reduce lipid accumulation and control blood glucose which reduced the risk factors from obesity for NAFLD. This was consistent with the results of TG, TC and some gene levels for lipid metabolism in the THL extract groups. SREBP-1c, a nuclear transcription factor mainly responsible for regulating genes related to lipid synthesis and uptake, is mainly expressed in hepatocytes and adipocytes and is a target gene for many drugs that interfere with NAFLD-related lipid accumulation. When NAFLD occurred, SREBP-1c is activated by LPS and inflammatory cytokines and subsequently the downstream target genes of SREBP-1c such as ACC-1 is activated.23 Our study showed that THL extracts could inhibit hepatic de novo fatty acid synthesis by downregulating expression of SREBP-1c and ACC-1, although the specific mechanism needs to be further explored. However, adenosine 5′-monophosphate (AMP)-activated protein kinase (AMPK) is a possible target based on a large number of previous studies. For example, vitexin, one of the main ingredients in THL, has been proved to suppress SREBP-1c and upregulate PPAR-α via AMPK.24 Interestingly, it is consistent with our results and in our previous studies, vitexin and its metabolism were detected in the blood which implied that they could be absorbed to play an active role.18 Also, 5-caffeoylquinic acid, another main ingredient in THL, has been confirmed to ameliorate lipid metabolism via activating PPAR-α which was consistent with the results of this paper.25 Actually, oxidative stress, lipid metabolism, and inflammation often occur together and exacerbate each other. Proinflammatory cytokines such as TNF-α and excessive reactive oxygen species (ROS) aggravate hepatic lipid accumulation.26,27 Thus, conversely, the positive effects of THL on these pathological conditions were also mutually influenced. The excellent anti-oxidative ability of THL extracts was obvious in this experiment, which may lie not only in the ability of the flavonoids in THL to bind free radicals but also in participating in some oxidative pathways. As expected, THL contributes to the production of antioxidant-related enzymes. This may be due to THL reducing the binding of Keap1 to Nrf2 and promoting Nrf2/ARE binding in the nucleus, resulting in the production of downstream antioxidant enzymes such as HO-1 and NQO1. Of course, research has shown that the activation of Nrf2 also relieved hepatic lipid metabolism and inflammation.28,29 Therefore, it is possible that THL may also mediate Nrf2 in other ways to interfere with NAFLD.
The gut–liver axis is a crucial target for NAFLD treatment and the gut microbiota community plays a key role. The portal circulation is the basis of the gut–liver crosstalk and the intestinal barrier is the first protective barrier of the liver. However, due to the complexity of the gut–liver axis, we only specify it as “gut barrier integrity” and “gut microbiota” in this paper. Herein, we are concerned about “gut barrier integrity” and “gut microbiota” in the gut–liver axis. Some parameters such as TBA and LPS in serum and intestinal tight junction proteins provide evidence that THL maintains intestinal barrier integrity. The accumulation of MCP-1 tends to be in high expression after stimulation by inflammatory cytokines such as TNF-α. Therefore, MCP-1 also could indicate the occurrence of inflammation. In addition, the continuous accumulation of MCP-1 promotes the development of steatosis, inflammation, and liver fibrosis,30 and thus it can serve as a major indicator for the development of colitis and NAFLD.31,32
It is now generally accepted that the interaction between polyphenols and microbiota is mutual. On the one hand, polyphenols are modified or degraded into metabolites that can be better absorbed and utilized. Moreover, studies have shown that these metabolites tend to have stronger activities and have a “prebiotic-like” effect. On the other hand, polyphenols modulate the composition of the gut microbiota.33,34 In addition, other metabolites of microorganisms such as SCFAs are important for maintaining intestinal homeostasis and beneficial for epithelial barrier function. Higher levels of SCFAs have positive effects on gut health and mitigation of NAFLD including maintenance of the gut barrier and improvement in hepatic steatosis and inflammation.35,36 Therefore, it is likely that the promotion of SCFA production by THL is another aspect of improving NAFLD. Butyrate promotes the epithelial barrier function, being the main energy source of the colon.37 This is also consistent with our results that THL extracts promoted the production of acetic acid, propionic acid, and butyric acid, and the gut barrier function was protected in the THL extract group. It may be attributed to the regulation of gut microbiota by THL extracts because the microbiota composition had changed and diversity levels increased after THL extract intervention. Specifically as the dominant bacteria in the THL group, Akkermansia has been reported to ameliorate NAFLD such as oxidative stress in the liver, and improve intestinal barrier integrity, which is consistent with our correlation analysis results.38,39 In Lefse analysis, many probiotics such as Ruminococcaceae, Lactobacillales, and Bifidobacteriales were enriched in the THL extract group. Ruminococcaceae family, a butyrate producer, was decreased in patients with NAFLD while it was enriched in the THL extract group.40,41Lactobacillales is conducive to the treatment of IBD for its anti-inflammatory effects. However, recent studies have indicated that Lactobacillales attenuates NAFLD via reducing cholesterol and steatosis.42 Interestingly, “the cross-feeding” was found in these bacteria, which refers to the bacteria feeding on each other. For instance, the lactate produced by Lactobacillales can stimulate butyrate-producing bacteria to produce more butyrate.43 Similar phenomena were also found in Bifidobacterium and butyrate-producing bacteria.44 Moreover, Blautia, a member of the Lachnospiraceae family, has potential to be a beneficial bacteria with palliative effects in inflammatory and metabolic diseases.45,46 Accordingly, there was a significantly increased abundance of these three bacteria in the THL extract group, compared to the HFD + DSS group. Therefore, Lactobacillales, Ruminococcaceae, and Bifidobacteriales may play an important role in the effect of THL extracts. Regarding inflammation and oxidative stress, THL extracts had manifested beneficial effects in our previous study.19 In summary, we inferred that the effect of THL extracts on NAFLD may be related to the restoration of gut microbiota dysbiosis.
Above all, the experiment also demonstrated that the effect of THL extracts on intestinal injury was conducive to the remission of NAFLD. It is an important target of crosstalk in the gut–liver axis. According to the results of this study, THL extracts can relieve NAFLD-related “multiple hit” manifestation. And these effects were direct or indirect, through maintaining intestinal homeostasis to reduce the damage to the liver from intestinal harmful substances or promoting the production of beneficial bacteria to help some beneficial metabolites to reduce liver damage. The research preliminarily proved that THL can improve NAFLD as gut barrier integrity and gut microbiota regulated by THL extracts played an important part. However, further exploration is needed to clarify the mechanism of how THL improves NAFLD, which is also our next goal.
5. Conclusion
Collectively, these findings indicated that THL extracts alleviated NAFLD-related oxidative stress, hepatic lipid accumulation, and inflammation in NAFLD mice with low-grade colitis. It is noteworthy that the anti-NAFLD effects of THL extracts may be related to the gut–liver axis which includes “protection of gut barrier integrity” and “regulation of gut microbiota”. To be specific, THL extracts reduced hepatic lipid synthesis and promoted lipid oxidation by regulating SREBP-1c, ACC-1, PPAR-α, and PPAR-γ genes. The effect of THL extracts on the gut includes protection of the intestinal epithelial barrier and a boost of beneficial bacteria which is beneficial for improving NAFLD. As was discussed above, the impact of the “multiple hits” was multifarious and the effect of THL extracts may be by multiple pathways. Both low- and high-dose THL extracts had a certain impact on NAFLD, although we could not determine whether it was dose-dependent. The overall mechanism underlying THL action on NAFLD is summarized in Fig. 6. More detailed experiments are needed to determine whether there is a dose dependence and what is the specific targeting molecular pathway. This study suggested for the first time that THL has the potential to intervene in NAFLD exacerbations via the gut–liver axis. However, the more specific mechanism needs to be further explored, which will be our next goal.
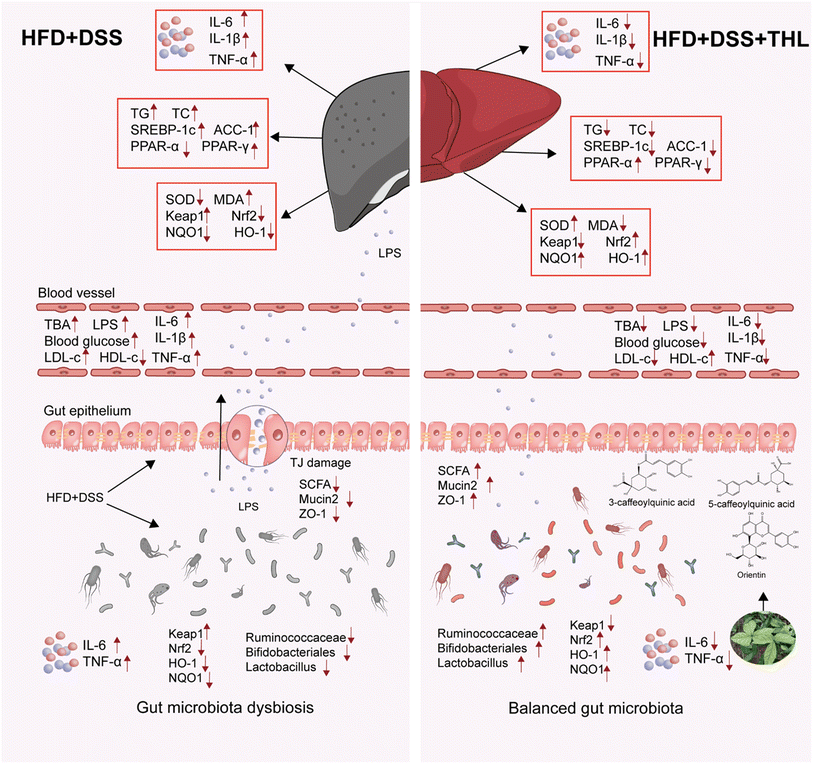 |
| Fig. 6 A conclusive diagram about THL extracts ameliorating HFD and DSS-induced NAFLD with colitis. The left of this diagram represents the status of gut microbiota, inflammation, lipid metabolism, and oxidative stress in the gut and liver under HFD and DSS stimulation. HFD and DSS generated hepatic lipid accumulation, inflammation, oxidative stress, and damaged epithelial barrier, dysbiosis of gut microbiota, and inflammation in the gut. The right hand side of this diagram represents the alteration of gut microbiota, inflammation, lipid metabolism, and oxidative stress in the gut and liver under THL extract treatment. After THL extract intervention, the inflammation, lipid metabolism, and oxidative stress were alleviative. THL extract increased the abundance of some beneficial bacteria such as Ruminococcaceae, Lactobacillus, and Bifidobacteriales. | |
Abbreviations
THL |
Tetrastigma hemsleyanum leaves |
NAFLD | Nonalcoholic fatty liver disease |
NASH | Non-alcoholic steatohepatitis |
HCC | Hepatocellular carcinoma |
IBD | Inflammatory bowel diseases |
TJ | Tight junction |
LPS | Lipopolysaccharide |
BAs | Bile acids |
TLR | Toll-like receptor |
HFD | High fat diet |
DSS | Dextran sulfate sodium salt |
MDA | Malondialdehyde |
SOD | Superoxide dismutase |
TG | Total triglycerides |
TC | Total cholesterol |
HDL-c | High-density lipoprotein cholesterol |
LDL-c | Low-density lipoprotein cholesterol |
TBA | Total bile acid |
AST | Aspartate aminotransferase |
ALT | Alanine aminotransferase |
IL-1β | Interleukin-1β |
IL-6 | Interleukin-6 |
TNF-α | Mice tumor necrosis factor-α |
RT-QPCR | Real time quantitative PCR |
AUC | Area under the curve |
H&E | Hematoxylin and eosin |
OGTT | Oral glucose tolerance |
MCP-1 | Monocyte chemoattractant protein |
HFD + DSS | High fat diet + DSS |
L-THL | Low dose group |
H-THL | High dose group |
ELISA | Enzyme linked immunosorbent assay |
PPARα/γ | Peroxisome proliferator-activated receptor alpha/gamma |
ACC-1 | Acetyl-CoA carboxylase-1 |
SREBP-1c | Sterol response element-binding protein-1c |
Keap1 | Kelch ECH associating protein 1 |
Nrf2 | Nuclear factor erythroid 2-related factor 2 |
SCFAs | Short chain fatty acids |
PCoA | Principal coordinates analysis |
NMDS | Non-metric multidimensional scaling |
LDA | Linear regression analysis |
AMP | Adenosine 5′-monophosphate |
AMPK | Adenosine 5′-monophosphate-activated protein kinase |
Author contributions
Lihua Xiao: conceptualization, investigation, methodology, validation, software, formal analysis, writing – original draft. Hua Xiong: conceptualization, funding acquisition. Zeyuan Deng: validation, resources. Xin Peng: validation, supervision. Kejun Cheng: validation. Hua Zhang: writing – review and editing, validation, resources. Li Jiang: conceptualization, investigation. Yong Sun: supervision, project administration, funding acquisition.
Conflicts of interest
The authors declare that they have no known competing financial interests or personal relationships that could have appeared to influence the work reported in this paper.
Acknowledgements
This study was supported by the National Natural Science Foundation of China (No. 82060781), the China Postdoctoral Science Foundation (No. 2020M671975) and Key R & D project of Jiangxi Provincial Department of Science and Technology (20212BBF63033).
References
- J. V. Lazarus, H. E. Mark, Q. M. Anstee, J. P. Arab, R. L. Batterham, L. Castera, H. Cortez-Pinto, J. Crespo, K. Cusi and M. A. Dirac, Advancing the global public health agenda for NAFLD: a consensus statement, Nat. Rev. Gastroenterol. Hepatol., 2021, 19, 60–78 CrossRef PubMed.
- Z. M. Younossi, A. B. Koenig, D. Abdelatif, Y. Fazel, L. Henry and M. Wymer, Global epidemiology of nonalcoholic fatty liver disease—meta–analytic assessment of prevalence, incidence, and outcomes, Hepatology, 2016, 64, 73–84 CrossRef PubMed.
- A. C. Sheka, O. Adeyi, J. Thompson, B. Hameed, P. A. Crawford and S. Ikramuddin, Nonalcoholic steatohepatitis: a review, J. Am. Med. Assoc., 2020, 323, 1175–1183 CrossRef CAS PubMed.
- Y.-L. Fang, H. Chen, C.-L. Wang and L. Liang, Pathogenesis of non-alcoholic fatty liver disease in children and adolescence: From “two hit theory” to “multiple hit model”, World J. Gastroenterol., 2018, 24, 2974 CrossRef CAS PubMed.
- J. K. Dowman, J. Tomlinson and P. Newsome, Pathogenesis of non-alcoholic fatty liver disease, QJM, 2010, 103, 71–83 CrossRef CAS PubMed.
- J. B. Schwimmer, C. Behling, R. Newbury, R. Deutsch, C. Nievergelt, N. J. Schork and J. E. Lavine, Histopathology of pediatric nonalcoholic fatty liver disease, Hepatology, 2005, 42, 641–649 CrossRef PubMed.
- V. Nobili, N. Alkhouri, A. Alisi, C. Della Corte, E. Fitzpatrick, M. Raponi and A. Dhawan, Nonalcoholic fatty liver disease: a challenge for pediatricians, JAMA Pediatr., 2015, 169, 170–176 CrossRef PubMed.
- A. Lin, H. Roth, A. Anyane-Yeboa, D. T. Rubin and S. Paul, Prevalence of nonalcoholic fatty liver disease in patients with inflammatory bowel disease: a systematic review and meta-analysis, Inflamm. Bowel Dis., 2021, 27, 947–955 CrossRef PubMed.
- L. Miele, V. Valenza, G. La Torre, M. Montalto, G. Cammarota, R. Ricci, R. Masciana, A. Forgione, M. L. Gabrieli and G. Perotti, Increased intestinal permeability and tight junction alterations in nonalcoholic fatty liver disease, Hepatology, 2009, 49, 1877–1887 CrossRef CAS PubMed.
- S. Citi, Intestinal barriers protect against disease, Science, 2018, 359, 1097–1098 CrossRef CAS PubMed.
- T. Csak, M. Ganz, J. Pespisa, K. Kodys, A. Dolganiuc and G. Szabo, Fatty acid and endotoxin activate inflammasomes in mouse hepatocytes that release danger signals to stimulate immune cells, Hepatology, 2011, 54, 133–144 CrossRef CAS PubMed.
- A. Sartini, S. Gitto, M. Bianchini, M. C. Verga, M. Di Girolamo, A. Bertani, M. Del Buono, F. Schepis, B. Lei and N. De Maria, Non-alcoholic fatty liver disease phenotypes in patients with inflammatory bowel disease, Cell Death Dis., 2018, 9, 1–8 CrossRef CAS PubMed.
- E. Gäbele, K. Dostert, C. Hofmann, R. Wiest, J. Schölmerich, C. Hellerbrand and F. Obermeier, DSS induced colitis increases portal LPS levels and enhances hepatic inflammation and fibrogenesis in experimental NASH, J. Hepatol., 2011, 55, 1391–1399 CrossRef PubMed.
- C. Cheng, J. Tan, W. Qian, L. Zhang and X. Hou, Gut inflammation exacerbates hepatic injury in the high-fat diet induced NAFLD mouse: Attention to the gut-vascular barrier dysfunction, Life Sci., 2018, 209, 157–166 CrossRef CAS PubMed.
- W. Hu, Y. Zheng, P. Xia and Z. Liang, The research progresses and future prospects of Tetrastigma hemsleyanum Diels et Gilg: A valuable Chinese herbal medicine, J. Ethnopharmacol., 2021, 271, 113836 CrossRef CAS PubMed.
- Y. Sun, H. Li, J. Hu, J. Li, Y. W. Fan, X. R. Liu and Z. Y. Deng, Qualitative and quantitative analysis of phenolics in Tetrastigma hemsleyanum and their antioxidant and antiproliferative activities, J. Agric. Food Chem., 2013, 61, 10507–10515 CrossRef CAS PubMed.
- Y. Sun, Q. Hui, R. Chen, H. Li, H. Peng, F. Chen and Z. Deng, Apoptosis in human hepatoma HepG2 cells induced by the phenolics of Tetrastigma hemsleyanum leaves and their antitumor effects in H22 tumor-bearing mice, J. Funct. Foods, 2018, 40, 349–364 CrossRef CAS.
- Y. Sun, R. Tsao, F. Chen, H. Li, J. Wang, H. Peng, K. Zhang and Z. Deng, The phytochemical composition, metabolites, bioavailability and in vivo antioxidant activity of Tetrastigma hemsleyanum leaves in rats, J. Funct. Foods, 2017, 30, 179–193 CrossRef CAS.
- T. Wu, X. Wang, H. Xiong, Z. Deng, X. Peng, L. Xiao, L. Jiang and Y. Sun, Bioactives and their metabolites from Tetrastigma hemsleyanum leaves ameliorate DSS-induced colitis via protecting the intestinal barrier, mitigating oxidative stress and regulating the gut microbiota, Food Funct., 2021, 12, 11760–11776 RSC.
- W. Ji, X. Peng, T. Lou, J. Wang and W. Qiu, Total flavonoids from Tetrastigma hemsleyanum ameliorates inflammatory stress in concanavalin A-induced autoimmune hepatitis mice by regulating Treg/Th17 immune homeostasis, Inflammopharmacology, 2019, 27, 1297–1307 CrossRef CAS PubMed.
- C. Rosso, L. Mezzabotta, M. Gaggini, F. Salomone, R. Gambino, A. Marengo, F. Saba, E. Vanni, R. Younes and C. Saponaro, Peripheral insulin resistance predicts liver damage in nondiabetic subjects with nonalcoholic fatty liver disease, Hepatology, 2016, 63, 107–116 CrossRef CAS PubMed.
- S.-Y. Cai, X. Ouyang, Y. Chen, C. J. Soroka, J. Wang, A. Mennone, Y. Wang, W. Z. Mehal, D. Jain and J. L. Boyer, Bile acids initiate cholestatic liver injury by triggering a hepatocyte-specific inflammatory response, JCI Insight, 2017, 2, e90780 CrossRef PubMed.
- X. Chen, C. Zhang, M. Zhao, C. E. Shi, R. M. Zhu, H. Wang, H. Zhao, W. Wei, J. B. Li and D. X. Xu, Melatonin alleviates lipopolysaccharide–induced hepatic SREBP–1c activation and lipid accumulation in mice, J. Pineal Res., 2011, 51, 416–425 CrossRef CAS PubMed.
- S. Inamdar, A. Joshi, S. Malik, R. Boppana and S. Ghaskadbi, Vitexin alleviates non-alcoholic fatty liver disease by activating AMPK in high fat diet fed mice, Biochem. Biophys. Res. Commun., 2019, 519, 106–112 CrossRef CAS PubMed.
- Y.-J. Lee, Y.-N. Jang, Y.-M. Han, H.-M. Kim, J.-M. Jeong, M. J. Son, C. B. Jin, H. J. Kim and H. S. Seo, Caffeoylquinic acid-rich extract of Aster glehni F. Schmidt ameliorates nonalcoholic fatty liver through the regulation of PPARδ and adiponectin in ApoE KO mice, PPAR Res., 2017, 2017, 3912567 Search PubMed.
- R. Gambino, G. Musso and M. Cassader, Redox balance in the pathogenesis of nonalcoholic fatty liver disease: mechanisms and therapeutic opportunities, Antioxid. Redox Signaling, 2011, 15, 1325–1365 CrossRef CAS PubMed.
- M. Endo, T. Masaki, M. Seike and H. Yoshimatsu, TNF-α induces hepatic steatosis in mice by enhancing gene expression of sterol regulatory element binding protein-1c (SREBP-1c), Exp. Biol. Med., 2007, 232, 614–621 CAS.
- S. S. Chambel, A. Santos-Gonçalves and T. L. Duarte, The dual role of Nrf2 in nonalcoholic fatty liver disease: regulation of antioxidant defenses and hepatic lipid metabolism, BioMed Res. Int., 2015, 2015, 597134 Search PubMed.
- L. Li, J. Fu, J. Sun, D. Liu, C. Chen, H. Wang, Y. Hou, Y. Xu and J. Pi, Is Nrf2-ARE a potential target in NAFLD mitigation?, Curr. Opin. Toxicol., 2019, 13, 35–44 CrossRef.
- C. Baeck, X. Wei, M. Bartneck, V. Fech, F. Heymann, N. Gassler, K. Hittatiya, D. Eulberg, T. Luedde and C. Trautwein, Pharmacological inhibition of the chemokine C–C motif chemokine ligand 2 (monocyte chemoattractant protein 1) accelerates liver fibrosis regression by suppressing Ly–6C + macrophage infiltration in mice, Hepatology, 2014, 59, 1060–1072 CrossRef CAS PubMed.
- W. I. Khan, Y. Motomura, H. Wang, R. T. El-Sharkawy, E. F. Verdu, M. Verma-Gandhu, B. J. Rollins and S. M. Collins, Critical role of MCP-1 in the pathogenesis of experimental colitis in the context of immune and enterochromaffin cells, Am. J. Physiol.: Gastrointest. Liver Physiol., 2006, 291, G803–G811 CrossRef CAS PubMed.
- J. W. Haukeland, J. K. Damås, Z. Konopski, E. M. Løberg, T. Haaland, I. Goverud, P. A. Torjesen, K. Birkeland, K. Bjøro and P. Aukrust, Systemic inflammation in nonalcoholic fatty liver disease is characterized by elevated levels of CCL2, J. Hepatol., 2006, 44, 1167–1174 CrossRef CAS PubMed.
- J. C. Espín, A. González-Sarrías and F. A. Tomás-Barberán, The gut microbiota: A key factor in the therapeutic effects of (poly) phenols, Biochem. Pharmacol., 2017, 139, 82–93 CrossRef PubMed.
- N. Zhou, X. Gu, T. Zhuang, Y. Xu, L. Yang and M. Zhou, Gut microbiota: a pivotal hub for polyphenols as antidepressants, J. Agric. Food Chem., 2020, 68, 6007–6020 CrossRef CAS PubMed.
- M. Deng, F. Qu, L. Chen, C. Liu, M. Zhang, F. Ren, H. Guo, H. Zhang, S. Ge and C. Wu, SCFAs alleviated steatosis and inflammation in mice with NASH induced by MCD, J. Endocrinol., 2020, 245, 425–437 CAS.
- Y. Feng, Y. Wang, P. Wang, Y. Huang and F. Wang, Short-chain fatty acids manifest stimulative and protective effects on intestinal barrier function through the inhibition of NLRP3 inflammasome and autophagy, Cell. Physiol. Biochem., 2018, 49, 190–205 CrossRef CAS PubMed.
- D. Parada Venegas, M. K. De la Fuente, G. Landskron, M. J. González, R. Quera, G. Dijkstra, H. J. Harmsen, K. N. Faber and M. A. Hermoso, Short chain fatty acids (SCFAs)-mediated gut epithelial and immune regulation and its relevance for inflammatory bowel diseases, Front. Immunol., 2019, 10, 277 CrossRef PubMed.
- Y. Rao, Z. Kuang, C. Li, S. Guo, Y. Xu, D. Zhao, Y. Hu, B. Song, Z. Jiang and Z. Ge, Gut Akkermansia muciniphila ameliorates metabolic dysfunction-associated fatty liver disease by regulating the metabolism of L-aspartate via gut-liver axis, Gut Microbes, 2021, 13, 1927633 CrossRef PubMed.
- M. Juárez-Fernández, D. Porras, P. Petrov, S. Román-Sagüillo, M. V. García-Mediavilla, P. Soluyanova, S. Martínez-Flórez, J. González-Gallego, E. Nistal and R. Jover, The Synbiotic Combination of Akkermansia muciniphila and Quercetin Ameliorates Early Obesity and NAFLD through Gut Microbiota Reshaping and Bile Acid Metabolism Modulation, Antioxidants, 2021, 10, 2001 CrossRef PubMed.
- W. Tang, X. Yao, F. Xia, M. Yang, Z. Chen, B. Zhou and Q. Liu, Modulation of the gut microbiota in rats by Hugan Qingzhi tablets during the treatment of high-fat-diet-induced nonalcoholic fatty liver disease, Oxid. Med. Cell. Longevity, 2018, 2018, 7261619 Search PubMed.
- M. Goffredo, K. Mass, E. J. Parks, D. A. Wagner, E. A. McClure, J. Graf, M. Savoye, B. Pierpont, G. Cline and N. Santoro, Role of gut microbiota and short chain fatty acids in modulating energy harvest and fat partitioning in youth, J. Clin. Endocrinol. Metab., 2016, 101, 4367–4376 CrossRef CAS PubMed.
- N. Y. Lee, M. J. Shin, G. S. Youn, S. J. Yoon, Y. R. Choi, H. S. Kim, H. Gupta, S. H. Han, B. K. Kim and D. Y. Lee, Lactobacillus attenuates progression of nonalcoholic fatty liver disease by lowering cholesterol and steatosis, Clin. Mol. Hepatol., 2021, 27, 110 CrossRef PubMed.
- Q. P. Da Zhou, F.-Z. Xin, R.-N. Zhang, C.-X. He, G.-Y. Chen, C. Liu, Y.-W. Chen and J.-G. Fan, Sodium butyrate attenuates high-fat diet-induced steatohepatitis in mice by improving gut microbiota and gastrointestinal barrier, World J. Gastroenterol., 2017, 23, 60 CrossRef PubMed.
- A. Rivière, M. Selak, D. Lantin, F. Leroy and L. De Vuyst, Bifidobacteria and butyrate-producing colon bacteria: importance and strategies for their stimulation in the human gut, Front. Microbiol., 2016, 7, 979 Search PubMed.
- X. Liu, B. Mao, J. Gu, J. Wu, S. Cui, G. Wang, J. Zhao, H. Zhang and W. Chen, Blautia—a new functional genus with potential probiotic properties?, Gut Microbes, 2021, 13, 1875796 CrossRef PubMed.
- A. Thong-On, K. Suzuki, S. Noda, J.-i. Inoue, S. Kajiwara and M. Ohkuma, Isolation and characterization of anaerobic bacteria for symbiotic recycling of uric acid nitrogen in the gut of various termites, Microbes Environ., 2012, 27, 186–192 CrossRef PubMed.
|
This journal is © The Royal Society of Chemistry 2023 |
Click here to see how this site uses Cookies. View our privacy policy here.