Water chestnut starch: extraction, chemical composition, properties, modifications, and application concerns
Received
11th November 2022
, Accepted 6th January 2023
First published on 1st March 2023
Abstract
The increase in non-biodegradable waste has prompted research on the development of new biodegradable compounds that can act as substitutes for the prevalent synthetic materials used for packaging. In this regard, due to the advantages of starch including its biodegradable, abundant, cost effective, and non-texture nature, it has attracted attention in the food and pharmaceutical industries. Presently, numerous studies have been reported on the search for new sources of starch. Herein, we summarize research on water chestnut as a non-conventional source of starch. Advantageously, due to low protein, ash and lipid content in water chestnut, its processing requires little effort and a large pure starch fraction can be extracted. The large amount of carbohydrates in water chestnut makes it a tremendous reservoir of starch for the food and non-food industries and it can replace other commercial sources of starchy such as wheat and potato. The starch in water chestnut can be extracted using a series of methods including centrifugation, and subsequently, its purification results in shapes ranging from elliptical and globular to prismatoid. However, the native form of starch is not stable with variations in temperature and pH, which restricts its use in specific applications. Thus, native starches are commonly altered to achieve improved characteristics such as solubility, swelling, texture, and thermal tolerance. Starch from water chestnut is known to show potential as a pharmaceutical excipient and also a potent candidate for the prevention of type II diabetes and obesity. This review highlights the physiochemical properties, modifications and applications of water chestnut starch in the food and non-food industries.
1. Introduction
Water chestnut (WC) is a floating leaved annual, aquatic herb found in freshwater wetlands, ponds, and lakes.1 According to its geographical distribution, higher rainfed areas contribute to its successful cultivation.2 Primarily, it is considered a vegetable and used in the preparation of human food including sweet dishes and baked stuff as dry flour. Furthermore, it possesses a wide range of pharmacological activities including microbicidal, anti-inflammatory, pain killer, and anti-diabetic activities.3–6 Water chestnut has several bioactive constituents including cysteine, lectin, quercetin, proteinase inhibitor, fibers, vitamins, fatty acids, and minerals. The main constituent of this vegetable is starch (85–97.4% dry basis), which signifies its potential as a healthy food.7,8 Moreover, the starch industry is continuously searching for novel sources to counteract production costs and also meet the increasing demand for new starches in food with pharma applications. Currently, the demand for starch has increased tremendously, which is widely employed for the production of ethanol and biodegradable plastics, besides its use in the food processing industry.9 In this case, water chestnuts are suitable candidates to supply a large quantity of starch given that they are widely available and economic. Furthermore, water chestnut starch has a unique characteristic compared to other sources of starch, i.e., potato and rice. Specifically, water chestnut can maintain a brittle and crunchy texture after heat treatment during cooking or canning.10 According to its geographical allocation, Chinese water chestnut starch shows variable morphological attributes ranging from round to croissant-shaped such as irregular and triangular;11 however, starches extracted from Indian chestnuts were observed to be irregular, oval, and cubic.10 Basically, starch is employed as a thickener, bulking, gelling, stabilizer, and water-retaining agent. Currently, most food processing industries utilize native and modified starches to give proprietary organoleptic properties to the finished product. Furthermore, presently modified food starches are considered critical and beneficial functional food additives.12 The functional role of starch in several foods is based on its physicochemical characteristics.13–15 Presently, the food processing industry is focused on finding starch that can tolerate the extreme heat and shear conditions associated with processing. This is because native starches cannot be employed alone due to their many limitations such as low paste clarity, low shear stress resistance, and high retrogradation extent. Alternatively, the modification of starch by physical or chemical means or both enhances its native functional characteristics, thus widening its application.16 Furthermore, because native starches exhibit some limitation, it is necessary to alter them to match the requirements of the food industry. In the case of physical modification, mainly gelatinization or simple chemical modification is employed to meet the specific requirements of food and other allied industries. Modified food starches usually exhibit efficient pasting properties, stability, enhanced obstruction to retrogradation, and freeze-thaw stability. Acid modification is broadly employed in the starch industry to design thin-boiling starches for use in food, paper, textile, and other industries.17 Another major use of modified starches in regards to human health is as a fat replacer, which provides a gross range of benefits to product developers and also healthy status to consumers by reducing the proportion of fat. Thus, water chestnut starch is a potential substitute for traditional starch, which is increasingly in demand by the food sector to meet the increasing needs of the global population and utilized in a variety of ways. In this case, the various features of water chestnut starch, including its extraction, chemical composition, physicochemical qualities, modification, and applications in food items, need to be summarized to complement the knowledge that is currently available.18
2. Chemical composition of water chestnut starch
The chemical composition of starch extracted from water chestnut is presented in Table 1. Starch extracted from water chestnut has 0.2% w/w protein, ash (0.2% w/w) and lipids (0.1% w/w), therefore containing 99.5% w/w pure starch.19 The amount of amylose in starch isolated from water chestnut is found to be in the range of 21.8 to 32.10 g per 100 g.20–22 The water chestnut starch from the Dal Lake showed a lower protein (0.11% w/w) and ash (0.006% w/w) content compared to that from the Wular and Anchar Lakes, depicting its high purity.14 Alternatively, the amylose content was observed to be greater in the water chestnut starch from the Anchar Lake (30.5% w/w), followed by that from Wular Lake (29.6% w/w) and Dal Lake (28.5% w/w). These differences in chemical composition may be due to the agro-climatic variations in different areas.7
Table 1 Chemical composition of different starchesa
S. no. |
Type of starch |
Protein (%) |
Ash (%) |
Lipid (%) |
Amylose (g per 100 g) |
References |
NR: Not reported.
|
1 |
Chinese water chestnut starch |
0.39 |
0.49 |
0.86 |
32.10 |
21
|
2 |
Native water chestnut starch |
0.1 |
0.17 |
0.19 |
29 |
22–24
|
3 |
Native water chestnut starch |
0.2 |
0.2 |
0.1 |
22.3 |
19
|
4 |
Native water chestnut starch |
0.21 |
0.04 |
NR |
NR |
25
|
5 |
Native water chestnut starch |
0.2 |
0.1 |
0.2 |
21.8 |
26
|
6 |
Native water chestnut starch |
NR |
0.69 |
NR |
26.78 |
27
|
3. Isolation of starch from water chestnut
Water chestnut fruit was peeled, washed, and blended with 0.2% w/w solution of NaOH. Firstly, the homogenate was sieved using a 100 μm mesh sieve, and then filtered through 170 μm mesh sieve followed by centrifugation. The upper brown-colored layer of protein was removed mechanically and the residue was washed several times with distilled water to remove the last traces of the brown layer. The extracted starch was washed twice with anhydrous ethanol, dried, and stored at −20 °C for further analysis. In another study, toluene was used for the removal of the protein layer to obtain pure starch22,28–31 (Fig. 1).
 |
| Fig. 1 Isolation of starch. | |
4. Properties of water chestnut starch
4.1. Thermal properties
Starch granules are practically insoluble in cold water, but they swell due to the absorption of water on heating in the presence of a sufficient amount of water. Consequently, the birefringence is lost due to the absorption of water in the amorphous regions of the starch granules, disrupting their crystalline structure. Gelatinization and the associated properties of starch can be well established by several methods, including electron microscopy, optical microscopy, X-ray diffraction, differential scanning calorimetry, Fourier transform infrared spectroscopy, and nuclear magnetic resonance spectroscopy.32,33 A researcher studied the gelatinization behavior of starch granules together with the disruption of their crystalline regions followed by their swelling using a polarising microscope combined with a hot stage. The distribution of amylose in the native starch granules was also studied via confocal laser scanning microscopy (CLSM) to explain the gelatinization process of starch granules. Specifically, 12 different starches were isolated from sweet potato tuberous root, lotus and yam rhizomes, potato tuber, water chestnut, and pea, barley, bean, wheat, lotus, and ginkgo seeds. Subsequently, four different patterns of gelatinization were observed. The gelatinization pattern in the water chestnut starch indicated that the disruption in its crystallinity started from the central hilum area, which then propagates along the central part and causes a small amount of swelling. With an increase in temperature, the crystallinity disruption continues and swelling of the starch granules occurs from the proximal to distal regions30 (Table 2).
Table 2 Thermal properties of water chestnut starch
S. no. |
Method of investigation |
T
o (°C) |
T
p (°C) |
T
c (°C) |
ΔHgel (J g−1) |
Reference |
1 |
DSC |
58.54 |
64.13 |
68.43 |
10.77 |
38
|
2 |
DSC |
58.00 |
70.40 |
82.8 |
13.22 |
35
|
3 |
DSC |
49.80 |
57.90 |
68.70 |
8.90 |
34
|
4 |
DSC |
69.60 |
73.30 |
75.20 |
7.30 |
10
|
5 |
DSC |
70.53 |
79.64 |
84.65 |
12.9 |
27
|
6 |
DSC |
66.77 |
71.99 |
79.84 |
12.04 |
26
|
The thermal properties of water chestnut starch were identified by Xu and Shoemaker,35 indicating an onset temperature (To) of 59.0 °C, peak temperature (Tp) of 70.4 °C, conclusion temperature (Tc) of 82.8 °C, and gelatinization enthalpy (ΔHgel) of 3.16 cal g−1. In another study,34 the thermal properties of C-type starches of fava bean, water chestnut, pea, and yam were investigated using differential scanning calorimetry (DSC). A much lower gelatinization temperature (49.8 °C) and enthalpy of gelatinization (8.9 J g−1) were reported for water chestnut starch in comparison to the previously reported values by Xu and Shoemaker,35 indicating a lower amount of energy is needed to initiate gelatinization. Also, much higher values of To (58.5 °C), Tp (64.1 °C), Tc (68.4 °C) and ΔHgel (10.8 J g−1) were reported for WCS. In general, the thermal parameters of starch are dependent on its (i) granular ultrastructure, (ii) size, (iii) amylopectin branch chain, (iv) amylose content, (v) damaged starch content, (vi) phosphorus content and (vii) crystalline structure. Moreover, the presence of a large number of amylopectin short chains in water chestnut starch results in inefficient packing, and thereby a low gelatinization temperature and enthalpy.36 Additionally, water chestnut starch contains a large proportion of damaged starch, and thus its ordered crystalline structure is converted into a random amorphous structure, which is easily accessible to water.37 Among the four starches investigated by Cai et al.,34 water chestnut starch was associated with the lowest degree of structural order and amylose content, smallest granule size, largest damaged starch content, and highest amylopectin short-chain content, which are responsible for its lower gelatinization temperature and enthalpy. Singh et al.10 described the thermal properties of water chestnut starch and compared it with commercial maize and potato starch. The highest gelatinization temperature of 69.60 °C was observed in the case of water chestnut starch in comparison to 68.7 °C for corn and 59.9 °C for potato starch. The starch extracted from water chestnut demonstrated the maximum Tc of 75.20 °C compared to corn (74.4 °C) and potato (73.7 °C) starch. However, the enthalpy of gelatinization was minimum for water chestnut starch.
4.2. Morphological characteristics
Microscopic studies indicated that the starch granules of water chestnut are elliptical or globular in shape. In addition, a few prismatoid granules were also observed. The granules of starch extracted from the water chestnut displayed a consistent “Maltese cross” with the hilum in the middle of the granules. Moreover, a few impaired granules were also observed in the water chestnut starch. Further, scanning electron microscopy (SEM) was employed to detect the sub-microscopic contour and surface structure of starch granules. The contour of starch showed an identical morphology as that observed under a light microscope. It was observed that the exterior of the granules was plain with negligible orifices and slits. Consistent with the findings of light microscopy, a few weakened granules were found in the starch extracted from water chestnut.34 Singh et al.19 demonstrated the morphological characteristics of native, acid, and hydrothermally modified water chestnut starches through SEM. The starch remnants that existed in native water chestnut were found to be flat, ovoid to aberrant. The diameter of the granules varied from minute to average in the range of 5–30 mm. The acid-modified starch showed a negligible effect on the conformation of the granules because acid mainly destroys the amorphous domains, whereas has a minor impact on the crystal zone of the starch granules. However, 1 M HCl treatment demonstrated the generation of small furrows on the exterior of the starch granule. Contrary to this, treatment with HMT revealed fragmentation of the starch granules. It was found that the proportion of highly minute starch granules (5 mm) was enhanced from 2% in native starch to 10% in hydrothermally modified starch. Gani et al.7 described that the starch purified from water chestnut from Dal Lake was observed to have expanded ovoid granules in comparison to that from Anchar and Wular Lakes. The starch extracted from Dal Lake exhibited several huge-sized granules as compared to that from Anchar and Wular Lake. This revealed that the starch extracted from water chestnuts from the different lakes display several materializations of granules. Various conformations of starch granules impact their physicochemical properties such as gelatinization temperature, amylose content, and pasting properties. Similarly, Guo et al.39 demonstrated the morphological characteristics of starch of chestnut grown in various areas. It was observed that the starch from various regions displayed an analogous granule shape because of their identical botanic source, but varied in size. Most of the starch granules presented an ovoid to globular shape, with few ovoidal ones dispersed among the granules. Starch granules displayed plain exterior and their edges and did not show any furrows. In accordance with Koteswara Reddy et al.,40 the morphology of starch granules is associated with the physiological characteristics of their botanic origin such as amylose content, transmittance, swelling, and water absorption capacity. Lutfi et al.22 demonstrated also globular or ovoid-shaped granules with “horns” bulging from the surface of water chestnut starch. The acid-thinned and acetylated starches showed granules with an uneven surface. Furthermore, it was observed that acetylation of starch caused remarkable surface erosion in comparison to the acid-thinned starch, depicting the rigidness of acetylated treatment. Pregelatinized water chestnut starch upon bifold modification by acetylation showed granules with a more uneven and wrinkled surface. The acetylation of pregelatinized starch changes the inter-and intra-granular interplay among its granules, which may alter the microarchitecture of the starch and create numerous surface slits and orifices. Thus, SEM studies depict the greater effect of double modification on the morphological and microarchitecture of starch in comparison to one-step chemical modification.
4.3. Physico-chemical properties of starch
The fundamental physiochemical properties of purified chestnut starches including swelling power (SP), water retention capacity (WRC), solubility, pasting, freeze-thaw stability, light transmittance, and syneresis.
4.3.1. Swelling property, solubility and water retention capacity (WRC).
The swelling power of starch is a measure of the extent of the interactions present between the crystalline and amorphous regions of its chains. However, the swelling power of starch granules is primarily decided by their water-retention capability due to the formation of hydrogen bonds. During the gelatinization process, the hydrogen bonds that stabilize the double helices of the starch crystallites break, and new bonds are found among the exposed –OH groups of amylose, amylopectin, and water molecules. It has been reported that in comparison to intact granules, hydration of the impaired granules occurs smoothly, and therefore they possess greater swelling capacity. Besides the gelatinization temperature, granule swelling is based on the extent of impairment and the nature of starches. Amylose inhibits the swelling of starch and preserves the existence of swollen granules. Water chestnut starch exhibits excellent swelling power, which is ascribed to the presence of less amylose and increased quantity of impaired starch. In comparison to other starches from beans, yam starch, and pea, water chestnut starch demonstrates higher swelling power, as investigated by Cai et al.34 in the temperature range of 40 °C to 95 °C at 5 °C intervals. The water chestnut starch displayed an abrupt increment in swelling ability from 50 °C, whereas its swelling ability slightly increased with an increase in temperature before gelatinization. Lutfi et al.22 compared the swelling ability and solubility of water chestnut starch and its modified forms. Its swelling ability was found to vary linearly with an increase in temperature up to 50–90 °C. The acid modifications and pregelatinizing significantly decreased the swelling power of water chestnut starch in contrast to its native counterpart. Acid affects the amorphous domains first, and then the crystalline areas, attacking the surface of the granule first, and then its inner core. The increased roughness of the surface of starch due to acid thinning and the increase in crystalline domains may be the major cause for its restricted swelling. A significant increase in swelling power was observed in acetylated starch of water chestnut (aWCS) in comparison to native starch (WCS) mainly at 80 °C to 90 °C. Acetylated water chestnut starches (aWCS) demonstrated a remarkable increase in swelling power than their native counterparts due to the improved hydrophilicity of the starch granules. Yadav et al.26 reported that annealing and heat moisture treatment decreased the swelling power and solubility of starch granules. The decreased swelling power of modified starches indicate the presence of robust forces of attraction in the crystallite regions. During annealing, the amorphous amylose content is transformed into helical chains, resulting in an increase in attractive forces between the amylose helices, and consequently a decrease in swelling power. Similarly, an increase in moisture content makes the amorphous starch chains more mobile and enables stronger interactions between the crystalline regions of the starch. When water formulations of starch granules are heated, hydration and swelling of the starch molecules occur with the release of a few soluble starch granules into the liquid. The swelling ability of water chestnut starch was determined at 10 °C intervals over a wide temperature range of 50–90 °C. It was observed that the starch extracted from water chestnut from Anchar Lake revealed a lower swelling power of 1.4% to 11% w/w in comparison to that from Wular Lake (2.2% to 15% w/w). The maximum swelling ability of starch extracted from water chestnut was found to be 4.2–18.3% w/w from Dal Lake. Similarly, Dal Lake also displayed the greater solubility of starch (3.4% to 17% w/w) in comparison to the starch from Wular Lake (1.4–14.4% w/w), while the starch from Anchar Lake showed 0.4–10% w/w solubility over the temperature range of 50–90 °C. This revealed that the starch granules from water chestnut withstand swelling at temperatures less than 70 °C. When the temperature increased from 70 °C to 90 °C, the starch granules showed swelling due to the rupture of the hydrogen bonding in their amorphous regions, which displayed inevitable and dynamic water absorption.7 Similarly, an enhancement in the swelling of starch granules was observed in T. natans and T. bispinosa in a wide temperature range.20
The starch extracted from water chestnut from Dal, Wular and Anchar Lakes showed an increment in water binding capacity from 13% to 60% w/w, 9% to 52% w/w, and 7% to 44% w/w with an enhancement in temperature from 50 °C to 90 °C (Fig. 2 and 3), respectively.7 The lowest increment in the water binding capacity of starch from Anchar Lake can be ascribed to its greater number of –OH groups for the formation of hydrogen and covalent bonding among the starch chains in contrast to water.41 However, Bello-Pérez et al.42 demonstrated that starch isolated from water chestnut possessed a similar water-binding capacity. The WRC values of the water chestnut starch were found to be 0.8–2.5 (g g−1) in different areas. The water-binding sites were enhanced given that the number of –OH groups was greater with an increase in temperature. The greater water absorption capacity of starches can increase the functional applications of some food products, which may gain gradual water uptake and hinder the accumulation of lumps upon mixing with other powder forms of food.39
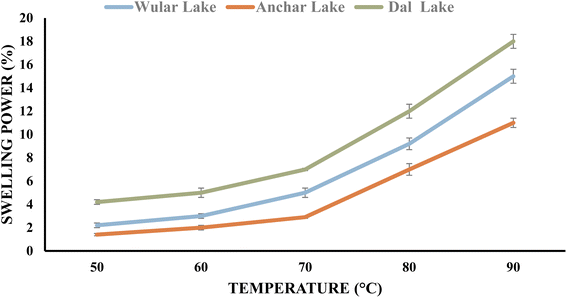 |
| Fig. 2 Swelling power of water chestnut starches at different temperatures.7 | |
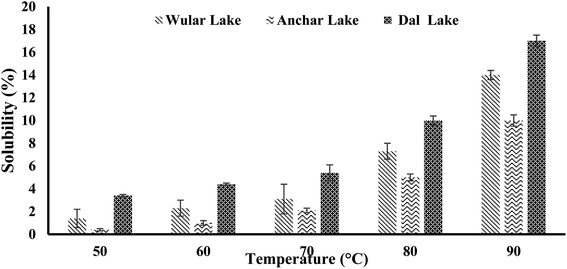 |
| Fig. 3 Solubility of water chestnut starches at different temperatures.7 | |
4.3.2. Syneresis.
Syneresis is the release of water from starch gel during its storage upon freezing. The rate of syneresis of chestnut starches was observed to be between 37.06% w/w (Fuzhou) and 56.40% w/w (Zhaotong).39 According to Hermansson and Svegmark,43 a greater amount of amylose causes greater interaction with the amylopectin chains, which leads to greater shrinkage of the gel and syneresis. It was found that a higher acid concentration leads to a reduction in the syneresis of starch gels.44 The decrease in the amylose content may be the reason for the decreased starch syneresis during gel formation. However, heat-moisture treatment boosts the syneresis of the to a lower degree in comparison to the native starch. This can be ascribed to the reduced water absorption of the starch granules during the course of their disintegration and casehardening of the granules during HMT. Lutfi et al.45 analysed the effect of salts on the syneresis of water chestnut starch and WCS-XG (xanthan gum) complexes. The proportion of syneresis for the native WCS was observed to be 38% w/w in comparison to that of corn, sweet potato starch, and mung bean starch, which was found to decrease.46,47 It was observed that the syneresis value of WCS was enhanced after treatment with a higher concentration of NaCl at a lower temperature. The mode of action behind this may be due to the rearrangement of the starch molecules or enhanced molecular interactions among the starch molecules at a lower temperature, leading to the release of water from the starch. The syneresis value of these starch mixtures was observed to be low in the absence of sodium chloride, according to the results reported by.48,49
4.3.3. Light transmittance.
Light transmittance is the quantity of light passing through starch paste, which gives important data about its behaviour. Many factors influence the transmittance including granule size, percentage of amylose, swelling power, amylose/amylopectin ratio, and proportion of swollen and non-swollen granule remnants. The transmittance values of WCS alone and WCS-XG complexes with and without the addition of NaCl were studied.45 It was found that the transmittance was enhance in water chestnut starch with the addition of salt. The transmittance value of the starch paste increased at salt concentrations of 0.5% w/w and 1% w/w. The enhancement in transmittance was due to the breakdown of the hydrogen bonds among the starch molecules, and also between the starch and water molecules. These hydrogen bonds hindered the starch molecules from reorganizing during retrogradation. The lower rate of retrogradation enhanced the light transmittance in these starch gels in contrast to the native starch gels. Similarly, extrusion-polyphenol treatment of WCS caused a remarkable decline in retrogradation.50 In addition, Singh et al.19 demonstrated that the transmittance of native, AM, and HMT water chestnut starches was reduced with an increase in their storage time. It was observed that acid-thinning caused an increase in the first part of light transmittance and HMT treatment decreased the ability of the starch suspension to transmit light compared to the native starch. A reduction in retrogradation is one of the reasons for the enhancement in light transmittance of the acid-thinned starch paste. The discharge of amorphous areas during acid-thinning increased the interactive bond formation among the amylopectin molecules, and hence boosted the light-transmitting efficiency.51 These results are consistent with previous research on banana starch.42 Similarly, Gani et al.7 demonstrated that water chestnut starch from Dal Lake showed higher transmittance and swelling power compared to that from Wular Lake and Anchar Lake.
4.3.4. Pasting properties.
The pasting properties of water chestnut starch include the parameters of pasting temperature (PT), peak viscosity (PV), trough viscosity (TV), final viscosity (FV), and setback viscosity (SB). Viscosity is one of the most crucial properties of starches and can provide important knowledge about their cooking behaviours during heating and cooling cycles.52 When a starch–water dispersion is shear heated at a higher gelatinization temperature, a phase change from ordered to random state occurs and the viscosity of the system enhances dramatically, resulting in the formation of starch paste. The characteristics of starch paste are affected by the amount and length of the amylose chain, as well as the width and position of the branched chains of amylopectin. Moreover, the pasting property of starches acts as an efficient mode to monitor their functionality with their structural characteristics and determining several potent applications for their use in industry as a condenser or binder.52,53 There were significant changes found in the PT, PV, TV, FV, and SB of the starch samples. Specifically, there was a constant enhancement in viscosity with an increase in temperature in the initial phase, as shown by the release of free water and reduced flow of water because of the enhancement in the amount of swollen starch granules, which occupy more space. PT is the temperature at which the viscosity starts to increase or the starch begins to gelatinize. The PT of starches was found to be between 68.67 °C (Kunming, Yunnan) and 77.76 °C (Yangjiang, Guangdong). According to Singh et al.,54 a higher PT depicts more resistance to swelling. Thus, the starches purified from chestnuts grown in Zhejiang, Jiangsu, Guangdong, and Henan revealed greater resistance to swelling in contrast to others. The starch purified from chestnut observed in Yangjiang (Guangdong) also showed a greater amylose content. The PV of chestnut starch from Zhaotong (Yunnan) was observed to be 1120.67 cP, while that from Liuzhou (Guangxi) showed the maximum PV, i.e., 4422.67 cP. Most of the starch samples showed PV values in the range of 3000 and 4000 cP. Singh et al.19 compared the pasting properties of water chestnut starch with corn and potato starch (Fig. 4). Potato starch showed the maximum PV of 8112 mPa s followed by water chestnut with 5554 mPa s and corn with 2907 mPa s. The trough viscosity also displayed a similar pattern. The breakdown viscosity (BV) was found to be minimum in native corn starch, whereas maximum in native water chestnut starch. The final viscosity (FV) was more in potato starch at 7277 mPa s followed by water chestnut and corn starch at 5624 and 2956 mPa s respectively. The increment in final viscosity could be due to the clustering of the amylose molecules.55 The setback (final viscosity minus trough viscosity) is the viscosity enhancement appearing from the movement of the amylose molecules that have been extracted from swollen starch granules during cooling and is usually employed to determine the gelling capability or retrogradation efficiency of starch.56 Water chestnut starch revealed the highest setback viscosity, which was similar in corn and potato starches. The pasting temperature of native potato starch was found to be minimum (66.97 °C), while water chestnut and corn starches showed higher pasting temperatures (75 °C and 76.77 °C). Xu and Shoemaker35 reported a greater gelatinization temperature for water chestnut starch compared to potato starch. Three potato cultivars were demonstrated to show pasting temperatures in the range of 68.2 °C to 74.5 °C.57
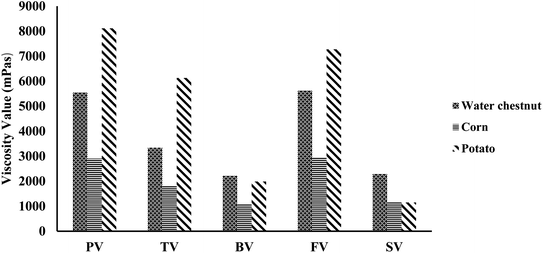 |
| Fig. 4 Comparison of the pasting properties of water chestnut starch with corn and potato starch.19 | |
The pasting properties are also based on the hardness of starch granules, which mainly affects the granule swelling potential and quantity of amylose released in solution.58 Water chestnut starches displayed a constant enhancement in viscosity with an increase in temperature. The enhancement in viscosity with temperature can be ascribed to the expulsion of water from the loosened amylose in the granules when they swelled.59 The PV was observed to be 2719 cP for the water chestnut starch from Anchar Lake, while 3714 cP in the case of that from Dal Lake. The final viscosity (FV) of water chestnut starch from Anchar Lake was found to be 3323; however, that from Dal Lake showed the maximum FV of 4385 cP.7 Miles et al.55 observed that the increment in final viscosity may be due to the clustering of the amylose molecules. The pasting temperature for water chestnut starches was found to be between 71.4 °C to 84 °C and the highest pasting temperature was shown by the water chestnut starch from Anchar Lake. The higher pasting temperature of water chestnut starches from Anchar Lake (84 °C) and Wular Lake (81 °C) compared to that from Dal Lake (71.4 °C) showed their increased obstruction towards swelling.
4.3.5. Freeze-thaw stability.
Freeze-thaw stability is a major factor to be appraised in refrigerated and frozen foods. Greater syneresis depicts the decreased freeze-thaw stability of starch. In this case, water chestnut starch showed greater freeze-thaw stability in a few mixtures and was used in the formation of frozen products and several industrial applications.24 When starch-containing foods such as sauces, ice-creams, and soups undergo repeated freeze-thawed (FT) cycles, their morphological and physicochemical status may be significantly altered. A gelatinized starch gel is a looped homogeneous system. After freezing, the starch gel system becomes heterogeneous and is divided into starch-rich and starch-deficient ice stages. Repeated FT cycles of thickened pastes result in the formation of cryotropic gel, and the final products possess a sponge-like texture.60 Duplicating the FT cycle invokes phase separation and ice growth61 due to the syneresis in the starch gel and the separation of the water from the gel. The freeze-thawed starch gels form a honeycomb-like network, which is almost cracked at the 7th FT cycle. Freeze-thawed starch gels exhibited a classic B-type crystal structure according to their X-ray diffractogram, which is distinct from native starch (A-type crystal structure). The crystallinity was found to be enhanced by recasting the FT cycles. Moreover, the thermodynamic values of the freeze-thawed starch gels were also boosted with an increase in the number of freeze-thaw cycles, and the retrogradation ratio was determined to be 30% w/w in the 7th FT cycle. In addition, the textural characteristics such as hardness and pliability of the freeze-thawed starch gels were observed to be affected by repeating the FT cycles. Freeze-thawing hindered the digestive enzyme of starch gels, which is due to the fast retrogression of seeped amylose.62 The syneresis was observed to be remarkably enhanced with an increment in freeze-thaw cycles. It was revealed that after the 5th cycle, a low proportion of syneresis appeared in HMT starch, whereas it was the greatest in the annealed starch.26 It has been observed that the liberation of water from starch gel systems preserved at low temperatures may be due to the enhanced amylose, amylose-amylopectin, or amylopectin interactions.63 During annealing, the dissolution and case crystallization of the starch granules lead to lower water absorption by the granules, which may be the probable cause for the increased syneresis in annealed starch.26 In another study, the freeze-thaw stability depicted that native water chestnut gel has a syneresis of 38% w/w, which is lower than that of sweet potato, corn, and bean starch.46,47 In contrast, the syneresis value of water chestnut gel is more than that of rice and banana starches.64 The reduction in syneresis may be due to the extensively branched amylopectin, which grips extra water, and subsequently decreases the rate of retrogradation.65
The freeze-thaw stability was boosted with the inclusion of gums and the highest increment was found with guar gum and acacia gum having values ranging from 38% w/w to 52% w/w. It was observed that the freeze-thaw stability increased upon the addition of hydrocolloids to starch because the hydrocolloids have good water absorption properties. Lee et al.47 and Funami et al.66 also demonstrated identical patterns. The insufficient hydration or swelling of starch granules is responsible for the decreased water gripping ability or enhanced syneresis in the aggregate phase. When starch is warmed in an aqueous condition, its amylopectin fraction disintegrates, resulting in the swelling of the starch; however, the amylose together with some of the amylopectin seeps out, resulting in moisture uptake.67
5. Physical modification of water chestnut starch
Natural starch suffers from the limitations of thermal instability and easy retrogradation,68 whereas modified starches possess distinct gelatinization and gel properties, and thus have been extensively used in food and related allied industries to fulfil the requirements of consumers. Moreover, physical modes of modification are gaining increasing attention from researchers due to the cost-effective modification procedures and the absence of any chemical residues compared to the chemical modes of modification.69 Physical modification techniques alter the functionality of starch in an environmentally acceptable mode without the use of any biological/chemical agents and production of any chemical waste.70 Guo et al.71 physically modified chestnut starch by high-pressure homogenization and observed that its solubility, swelling and transmittance were enhanced with an increase in pressure. However, the morphology and crystalline architecture of the starch particles remained unchanged. Dry heat treatment (continuous and repeated dry heating) resulted in a significant improvement in the gelatinization, rheology and morphology of starch, whereas continuous dry heating was found to be more effective to decrease the pasting viscosity.72 Physical modifications of starch via heat-moisture treatment (HMT) and annealing (ANN) produce desirable physicochemical properties in native starch without changing its granular structure.73–75 Heat-moisture-treated and annealed starches play a vital role in the food industry owing to their enhanced thermal stability and reduced degree of retrogradation.73 Both HMT and ANN are closely related processes, which can be controlled by various parameters including the starch: moisture ratio, heating period and temperature; however, they differ in the quantity of water required and temperature conditions.76 Elevated temperatures in the range of 90 °C to 120 °C above the gelatinization temperature and limited moisture content ranging from 10–30% are essential for HMT, whereas annealing needs lower temperatures below the gelatinization point and higher than the glass transition temperature in the presence of an excess amount of water (50% to 60%).75 HMT restricts the molecular mobility at elevated temperatures by controlling the quantity of water.76 However, the structural and functional modifications of starches are dependent on the source of starch and amount of amylose present in them.70 Annealing causes reorganisation in starches, giving them a well-organised configuration comprised of double helices of amylopectin.77
Recently, the interest in the blending of starches with hydrocolloids to alter their rheological properties has been increasing. The properties of the blend depend on the concentration of the hydrocolloids and starches, conditions for the preparation of the blend and type and level of interactions between them.78 The presence of hydrocolloids in starches primarily influences their flow, texture and viscosity, and also provides shear stability to the starch granules together with decreased syneresis.79 Consequently, they provide stability and enhance the quality of food products. The treatment of starches with various hydrocolloids intensifies the outcomes of their physical modification, particularly the stability and gel formation ability of starch pastes.68,80,81 The addition of hydrocolloids is also a very effective method for the physical modification of starches, and simultaneously is more economical. Carboxy methyl cellulose (CMC), a derivative of chitosan, is the most employed cationic hydrocolloid, which contains carboxyl and amino groups attached to it and is extensively used in the food industry owing to its good solubility in water.82 Similarly, xanthan gum (XG) is an anionic hydrocolloid having a rigid β-glucan backbone and polar water-loving trisaccharide side chains, which impart gel-thickening and emulsifying stability to starch pastes.80 Likewise, the anionic hydrocolloid sodium alginate (SA) is also associated with excellent gel thickening and stabilization property. Water chestnut starch has potential to serve as a promising starch resource in the food processing industry but it has not been exploited fully due to the poor understanding of its modification. Also, thorough exploration of the properties of water chestnut starch can be helpful for its applications in the food and other allied industries and will permit its modification for application-oriented utility. Some researchers have explored the effects of hydrocolloids on the physicochemical properties of WCS. The prime aim of these modifications is to improve the properties of WCS to accomplish the requirements of starch-based foods. Gul et al.27 studied the effects on the physicochemical, thermal, pasting and morphological properties of water chestnut starch (WCS) by modification through dry heating and addition of ionic gums, i.e., carboxy methyl cellulose (CMC) and sodium alginate (SA). Native starch was modified by dry heating treatment (DHT) and with and without the addition of ionic gums using a reported method.83 Initially, gum solutions of (0.4 g) SA and CMC were prepared separately by vigorous stirring them in distilled water (70 mL), and the dispersion formed by adding starch (39.6 g) to these prepared gum solutions was stirred continuously at room temperature for 30 min, and then transferred to a dish and dried in an oven at 45 °C. Similarly, Lutfi et al.23 evaluated the functional properties of water chestnut (Trapa bispinosa) starch in the presence of four different gums, i.e., xanthan, CMC, guar and acacia, by preparing aqueous solutions of isolated WCS with hydrocolloids including XG, gum acacia, gum guar and CMC having a moisture content of 10.1%, 14.3%, 13.7% and 11.5% w/w, respectively (Table 3).
Table 3 Physical modifications of starch
Type of starch |
Solubility (g per 100 g) |
Swelling power (g g−1) |
Water binding capacity (g g−1) |
Oil binding capacity (g g−1) |
Viscosity |
Thermal Characteristics |
Light transmittance |
Physiochemical characteristics |
References |
Moisture |
Ash |
Amylose |
Total carbohydrates |
Native starch |
19.5 |
8.67 |
106 |
122.3 |
PV = 3402 cP |
T
o = 70.53 °C |
6.9 |
9.12 |
0.34 |
28.77 |
70.1 |
Gul et al., 2014 27 |
BV = 1980 cP |
T
p = 79.64 °C |
SV = 2748 cP |
T
e = 84.65 °C |
PT = 81.7 °C |
ΔH = 12.9 (J g−1) |
Native starch + heating (130 °C for 2 h) |
19.6 |
7.78 |
120.2 |
150.2 |
PV = 3867 cP |
T
o = 31.51 °C |
5.8 |
4.71 |
0.36 |
28.81 |
74.56 |
BV = 2429 cP |
T
p = 79.16 °C |
SV = 2988 cP |
T
e = 84.33 °C |
PT = 81.21 °C |
ΔH = 11.3 (J g−1) |
Native starch + Heating (130 °C for 4 h) |
18.2 |
7.39 |
134 |
153.4 |
PV = 4327 cP |
T
o = 69.46 °C |
6.4 |
4.60 |
0.35 |
29.69 |
74.14 |
BV = 2798 cP |
T
p = 78.54 °C |
SV = 3423 cP |
T
e = 85.2 °C |
PT = 81.8 °C |
ΔH = 17.3 (J g−1) |
Native starch + CMC |
20 |
7.53 |
122.2 |
140.1 |
PV = 3286 cP |
T
o = 70.82 °C |
5.1 |
9.81 |
0.69 |
26.78 |
75.45 |
BV = 1902 cP |
T
p = 79.76 °C |
SV = 3180 cP |
T
e = 85.56 °C |
PT = 81.65 °C |
ΔH = 32.6 (J g−1) |
Native starch + CMC (130 °C for 2 h) |
19.4 |
7.45 |
122 |
154.3 |
PV = 4317 cP |
T
o = 65.46 °C |
4.9 |
4.31 |
0.68 |
27.37 |
74.91 |
|
BV = 2875 cP |
T
p = 79.75 °C |
SV = 3822 cP |
T
e = 85.98 °C |
PT = 79.75 °C |
ΔH = 27.1 (J g−1) |
Native starch + CMC |
18.8 |
7.22 |
138.1 |
151.1 |
PV = 3950 cP |
T
o = 31.26 °C |
4.6 |
4.24 |
0.69 |
24.43 |
74.66 |
BV = 2303 cP |
T
p = 78.06 °C |
SV = 3567 cP |
T
e = 84.3 °C |
PT = 80 °C |
ΔH = 29.9 (J g−1) |
Native starch + SA |
17.6 |
7.13 |
136.1 |
157.1 |
PV = 3569 cP |
T
o = 71.09 °C |
6.3 |
9.67 |
0.74 |
26.56 |
76.12 |
BV = 2042 cP |
T
p = 79.87 °C |
SV = 2991 cP |
T
e = 85.35 °C |
PT = 81.5 °C |
ΔH = 20.7 (J g−1) |
Native starch + SA (130 °C for 2 h) |
17.2 |
6.97 |
148.2 |
163.2 |
PV = 4299 cP |
T
o = 71.63 °C |
6.8 |
4.69 |
0.73 |
25.6 |
76.56 |
BV = 2786 cP |
T
p = 79.12 °C |
SV = 3738 cP |
T
e = 84.95 °C |
PT = 80 °C |
ΔH = 16.9 (J g−1) |
Native starch + SA (130 °C for 4 h) |
16.5 |
6.26 |
151.1 |
167.1 |
PV = 4404 cP |
T
o = 72.69 °C |
5.4 |
3.77 |
0.73 |
24.77 |
75.45 |
BV = 2742 cP |
T
p = 79.13 |
SV = 3778 cP |
T
e = 84.41 |
PT = 79.95 °C |
ΔH = 20 (J g−1) |
Native starch |
60 °C = 2.8 |
60 °C = 2.61 |
98.21 |
|
PV = 72.3BU |
ND |
3.5 |
— |
— |
— |
— |
Lutfi et al., 2017 23 |
70 °C = 3.8 |
70 °C = 5.58 |
BV = 0 |
80 °C = 5.6 |
80 °C = 9.60 |
SV = 25.2 BU |
90 °C = 5.7 |
90 °C = 12.23 |
T
g = 77.2 °C |
Native starch + XG |
60 °C = 9.9 |
60 °C = 3.99 |
109.0 |
|
PV = 98.2BU |
|
4.3 |
— |
— |
— |
— |
70 °C = 17.5 |
70 °C = 5.45 |
BV = 19.1 BU |
80 °C = 17.7 |
80 °C = 8.53 |
SV = 36.5 BU |
90 °C = 7.5 |
90 °C = 6.14 |
T
g = 77.1 °C |
Native starch + guar gum |
60 °C = 13.8 |
60 °C = 2.30 |
72.7 |
|
PV = 79.5BU |
|
4.6 |
— |
— |
— |
— |
70 °C = 11.8 |
70 °C = 3.13 |
BV = 2.1 BU |
80 °C = 13.5 |
80 °C = 6.67 |
SV = 34.2 BU |
90 °C = 11.6 |
90 °C = 6.69 |
T
g = 77.5 °C |
Native starch + CMC |
60 °C = 15.8 |
60 °C = 2.51 |
72.7 |
|
PV = 66.2U |
|
4.1 |
— |
— |
— |
— |
70 °C = 13.8 |
70 °C = 2.85 |
BV = 1.4 BU |
|
80 °C = 9.7 |
80 °C = 5.22 |
SV = 32.1 BU |
90 °C = 15.4 |
90 °C = 6.94 |
T
g = 74.2 °C |
Native starch + gum acacia |
60 °C = 15.8 |
60 °C = 2.06 |
36.3 |
|
PV = 65.4BU |
|
3.6 |
— |
— |
— |
— |
70 °C = 13.6 |
70 °C = 2.65 |
BV = 0BU |
80 °C = 17.5 |
80 °C = 5.97 |
SV = 20.5 BU |
90 °C = 13.6 |
90 °C = 6.86 |
T
g = 79.2 °C |
Native starch |
|
|
98.2 |
|
PV = 72.3BU |
|
3.5 |
— |
— |
— |
— |
BV = 0 |
SV = 25.2 BU |
T
g = 77.2 °C |
Native starch + NaCl (0.5%) |
|
|
76.19 |
|
PV = 70.1BU |
|
3.9 |
— |
— |
— |
— |
BV = 2.1 |
SV = 17.2 BU |
T
g = 78.2 °C |
Native starch + NaCl (1%) |
|
|
95.45 |
|
PV = 78.5BU |
|
4.4 |
— |
— |
— |
— |
BV = 1.1 |
SV = 18.2 BU |
T
g = 77.8 °C |
Native starch + NaCl (2%) |
|
|
70.83 |
|
PV = 61.2BU |
|
3.1 |
— |
— |
— |
— |
BV = 1.4BU |
SV = 30.1 BU |
T
g = 84.2 °C |
Native starch + XG |
|
|
176.92 |
|
PV = 170.2BU |
|
3.2 |
— |
— |
— |
— |
BV = 52.0BU |
SV = 37.2 BU |
T
g = 70.6 °C |
Native starch + XG + NaCl (0.5%) |
|
|
370.3 |
|
PV = 172.1BU |
|
0.3 |
— |
— |
— |
— |
BV = 27.1BU |
SV = 42.2 BU |
T
g = 71.9 °C |
Native starch + XG + NaCl (1%) |
|
|
300.8 |
|
PV = 176.5BU |
|
1.0 |
— |
— |
— |
— |
|
BV = 16.1BU |
SV = 41.2 BU |
T
g = 75.2 °C |
Native starch + XG + NaCl (2 h) |
|
|
211.3 |
|
PV = 185.2BU |
|
1.8 |
— |
— |
— |
— |
|
BV = 10.4BU |
SV = 39.1 BU |
T
g = 80.1 °C |
Native starch + Heating (2 h) |
|
|
1.66 |
|
PV = 780.63 RVU |
T
o = 65.34 °C |
3.18 |
9.73 |
0.37 |
28.90 |
74.36 |
Lutfi et al., 202184 |
BV = 719.56 RVU |
T
p = 75.76 °C |
SV = 200.34 RVU |
T
c = 80.56 °C |
T
g = 64.65 °C |
ΔH = 12.34 (J g−1) |
Native starch + Heating (4 h) |
|
|
2.01 |
|
PV = 817.76RVU |
T
o = 62.14 °C |
3.17 |
9.34 |
0.36 |
27.80 |
74.25 |
BV = 730.08RVU |
T
p = 74.12 °C |
SV = 267.03RVU |
T
c = 81.80 °C |
T
g = 65.14 °C |
ΔH = 11.03 (J g−1) |
Native starch + SA |
|
|
1.501 |
|
PV = 518.56RVU |
T
o = 67.90 °C |
3.05 |
9.61 |
0.71 |
29.54 |
76.14 |
BV = 164.54RVU |
T
p = 77.89 °C |
SV = 86.14RVU |
T
c = 82.54 °C |
T
g = 68.40 °C |
ΔH = 21.14 (J g−1) |
Native starch + SA + Heat(130 °C, 2 h) |
|
|
1.66 |
|
PV = 480.56RVU |
T
o = 68.10 °C |
2.94 |
8.68 |
0.70 |
28.84 |
76.31 |
BV = 141.84RVU |
T
p = 77.51 °C |
SV = 85.31RVU |
T
c = 81.84 °C |
T
g = 67.10 °C |
ΔH = 17.31 (J g−1) |
Native starch + SA + Heating (130 °C, 4 h) |
|
|
2.16 |
|
PV = 450.45RVU |
T
o = 68.56 °C |
2.84 |
8.38 |
0.69 |
27.81 |
75.40 |
BV = 147.34RVU |
T
p = 77.23 °C |
SV = 118.12RVU |
T
c = 81.34 °C |
T
g = 66.56 °C |
ΔH = 21.12 (J g−1) |
Native starch |
|
|
|
|
PV = 2731 cP |
T
o = 66.77 °C |
— |
— |
— |
— |
— |
BV = 347 cP |
T
p = 71.99 °C |
SV = 2291 cP |
T
c = 79.84 °C |
PT = 76 °C |
ΔH = 12.04 (J g−1) |
Native starch + HMT (20 °C) |
|
|
|
|
PV = 1576 cP |
T
o = 75.53 °C |
— |
— |
— |
— |
— |
Yadav et al., 2013 26 |
BV = 194 cP |
T
p = 78.85 °C |
SV = 968 cP |
T
c = 89.01 °C |
PT = 80.9 °C |
ΔH = 19.18 (J g−1) |
Native starch + HMT (25 °C) |
|
|
|
|
PV = 1864 cP |
T
o = 81.09 °C |
— |
— |
— |
— |
— |
BV = 182 cP |
T
p = 85.48 °C |
SV = 1088 cP |
T
c = 90.25 °C |
PT = 85.1 °C |
ΔH = 12.65 (J g−1) |
Native starch + HMT (30 °C) |
|
|
|
|
PV = 1637 cP |
T
o = 82.98 °C |
— |
— |
— |
— |
— |
BV = 57 cP |
T
p = 87.35 °C |
SV = 856 cP |
T
c = 91.87 °C |
PT = 87.3 °C |
ΔH = 12.84 (J g−1) |
Annealed starch |
|
|
|
|
PV = 662 cP |
T
o = 81.58 °C |
— |
— |
— |
— |
— |
|
BV = −223 cP |
T
p = 84.96 °C |
SV = 764 cP |
T
c = 88.12 °C |
PT = 85.8 °C |
ΔH = 12.59 (J g−1) |
5.1. Impact on morphology
Lutfi et al.23 observed roughness on the surfaces of WCS granules containing carboxy methyl cellulose (CMC) via scanning electron microscopy prior to gelatinization due to the adherence of CMC on the surfaces of the starch granules. In contrast, after gelatinization, the starch granules were ruptured and formed a large number of smaller lumps, which provided compactness to the starch structure. Similar roughness in starch granules was also observed in another report.27 The authors concluded that the granular size of WCS was affected to a lower extent and insignificant damage to its surface morphology occurred with the addition of CMC or SA. Additionally, the average size of the granules was found to be bigger when treated with SA (26 μm) than that in the presence of CMC (21 μm). The SEM images of the modified starches (with SA and CMC) indicated roughness and the presence of cracks on their granular surfaces because of the leaching of amylose in the presence of heat; also, the amount of cracks increased with an increase in temperature. Dry heating of WCS in the presence of sodium alginate for 4 h at 130 °C also resulted in similar rupturing of the starch granules.84 In another study, the effect of NaCl on WCS and WCS-XG blends was investigated and Lutfi et al.45 observed that in comparison to the oval, bulging, undamaged granules having smooth surfaces present in native WCS, the presence of NaCl at different levels of concentration yielded defective and ruptured starch granules, which had lost their original shapes and exhibited an increase in porosity. In the WCS-XG blend, the SEM images indicated presence of both intact swollen starch granules and gum penetrated inside the starch granules. The addition of NaCl to the WCS-XG combination produced more defective granules with reduced sizes and increased porosity.
5.2. Impact on physico-chemical properties
Annealed- and heat-moisture-treated (HMT) water chestnut starches exhibited no change in amylose content (20.1–21.5 g per 100 g) in contrast to the 21.8 g per 100 g present in the native starch, supporting the fact that no chemical modifications in starch takes place after heat moisture treatment and annealing.19,26,85
The physicochemical properties of water chestnut starch (WCS) are significantly affected by the addition of ionic gums in the absence and presence of heating. Gul et al.27 investigated the physicochemical properties of native and heat-treated WCS in the presence of ionic gums including SA and CMC and noticed that the moisture content in native starch, WCS-CMC and WCS-SA blends exhibited a remarkable decrease on heating and the decrease in moisture content became more significant on increasing the duration of heating to 2 h and 4 h. Heat penetrated the interior of the starch granules and decreased their moisture content. Also, starch tailored with sodium alginate gum displayed a higher ash content (0.73–0.74 g per 100 g) in contrast to (0.68–0.69 g per 100 g) in starch modified with CMC, whereas the ash content in native starch was observed to be 0.34 g per 100 g. Similarly, an increase in ash content due to the addition of XG and heating the blend at 130 °C for a duration of 2–4 h was also observed. The starches modified with ionic gums, i.e., CMC, SA and XG, were observed to have a higher amylose content (28.77–24.43) g per 100 g and higher carbohydrate content (70.01–76.56) g per 100 g in comparison to that present in native starch. However, starch modified by gums (CMC, XG and SA) exhibited a significant decrease in carbohydrate content when subjected to heat treatment.27
5.2.1. Impact on water binding capacity and oil binding capacity.
The water absorption capacity (WAC) signifies the degree of association between polymeric chains of amylose and amylopectin present in starch granules.86 In comparison to other available starches, WCS has a larger WAC owing to the large size of its granules27 and the weaker forces of attraction between the amylose and amylopectin chains of native starch.87 Modification of WCS with sodium alginate and CMC in the presence of heating is associated with remarkable changes in the WAC of its granules. Upon DHT, starch granules exhibited an increase in water absorption capacity in comparison to native unmodified starch, which increased with an increase in the duration of heating from 2 to 4 h. Heat treatment exposes the hydrophilic groups of the granules and increases their water absorption tendency. Starches modified with ionic gums have increased concentration of carboxyl groups, which significantly increase the absorption of water molecules. Heating further intensified the starch–alginate interactions, thereby increasing the surface area for water absorption. Dry heating of water chestnut starch caused an increase in its oil binding capacity from 122.32 g g−1 in the native starch to 167.1 g g−1 when heated for a duration of 4 h. Hydrocolloid-modified WCS with CMC and SA increased its OAC significantly. Heating of WCS for a longer time and addition of CMC and SA increased its lipophilic character. Moreover, the effect of sodium alginate (SA) on OAC of WCS was more prominent than the effect of carboxy methyl cellulose (CMC) with an increase in oil binding capacity from 153.4.1 g g−1 in native starch to 167.1 g g−1 in the sodium alginate–water chestnut starch blend on heating at 130 °C for a duration of 4 h. In contrast, the oil binding capacity of the CMC-containing starch only marginally changed from 140.1 g g−1 to 154.3 g g−1 when heated for a period of 2 h. In another study performed by Lutfi et al.,23 the addition of xanthan gum (XG) to native WCS increased its water absorption capacity considerably, which is probably due to the increase in hydrophilicity and increased possibility of interactions of water molecules via hydrogen bonding with the larger number of hydroxyl groups attached to XG.88 Contrarily, the presence of guar, acacia or CMC in WCS decreased its WAC possibly due to the electrostatic nature of the water-polymeric starch chain interactions, which is inhibited by the presence of these gums. However, WCS modified with sodium alginate gum exhibited an increase in WAC when the temperature was increased from 2 to 4 h.84 The presence of NaCl in WCS significantly affected its WAC and the inverse relationship existed between the two.45 The movement of water molecules inside the starch granules is prevented in the presence of salt due to the formation of an electrostatic barrier. The addition of salt to the combination of WCS and XG caused a remarkable increase in the WAC of the blend due to the synergistic effect of the salt and XG.89
5.2.2. Impact on swelling power and solubility.
The amylose content influences the water solubility, while amylopectin affects the swelling power of starches. The swelling power of starches is influenced by the aggregated structure of their granules and the extent of interactions in the starch chains prevailing in both the crystalline and amorphous parts of starch.90 Both annealing and HMT bring about a significant decrease (p < 0.05) in swelling power and solubility in water chestnut starch, which is probably due to the increase in crystallinity, increased interactions between amylose and amylopectin molecules, stronger intramolecular forces of attraction, transformations in the crystalline areas of starch,91 lower hydration of the amorphous regions of starch92 and increased organisation of its molecules. However, the swelling capacity was found to be a function of temperature and its average value increased appreciably with an increase in temperature (p < 0.05). Both the increased crystalline organisation and extent of interaction of amylose with itself and with amylopectin due to annealing prevent the hydration of the amorphous parts of starch, thereby decreasing the swelling ability of starch granules.26 Both annealing and heat-moisture-treatment result in decreased water solubility in water chestnut starch. The increased organisation of the WCS molecules due to annealing results in the lower solubilization of starch granules.93 Additionally, annealing results in the strengthening of the amylose bonds with amylose itself and with amylopectin and inhibits the leakage of the starch granules. In contrast, the decreased solubility of starch molecules due to HMT may be because of the decreased stability of the starch granules and disentanglement in the double helical structure of the native starch.77
Gul et al.27 noticed that water chestnut starch (WCS)-modified by heat treatment exhibited a decrease in swelling power, which is attributed to the damage caused by heating. The inhibited swelling of hydrocolloid (CMC and SA)-modified starches is due to the formation of a complex matrix with amylose present outside the starch granules.94 CMC-modified starches exhibit a considerable decrease in solubility probably due to the formation of ester linkages between the starch and CMC on dry-heating. However, more severe inhibition of swelling was caused by CMC than that produced by sodium alginate. Similarly, reduction in solubility was mentioned by Lutfi et al.84 on the addition of SA to WCS due to the reduced water availability caused by the hydrophilic character of the gum and formation of a complex matrix with the previously leached amylose, which prohibits the swelling of the granules and more leaching of amylose.95 In another study, Lutfi et al.23 evaluated the swelling power of blends of water chestnut starch-gums (acacia, xanthan, and guar) at different temperatures. The swelling of these blends was found to be temperature dependent and took place before gelatinization. Swelling increased quickly with an increase in temperature after gelatinization. Maximum swelling of water chestnut starch was recorded at 90 °C. However, its swelling power was reduced considerably with the addition of different gums. The reduction in swelling power can be attributed to the strong interaction of gums with leached amylose, which restricts the swelling of the starch granules.96 At the onset of heating, the gelatinized granules of starch approach each other and are held by the gums, which increases the forces of attraction between the two and increases the diffusion of water and subsequent leaching of amylose. When the temperature further increases (beyond 80 °C and 90 °C), the leached amylose and gums form a covering around the starch granules, restricting the leaching process. Moreover, the presence of hydrocolloids alters the rheology of water, which prevents the entry of water molecules in the swollen granules. Among the four hydrocolloids, gum acacia produced the largest decrease in the swelling power of WCS due to its lower viscosity observed at lower concentrations (<10% w/w).97 Native starch exhibited the highest solubility of 5.7 g g−1 at 90 °C. An increase in solubility was observed with the addition of all the gums but the maximum effect was exhibited by CMC, which dramatically increased the solubility of WCS to 15.4 g g−1. Likewise, the swelling power of WCS was observed to decrease when subjected to a high temperature of 130 °C for a duration of 2–4 h, which was further reduced upon the addition of sodium alginate.84
The swelling power of WCS decreased in the presence of NaCl at the 0.5% w/w level from a value of 12.33 g g−1 in native starch to 5.60 g g−145 and an increase in the concentration of NaCl to 2% w/w further reduced its swelling power to 4.65 g g−1. The most probable cause for this reduction in swelling power is the competition between the starch and NaCl salt for water molecules. Due to the decrease in swelling power, the leaching of amylose also decreases.98 Additionally, the swelling power of a water chestnut starch–XG mixture was lowered with the 2% level of salt concentration. Lufti et al.45 reported a lower solubility value (5.7% at 90 °C) for WCS than that reported by Gul et al.27 This could be because of the difference in cultivars of WCS. With an increase in the concentration of NaCl, the solubility of WCS also increased. The large-sized chloride ion, due to its good polarization power, disturbs the hydrogen bonding interactions between starch–water and starch–starch molecules and increases the access of more water molecules, thereby increasing the solubility of WCS.99 An increase in the solubility of WCS in the presence of XG was also reported. The addition of NaCl at the 2% w/w concentration level dramatically increased the solubility of the WCS-XG blend and a maximum solubility of 21.2 g g−1 was detected.45
5.2.3. Impact on paste clarity.
In comparison to native starch, annealed starches exhibit higher paste clarity, while HMT starches have comparatively lower paste clarity. The paste clarity varies with time and significantly decreases with an increase in the storage time of chestnut starch paste.26 Upon annealing, the swollen starch granules become fragile and starch pastes comprised of more damaged granules leads to better transmittance of light in contrast to that containing larger amounts of intact swollen granules.100 An increase in storage period led to a decrease in % transmittance (T), similar to the effect reported for banana starch.42
As investigated by Gul et al.,27 the paste clarity of modified starches varied significantly (p < 0.05) in comparison to native starch. When water chestnut starch (WCS) was heated to a temperature of 130 °C, the paste clarity was reduced from 6.9% w/w in native starch to 4.6% w/w. Heating of a starch suspension to a high temperature causes rupturing of the starch granules, which results in the leaching of amylose and formation of shorter chains of amylose with an increase in temperature and duration of heating. Cooling initiates the reassociation of amylose to form a network during the process of retrogradation. The paste clarity of WCS modified by adding gums varied significantly (p < 0.05) in comparison to that of native WCS.23 The %T of native WCS was reported to be 3.5% w/w, which is much lower than the previously reported values7 probably due to the heating of starch suspensions as explained by Gul et al.27 The presence of selected hydrocolloids had insignificant effects on the paste clarity of native water chestnut starch. Additionally, the gums bind with the leached amylose and cause opacity in the starch pastes. Likewise, a noticeable decrease in paste clarity of WCS occurred upon the addition of sodium alginate gum in comparison to the native unmodified WCS.84 In contrast, the addition of NaCl at the 0.5% w/w and 1% w/w levels resulted in a noticeable increase in the paste clarity of WCS. The addition of salt cleaves the hydrogen bonds present between starch–starch and starch–water systems to certain extent and prohibits the rearrangement of the starch molecules during retrogradation, subsequently increasing the paste clarity. Conversely, the addition of NaCl to the WCS-XG blend decreased the paste clarity.45
5.2.4. Impact on pasting properties.
Both heat-moisture treatment (HMT) and annealing alter the pasting properties of water chestnut starch considerably. Hydrothermal treatment of starches causes alterations in their crystallinity and association of chains present in the amorphous areas of starch and these transformations increase with an increase in the moisture content. Due to the increase in the strength of these intragranular bonds and presence of cross-links, more heat is needed for the disintegration of the starch structure and for paste formation.101 Therefore, an increase in pasting temperature takes place on HMT. According to Yadav et al.,26 native-unmodified water chestnut starch exhibited a pasting temperature of 76 °C, while the hydrothermally modified and annealed starch displayed an increase in pasting temperature ranging from 80 °C to 85 °C. Both the annealed and heat-moisture-treated water chestnut starch displayed reduced values for PV, SB and BD viscosities although the annealed starch exhibited the lowest values for all the viscosities. However, a decline in peak viscosity, breakdown viscosity, final viscosity and retrogradation was observed after HMT, which is probably due to the increased reorganisation and reduced swelling capacity of the water chestnut starch granules, resulting in the restricted leaching of amylose in the starch medium.26 The decrease in breakdown viscosity and SB viscosity on hydrothermal treatment indicated more thermal and shear stability of water chestnut starch pastes. The decrease in SB viscosity on annealing and HMT can find potential applications in frozen and canned food. The influence of annealing on the pasting characteristics of starch is still under debate. In the case of potato starch, it causes an increase in pasting temperature and final viscosity but it reduces the peak viscosity,102,103 while in the case of pea, rice and wheat starch, annealing increases both their peak and final viscosities.102 However, the structural characteristics and analysis conditions during annealing affect the pasting properties of starch. Annealed starches offer better resistance to heat and mechanical agitation than native starches based on the heating rates.104 Annealing also promotes the strengthening of bonds, resulting in higher gelatinization temperatures.105 Annealing of water chestnut starch resulted in an increase in the pasting temperature and better shear stability due to the lower swelling and leaching of amylose and decrease in the peak viscosity and viscosity at the termination of the cooling cycle.85
The heating effects of starches are enhanced in the presence of hydrocolloidal gums, which increases the paste viscosities.27 Additionally, heating disrupts the granular structure of starches, which also increases the paste viscosity. On increasing the duration of heating from 2 h to 4 h, the amorphous region of WCS was altered, which increased the values of the breakdown viscosity, final viscosity and setback viscosities. However, the addition of gums caused decrease in peak viscosity, which is probably because the heat treatment and the addition of CMC and SA altered the packing arrangement of the starch chains, accompanied by some melting of the crystal structure in the presence of high temperature.27 Starch modified with CMC in the absence of heating displayed the lowest peak viscosity, which is probably due to the sticking of ionic gums on the surface of the starch granules, creating an overall negative charge on the surface and restricting the approach of water molecules. This causes delayed swelling of the granules. Heating of a WCS-CMC blend to 130 °C for 2 h resulted in a remarkable increase in the peak and final viscosities. The paste viscosity increased prominently when heating was performed after the addition of CMC owing to the faster impregnation of the reactive carboxy methyl cellulose (CMC) into the starch aqueous solution.83,106,107 A marginal increase in PV and FV occurred on the addition of SA in the absence of heat treatment in comparison to the control starch. However, the increase caused by SA was comparatively less than that caused by CMC. Heating the starch–sodium alginate blend for 2 h caused a significant increase in peak viscosity due to the increased interactions between the carboxyl groups of sodium alginate and –OH groups of starch on the removal of moisture on heating. Likewise, among the tested gums including acacia, xanthan, acacia and CMC, early gelatinization could only be achieved in the case of CMC-containing WCS.23 The addition of carboxy methyl cellulose created new interactions between the hydroxyl groups of CMC and water chestnut starch, which reduced the pasting temperature of the blend. The peak viscosity of the starch–gum mixture significantly increased in the presence of xanthan and guar gums (from 72 to 98 and 79 BU, respectively), whereas a decrease in peak viscosity was observed in the presence of gum acacia and CMC probably due to the restricted leaching of amylose from the starch granules. WCS exhibited zero breakdown, which suggested high paste stability and excellent shearing resistance. The addition of different gums had no significant effect on the breakdown viscosity of WCS. However, the breakdown viscosity increased in the presence of xanthan gum, which decreased the stability of water chestnut starch during cooking.23 The addition of gums is supposed to increase the shear forces and breakdown of the granules, causing easy disruption of the granular integrity of starch. However, the interaction of gums (xanthan, acacia, guar and CMC) with WCS had no effect on swollen granules of starch and rendered the breakdown tough. The setback (SB) viscosity of WCS was found to be enhanced in the presence of all the tested gums of guar, CMC and xanthan with the maximum increase detected in the presence of XG, whereas the minimum was observed with gum acacia. The increase in SB viscosity caused by xanthan, CMC and guar signified the enhancement in swelling power of the starch granules due to the restricted oozing amylose during gelatinization. In contrast, the decrease in SB viscosity in the presence of gum acacia was perhaps due to the interaction of gum with the leached amylose, which delayed the re-association of the amylose chains, and consequently decreased the setback ability of water chestnut starch.
A hydrocolloid-starch mixture displayed significantly improved pasting properties in the presence of salts. Recently, the influence of different concentrations of NaCl salt (0.5% w/w, 1% w/w and 2% w/w) on the pasting characteristics of water chestnut starch (WCS) alone and WCS-xanthan gum (XG) mixtures at fixed concentrations of water chestnut starch (5% w/w) and xanthan gum (0.3% w/w) has also been investigated. A linear relationship was observed between the pasting temperatures of both the native WCS and WCS-XG complex and concentration of NaCl, whereas the peak viscosity, breakdown values and setback values decreased in WSC alone and a pronounced increase in peak viscosity, breakdown and setback values of WCS-XG complex was observed. The increase in peak viscosity was a synergistic effect of the biphasic system and presence of XG in the entire volume of the continuous phase, causing lesser accessibility due to the swelling of starch during the course of pasting. The breakdown values and setback values of the WCS-XG blend increased due to the reduced stability and increased retrogradation, respectively, promoted by hydrocolloids.45
5.2.5. Impact on gelatinization/thermal characteristics.
The heating of WCS results in a decrease in its gelatinization temperature and enthalpy of gelatinization due to the disruption of its granular structure.27 Accordingly, the minimum gelatinization temperature (To = 31.51 °C) was detected when WCS was heated for a duration of 2 h. Additionally, heating starch for a longer period of 4 h resulted in an increased gelatinization temperature (69.46 °C), whereas a much higher gelatinization temperature of 70.53 °C was observed for its non-heated native starch counterpart. A marginal increase in the gelatinization temperature was observed in the presence of sodium alginate (SA) gum without heating WCS. However, and increase in heating duration from 2 to 4 h increased To, which is probably due to the inhibition of gelatinization caused by the restricted interactions between the large-sized gum molecules and comparatively smaller starch molecules. The onset temperature (To) attained its lowest value (31.26 °C) when CMC was added to WCS and the heating period was 4 h. The enthalpy of gelatinization (ΔHgel) significantly increased on heating WCS for a period of 4 h (12.9 J g−1 to 17.30 J g−1). However, the addition of CMC and SA gums resulted in a further intensification in the ΔHgel values, which exhibited a decrease with an increase in the heating duration from 2 to 4 h. A similar decrease in gelatinization temperature was also reported by Lutfi et al.,84 where on heating the native water chestnut starch for a period of 4 h (from 65.34 °C to 62.14 °C) due to the disruption of its cell walls at elevated temperatures. Additionally, Tp and Tc decreased due to the weakened starch granules. However, when sodium alginate was added to WCS before heating, a small increase in To (67.90 °C) and a significant increase in the enthalpy of gelatinization (21.14 J g−1) was observed. The restricted gelatinization indicated the presence of strong interactions between starch–sodium alginate, which caused the starch granules to become compact.
In comparison to native chestnut starch, annealed and heat-moisture-treated (HMT) starches exhibited an increase in To, Tp and Tc of gelatinization together with a significant decline in the gelatinization temperature range.26 Moreover, the gelatinization temperature range (Tc–To) varied inversely with the moisture content of the water chestnut starch. However, the lowest gelatinization temperature range was displayed by the annealed starch (88.12 °C to 81.58 °C). During the gelatinization process, the crystalline parts and double helices of starch melt, which is decided by the hydration and swelling abilities of the amorphous parts of the granules. Swelling of the amorphous regions puts a pressure on the crystalline regions. Post HMT, the new interactions between amylose–lipid and amylose–amylose restrict the mobility of the amylopectin chains present in the amorphous areas, and consequently a comparatively higher temperature is required for swelling and breaking of the crystalline areas.91 This leads to an increase in the onset temperature (To), peak temperature (Tp) and conclusion temperature (Tc) of gelatinization. Annealing influences the gelatinization characteristics of native water chestnut starch, with a remarkably increase in To (from 66.77 °C to 81.58 °C), Tp (from 71.99 °C to 84.96 °C) and Tc (from 79.84 °C to 88.12 °C). However, a marginal decrease in To (from 69.58 °C to 68.72 °C) and ΔHgel (from 7.29 to 7.21 J g−1) was reported by Singh et al.19
5.2.6. Impact on freeze thaw stability.
Native WCS has a syneresis value of 37.8% w/w22 and this low value is perhaps due to the presence of substantially branched amylopectin, which has greater water holding capacity and lower rate of retrogradation.65 The addition of gums substantially increased the freeze-thaw stability of WCS with highest increase observed with acacia and guar gums (52.0% w/w syneresis value). Generally, the addition of hydrocolloids to starch increases its freeze-thaw stability because of the good WAC of hydrocolloids. However, Lutfi et al.23 reported the opposite results, probably due to the incomplete swelling of water chestnut starch, which decreased the water holding capacity of the granules. The addition of gum acacia or guar gum to WCS restricted the swelling of the starch granules due to the generation of a polysaccharide barrier, which partially or totally encloses the starch granules and prohibits moisture intake.
The evaluation of the syneresis of WCS and WCS-XG blend in the absence and presence of NaCl was performed. With the incremental addition of salt, the syneresis value (37.8% w/w) of WCS exhibited an increase in cold storage possibly because of the stronger molecular interactions between the WCS chains or due to the re-organization of starch at lower temperatures, facilitating water leakage from the starch pastes. Moreover, the addition of 2% w/w concentration of sodium chloride to the WCS-XG blend caused a remarkable increase in syneresis (62.0% w/w) due to the probable starch–salt interactions.45
6. Chemical modification of water chestnut starch
Native starch is associated with problems of insolubility in cold water and easy retrogradation. Therefore, with the intention of overcoming the unsuitable physiochemical properties of native starch, chemical modification is the most appropriate approach for introducing different functionalities in its backbone to alter its morphological and physicochemical properties. The chemical modification of starch can be achieved using diverse methods including grafting, etherification, esterification, oxidation, acidification and dual modification. The properties of modified starches are governed by factors including source of starch biopolymer, conditions and methods exploited for their modification.108 Hydroxypropylated starches (HPS) are known to exhibit enhanced free thaw stability, cold storage stability and high clarity,109 whereas succinylated starches (SS) due to their polyelectrolytic nature possess outstanding swelling ability in cold water, acetylated starches with high degree of substitution behave like thermoplastic materials, although low degree-substituted acetylated starches display excellent freeze thaw stability, lower gelatinization temperatures, improved clarity, binding and film-forming capabilities.38 Carboxymethylated starches (CMS) are quantified to have increased solubility with an increase in the degree of substitution, where the highly substituted ones are soluble even in cold water.110 Xiao et al.111 attempted the chemical modification of Chinese water chestnut starch by acetylation, succinylation and carboxymethylation owing to the presence of a large number of hydroxyl groups in its back bone. The isolation of starch from the corms of Chinese water chestnut was conducted according to the method reported by Wang et al.112 with a few modifications, which was subsequently acetylated, succinylated and carboxymethylated by reaction with acetic acid, succinic acid and chloroacetic acid, respectively.113–115 Subsequently, the physicochemical and structural properties of chemically modified acetylated water chestnut starch (AWCS), succinylated water chestnut starch (SWCS) and carboxymethylated water chestnut starch (CMWCS) were investigated and compared with that of native water chestnut starch. The degree of substitution of AWCS, SWCS and CMWCS was found to be 0.087, 0.575 and 0.972, respectively. The appearance of new peaks in the FT-IR spectra of the modified water chestnut starches substantiated the successful incorporation of new functional groups in the backbone of starch.113,115,116
Similarly, Ansari et al.117 attempted the succinylation and acetylation of water chestnut starch by using esterifying agents, i.e., succinic anhydride and acetic anhydride, and hydroxypropylation and carboxymethylation by employing propylene oxide and chloroacetic acid, respectively, as etherifying agents and all reactions were carried out under alkaline mediums. The degree of substitution in AWCS, SWCS and HPWCS was detected to be 2.4%, 2.5% and 4.1% w/w, respectively (Table 4). In another study, Lutfi et al.23 attempted the chemical modification of WCS by acetylation, acid-thinning and dual modification employing acetylation and acid-thinning of the pregelatinized starch for obtaining pregelatinized acid-thinned and pregelatinized acetylated WCS using the methods proposed in ref. 118.
Table 4 Chemical modifications of water chestnut starch
Types of starch |
Degree of substitution |
Relative crystallinity % |
Thermal properties |
Swelling power (g g−1) |
Solubility (%) |
Pasting properties |
Paste clarity (%) |
Water absorption capacity (%) |
Water retention capacity (%) |
% Functional groups |
References |
Native starch |
ND |
30.6 |
T
o = 52 °C |
19 |
13 |
|
25.1 |
— |
— |
— |
Xiao et al., 2018 111 |
T
p = 57.4 °C |
T
c = 64.6 °C |
ΔH = 9.2 J g−1 |
Acetylated starch |
0.087 |
28.9 |
T
o = 51.4 °C |
28.5 |
23 |
|
28.6 |
— |
— |
— |
|
T
p = 56.8 °C |
T
c = 65.5 °C |
ΔH = 5.2 J g−1 |
Succinylated starch |
0.575 |
29.1 |
T
o = 52.7 °C |
67.3 |
18 |
|
31.6 |
— |
— |
— |
|
T
p = 57.5 °C |
T
c = 70.5 °C |
ΔH = 5.7 J g−1 |
Carboxymethylated starch |
0.972 |
ND |
ND |
89.7 |
35.3 |
|
81.5 |
— |
— |
— |
|
Native starch |
— |
— |
— |
9.6 |
0.17 g g−1 |
|
PT = 72.6 °C |
— |
— |
— |
Ansari et al., 2016 117 |
PV = 264.5BU |
BD = 49BU |
SB = 199.5 BU |
Succinylated starch |
— |
— |
— |
14.3 |
0.72 g g−1 |
|
PT = 61.7 °C |
— |
— |
2.5 |
|
PV = 330BU |
BD = 122.5BU |
SB = 87.5BU |
Acetylated starch |
— |
— |
— |
11.1 |
0.22 g g−1 |
|
PT = 69.1 °C |
— |
— |
2.4 |
|
PV = 330.5BU |
BD = 110.5BU |
SB = 137.5BU |
Hydroxypropylated starch |
— |
— |
— |
8.4 |
0.46 g g−1 |
|
PT = 57.6 °C |
— |
— |
4.1 |
|
PV = 355.5BU |
BD = 239BU |
SB = 93 BU |
Native starch |
— |
— |
— |
50 °C = 224 |
50 °C = 2.8 |
T
g = 77.2 °C |
3.5 |
98.2 |
50 °C = 71.5 |
— |
Lutfi et al., 2017 22 |
60 °C = 261 |
60 °C = 2.8 |
PV = 72BU |
60 °C = 69.8 |
70 °C = 558 |
70 °C = 3.8 |
BD = 0BU |
70 °C = 201.4 |
80 °C = 960 |
80 °C = 5.6 |
SB = 25 BU |
80 °C = 588.5 |
90 °C = 1223 |
90 °C = 5.7 |
|
90 °C = 772.9 |
Acetylated starch |
|
— |
— |
50 °C = 205.1 |
50 °C = 4.3 |
T
g = 72.8 °C |
25.7 |
85.1 |
50 °C = 40.6 |
— |
|
60 °C = 205.1 |
60 °C = 7.8 |
PV = 39BU |
60 °C = 81.4 |
70 °C = 670.1 |
70 °C = 11.3 |
BD = 0BU |
70 °C = 318.1 |
80 °C = 1144.1 |
80 °C = 21.6 |
SB = 27 BU |
80 °C = 822.2 |
90 °C = 1262.1 |
90 °C = 25.4 |
|
90 °C = 1136.1 |
Acid thinning |
— |
— |
— |
50 °C = 222.2 |
50 °C = 7.1 |
T
g = 33.9 °C |
2.4 |
96.06 |
50 °C = 100.7 |
— |
|
60 °C = 224.8 |
60 C = 9.3 |
PV = 7BU |
60 °C = 100.5 |
70 °C = 267.1 |
70 °C = 10.9 |
BD = 0BU |
70 °C = 129.4 |
80 °C = 783.1 |
80 °C = 13.8 |
SB = 0 BU |
80 °C = 537.2 |
90 °C = 986.1 |
90 °C = 19.3 |
|
90 °C = 690.1 |
Pregelatinized acetylated starch |
— |
— |
— |
50 °C = 656.7 |
50 °C = 9.8 |
T
g = 77.2 °C |
3.4 |
366.2 |
50 °C = 406.9 |
— |
|
60 °C = 740.1 |
60 °C = 10.1 |
PV = 72BU |
60 °C = 459.3 |
70 °C = 820.1 |
70 °C = 11.1 |
BD = 0BU |
70 °C = 619.5 |
80 °C = 1058.2 |
80 °C = 15.3 |
SB = 25 BU |
80 °C = 773.1 |
90 °C = 1235.6 |
90 C = 17.1 |
|
90 °C = 822.4 |
Pregelatinized acid-thinned starch |
— |
— |
— |
50 °C = 518.1 |
50 °C = 9.6 |
T
g = 25.3 °C |
1.9 |
315.05 |
50 °C = 350.9 |
— |
|
60 °C = 578.1 |
60 °C = 10.3 |
PV = 5 BU |
60 °C = 379.9 |
70 °C = 653.3 |
70 °C = 9.3 |
BD = 0 BU |
70 °C = 402.3 |
80 °C = 804.6 |
80 °C = 17.1 |
SB = 0 BU |
80 °C = 625.7 |
90 °C = 991.4 |
90 °C = 17.3 |
|
90 °C = 627.6 |
6.1. Impact on morphology
The morphology of native and chemically modified starches as examined by SEM demonstrates oval, polygonal or round starch granules with a particle size ranging from 5–20 μm. In contrast, AWCS and SWCS reveal considerable rough surfaces with small changes in shapes.111 Acetylation causes larger surface erosion, which is attributed to the harsh reaction conditions used in the acetylation process.23 In another study, acetylation and phosphorylation caused irregular concavities on the surface and clustering of starch granules.119 This deformation in AWCS or SWCS may be due to the introduction of functional groups in their backbone.111 However, Ansari et al.117 reported that these chemical modifications hardly affect the shape, size and smoothness of starch granules. The destruction of starch granules resulting from their deformation was reported during the carboxymethylation process. In contrast, dual-modified, acetylated-pregelatinized starch (pgAWCS) exhibited extremely uneven and rough surfaces due to the alteration of the microstructure of water chestnut starch resulting from the changed intra- and inter-granular interactions existing in the starch granules.23
6.2. Impact on swelling power and solubility
Generally, chemically modified water chestnut starch exhibits higher swelling power and solubility in comparison to native water chestnut starch. This difference in the swelling powers of chemically modified and native water chestnut starches reveals differences in their water absorption capacity. The replacement of the hydroxyl groups in native starches with bulkier organic substituents probably reduces the intermolecular forces of attractions existing between starch granules and increases the steric hindrance caused by the hydrophilic acetyl and succinyl groups in the glucan chains of AWCS and SWCS, resulting in the formation of steady hydration of amorphous areas and water percolating into starch granules causing them to swell.111,117
Moreover, a linear relationship existed between the swelling power and solubility of native and modified water chestnut starches in the temperature range of 50–90 °C. In comparison to native water chestnut starch (WCS), a prominent increase in the swelling power of AWCS in the temperature range of 80–90 °C was reported. The incorporation of acetyl group improved the hydrophilicity and played a vital role in increasing the swelling power of AWCS in comparison to native WCS. Remarkably, SWCS displayed higher swelling power than AWCS probably due to much higher degree of substitution of SWCS in comparison to AWCS117 and the bulkier succinyl groups stimulated the starch chains to swell to a larger extent than the relatively smaller acetyl groups.120 Moreover, phosphorylated WCS revealed greater swelling power compared to the native WCS.119
Xiao et al.111 observed that in comparison to AWCS and SWCS, the highest swelling powers and solubility were exhibited by CMWCS, which is probably due to its highest hydrophilicity owing to the amorphous state of carboxymethylated starches.109 However, Ansari et al.117 noticed that hydroxy-propylation reduced the swelling power of WCS, which can be attributed to its higher solubility at elevated temperatures. Chemical modification of starches significantly increases their solubility in comparison to native starch. However, the solubility of SWCS was found to be lower than that of AWCS, indicating that succinylation was not very effective in enhancing the solubility of water chestnut starch.121
6.3. Impact on light transmittance
Chemically modified starches (AWCS, SWCS, CMWCS, atWCS, pgatWCS, and pgAWCS) exhibit better paste clarity in comparison to native starches,23,111 which can be attributed to the presence of swollen granules in water chestnut starch, resulting in the refraction of light to different extents and blurred images.38 Due to the increased steric hindrance and electrostatic repulsions owing to the introduction of bulky functional groups, the re-association of the starch granular chains is prevented, which gives better light transmittance and better clarity to starch pastes. Especially, CMWCS presented the highest paste clarity among the chemically modified water chestnut starches, owing to the introduction of carboxymethyl groups, which facilitate the decomposition of the starch granules.110 Consequently, CMWCS is more suitable than AWCS and SWCS for the preparation of clear beverages and pie fillings.122 Furthermore, both singly chemically modified acid-thinned water chestnut starch (atWCS) and acetylated water chestnut starch (AWCS) as well as dual-modified, pregelatinized acid-thinned (pgatWCS) and pregelatinized acetylated starches (pgAWCS) showed enhanced paste clarity than native WCS. The noticeable increase observed in acetylated water chestnut starch (AWCS) compared to native and other single and dual modifications was principally due to the presence of newly added acetyl groups.23
6.4. Impact on thermal stability
The comparison of the stability of chemically modified starches with native starches can be performed using thermal gravimetric analysis (TGA) curves. Native and AS display two stages of weight loss in comparison to three in the case of SS and CMS. The first stage corresponds to the loss of water molecules, whereas the second stage represents the thermal decomposition of starch itself. In accordance with the TGA curves, the weight loss in various modified starches follow the order of SWCS > Native starch > AWCS > CMWCS, showing that the highest stability is exhibited by CMWCS.111,123
6.5. Impact on pasting properties
The peak viscosities of acid-thinned water chestnut starch (atWCS), acetylated water chestnut starch (AWCS), and dual-modified pregelatinized acetylated water chestnut starch (pgAWCS) and pregelatinized acid-thinned water chestnut starch (pgatWCS) were studied by Lutfi et al.,23 which exhibited a significant reduction as compared to the native WCS (Fig. 5). This can be attributed to the partial cleavage of the glycosidic bonds as a result of chemical modification and the damaged network being more susceptible to shear forces. Moreover, there was a reduction in the time needed to attain the peak viscosities in AWCS, pgWCS and atWCS due to the unhindered penetration of water molecules inside the starch granules. The time taken to reach the peak viscosity further decreased in acid-thinned WCS (atWCS) because of the easier penetration of water owing to the cleavage of longer starch chains into dextrins.
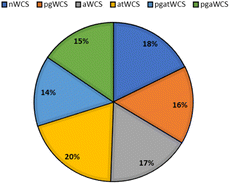 |
| Fig. 5 Freeze thaw stability of nWCS, pgWCS, aWCS, atWCS, pgatWCS, and pgaWCS.23 | |
A similar observation of a decrease in the pasting temperatures of acetylated water chestnut starch (AWCS), succinylated water chestnut starch (SWCS) and hydroxypropylated water chestnut starch (HPWCS) was reported by Ansari et al.;117 however, the peak viscosities were observed to increase with respect to the native starch. Simultaneously, not much difference was seen in the peak viscosities of the differently chemically modified starches (AWCS, SWCS, and HPWCS). Chemical modifications disrupted the inter- and intra-molecular forces existing between the different macromolecules, causing larger percolation of water molecules into the starch granules, and thus an increase in the peak viscosity values. The hot paste viscosity (HPV), which was measured after holding the starch paste for 10 min at 95 °C, was found to be the lowest for HPWCS compared to AWCS, SWCS and native water chestnut starch.117 The lowest hot paste viscosity exhibited by HPWCS may be due to the structural weakening and disruption at elevated temperatures.124 The breakdown viscosity (BD) of starches signifies their instability against heat and shear. In comparison to AWCS or SWCS, HPWCS exhibited the highest breakdown viscosity (BD) value, signifying that etherification causes greater weakening of the starch granular structures in comparison to esterification in the case of AWCS and SWCS.117 However, insignificant changes in the BD viscosities of native starch upon chemical modifications such as acid thinning, acetylation, pregelatinized acid-thinning and pregelatinized acetylation of water chestnut starches have also been reported.23
HPWCS also exhibited lower values of both cold paste (CPV) and set back (SB) viscosities, showing short-term gelation or retrogradation tendency of starch granules on cooling in comparison to other modified and native starches. The decline in viscosity in comparison to native starch during cooling reveals that the short-term retrogradation or reassociation affinity of starches declined significantly in the modified starches.117 Acid thinning also caused a similar decline in the SB viscosity. Starches with lower SB viscosities can be applied in the preparation of puddings and custards, where they can prevent syneresis and provide cold temperature stability.23
6.6. Impact on thermal properties
Acetylated water chestnut starches (AWCS) have a lower onset temperature (To) and peak temperature (Tp) but higher conclusion temperature (Tc) values than the native water chestnut starch, whereas succinylated starch (SWCS) possesses higher values of conclusion and onset temperatures but have the same peak temperature values.111 Both AWCS and SWCS displayed much lower gelatinization enthalpy values in comparison to native starch, signifying that a lower energy is needed to start the gelatinization of AWCS and SWCS.120,123 The probable cause for this is the decrease in the degree of crystallinity exhibited by acetylated and succinylated WCS. However, carboxymethylation results in the total disruption of the crystalline structure of the starch granules, transforming the starch into a total amorphous moiety, which makes it difficult to determine its gelatinisation temperature and enthalpy of gelatinization.110 In addition, phosphorylation disorganizes the molecular arrangement of the double helices in chestnut starch, which leads to a reduction in the gelatinization temperature and enthalpy.119
6.7. Impact on crystallinity
Generally, starch granules can be categorised into A-, B- or C type of crystallinities based on their XRD patterns.125 A-type starch crystals exhibit strong diffraction peaks at 2θ values of around 15° and 23° together with an unresolved doublet at 2θ values of about 17° and 18°. In contrast, crystalline B-type starches show an intense diffraction peak at about 17°, a characteristic peak at the 2θ value of 5.6°, together with a few smaller peaks at 2θ values of 15°, 20°, 22° and 24°. C-type starches are a combination of A- and B-type crystallinities, which can be further classified as CA type and are closer to A type, C type or CB type, while resembling more B-type crystallinities based on the amount of A- and B- polymorphs. A typical C-type starch granule exhibits strong diffraction peaks at 2θ values of around 17° and 23°, CA type shows intense diffraction peaks at 2θ of around 15° and 23° together with a shoulder at 2θ near 18°, which is characteristic of an A-type crystal structure. The XRD pattern of CB-type crystal granules is comprised of a doublet of shoulders at 2θ of around 22° and 24°, in addition to a weak peak at a 2θ of about 15°. Cai et al.30 investigated the XRD pattern of twelve starches isolated from a wide variety of plant sources comprised of corms, tuberous roots, rhizomes, tubers and seeds. The starches isolated from water chestnut were established to have CA-type crystallinities based on their XRD patterns. Due to fact that the crystalline characteristics of A- and B-type crystal starches are not altered by acid modification, they can be exploited for the investigation of the allomorphic distribution of C-type starches.126 The XRD pattern of acid-modified water chestnut starches indicate the disappearance of the characteristic peak at the 2θ value of 5.6°, appearance of a peak at 18°, and sharpening of the peak at 23°, revealing the presence of A-type crystallinities in acid-modified starches.34 This is probably due to the early or faster degradation of the B-type allomorph present in C- or CA-type starches in comparison to their A-type counterpart during acid hydrolysis. The direction of acid hydrolysis of starch granule by HCl is generally from the inner to outer region.126 This change in the crystallinity of acid-modified starches suggests the existence of B-type allomorphs in the interior part of C-type starch granules, accompanied by the presence of A-type allomorphs at the periphery. Xiao et al.111 assigned the A-type crystallinity pattern to water chestnut starches owing to the presence of strong diffraction peaks at 2θ values of 15.36° and 22.63° together with an unresolved doublet at about 17°. The X-ray diffraction pattern of native water chestnut starch exhibited insignificant changes upon acetylation and succinylation. However, the degree of crystallinity of AWCS and SWCS showed a decline due to the destruction of the crystalline regions of starch upon chemical modification. However, CMWCS displayed complete disruption of its crystallinity due to the harsh alkaline reaction conditions of the carboxymethylation reaction.127 However, phosphorylation and acetylation had little effect on the C-type crystalline architecture of starch of water chestnut.119
6.8. Impact on rheological properties
The thickening potential of starches for numerous applications is determined by their rheological properties. Factors such as the chemical structure of starch, pasting and storage conditions and concentration determine the rheological characteristics of starch pastes. The viscoelastic properties of starches are analysed based on their oscillatory rheological parameters including storage modulus (G′), loss modulus (G′′) and loss tangent (tan
δ), which is the ratio of the loss modulus and storage modulus. The viscoelastic properties of starches are significantly affected by chemical modification. Xiao et al.111 reported an increase in the values of storage modulus and loss modulus for SWCS and CMWCS with an increase in frequency from 0.01 Hz to 10 Hz in comparison to the native WCS. Moreover, the relative increase in storage modulus was greater than the increase in loss modulus, indicating that the succinylated and carboxymethylated water chestnut starches exhibited greater elasticity and less solid/gel-like characteristics.128,129 An increase in temperature had an inverse effect on the viscoelastic parameters (G′ and G′′), which declined with an increase in temperature from 25 °C to 85 °C. The tan
δ value was never greater than one, signifying that all the modified starches (AWCS, SWCS and CMWCS) displayed solid-like behaviour even at elevated temperatures. The viscoelastic properties of chemically modified water chestnut starches increased in the order of SWCS > CMWCS > native WCS > AWCS. The superior gelatinization behaviour of succinylated and carboxymethylated water chestnut starch pastes increases their applicability as thickening agents in hot beverages.130
6.9. Impact on water retention and water absorption capacity
As reported by Lutfi et al.,23 the water retention capacity (WRC) of modified WCS significantly increased in comparison to native WCS when heated from 50 °C to 90 °C. Acetylated water chestnut starch (AWCS) and pregelatinized acetylated water chestnut starches showed a remarkable enhancement in water retention capacity with an increase in temperature from 50 °C to 90 °C. The accessibility of the water binding sites due to the disruption of the hydrogen bonds at elevated temperatures may be the reason for the increase in the WRC of these starches.
The water absorption capacity of dual-modified pgAWCS substantially increased in contrast to the native water chestnut starch.23 The observed increase can be attributed to the electrostatic repulsions between the starch molecules, enabling the easy penetration of water molecules in the matrix. In contrast, AWCS and atWCS exhibited a decrease in WAC due to the lower availability of binding sites in the starch granules for water molecules.131
6.10. Impact on freeze-thaw stability
Lutfi et al.23 reported that the freezing of starch gel leads to the creation of starch-rich areas in the matrix and presence of unfrozen water in the matrix. Subsequently, syneresis occurs in the starch gel upon thawing and leaves it with a sponge-like form. In the case of acetylated starch, the acetyl groups present on the amylose chain hindered the re-joining of the starch chains and formation of a proper gel network. Acid-thinned starch exhibited lower strength, which may be due to the less intermolecular binding forces among the low molecular weight starch chains. Acid-modified starch from water chestnut showed greater freeze-thaw stability, and thus can be utilized in the formation of frozen food products and non-food industrial applications.
7. Nanoparticle formation with water chestnut starch
Currently, the synthesis of starch nanoparticles (SNPs) has revolutionised starch-related research owing to their wider applications in the bio-medical field as nanocarriers, in water treatment plants, catalytic nanosystems and food industry. SNPs have gained considerable attention due to their distinctive functional properties such as nano-meter size, non-toxic nature, better biocompatibility and biodegradability, increased surface area and excellent absorptivity in comparison to its natural form.132,133 Starch nanoparticles can act as fillers in starch composites and exhibit exceptional mechanical properties and biodegradability, which may be of significance in the future for packaging purposes.134 Recently, naturally occurring starch polymers have also been employed in the synthesis of nanostructured materials associated with excellent biocompatibility and biodegradability in various fields such as drug carriers and agricultural and packaging applications.135 Starch has also been utilized in the preparation of biodegradable and non-toxic edible coatings and films that contain antimicrobial agents for increasing the shelf life of food products, preventing rancidity, moisture loss and flavour loss from food products.136 Dularia et al.29 synthesized a water chestnut starch (WCS) film via the solution casting method and nano water chestnut starch-based composite films by adding native WCS to nano starch (5% w/w, dry basis) in varying concentrations including 0.5% w/w, 1% w/w, 2% w/w and 10% w/w. The thickness and flexibility of the nanocomposite films increased with an increase in the concentration of WCSNPs. Alternatively, the moisture content of the native starch film was found to be the maximum, which decreased with an increase in the concentration of WCSNPs to starch for the formation of composite films. The water vapour transmission rate (WVTR) decreased from 4.678 × 10−3 ± 0.42 g m−2 s−1 in native starch film to 2.221 × 10−3 ± 0.42 g m−2 s−1 in nano starch composite film at 10% concentration of WCSNPs. This decrease in WVTR in the composite film is possibly due to the decrease in the size and increase in the surface to volume ratio, which inhibited the mobility of the polymeric chains and increased the compactness of the composite film, thus increasing the water vapour resistance of the film.137 The native water chestnut starch film exhibited the highest solubility in comparison to the nano starch composite films containing different concentrations of WCSNPs.29 The burst strength of the water chestnut starch-based composite films increased with an increase in the concentration of WCSNPs, whereas the native WCS based film exhibited the lowest burst strength. The nano starch-based composite film at 10% w/w concentration required the maximum amount of force to burst it (1488.5 ± 12.14 g) in comparison to 976.4 ± 12.47 g for the native water chestnut starch film.
Numerous methods have been reported for the preparation of starch nanoparticles (SNPs), such as acid hydrolysis, enzymatic hydrolysis, nanoprecipitation, ultrasonication, physical methods including reactive extrusion, high-pressure homogenization, stirred media milling and gamma irradiation, and the combination of methods such as heat-moisture treatment (HMT) and homogenization under mild acidic conditions as well as HMT under mild acidic conditions.138–143 The preparation of SNPs by physical methods is more advantageous compared to other chemical methods given that it is safer and more environment friendly. A combination of nano-precipitation and ultrasonication acts is considered a simple, reliable, effective and environment-friendly method, which involves the slow addition of a dilute solution of starch to a solvent, and then breaking of the covalent bonds of starch due to the strong shear forces or mechanical forces of sound waves.142,144,145 SNPs can also be prepared via the ultrasound method in the absence of chemical additives. However, to obtain nanoparticles with desirable properties, a combination of physical and chemical methods is more desirable.132 Ahmad et al.146 prepared SNPs from water chestnut starch (WCS) via the ball milling process, resulting in the modification in the crystalline structure and morphology of the starch granules.147–149 In another study, Ahmad et al.28 prepared water chestnut starch nanoparticles (WCSNPs) via a combination of alkaline hydrolysis under mild reaction conditions and ultra-sonication method, which served as a simple, trustworthy and environment-friendly method for synthesizing nanoparticles with a desirable size (Table 5).
Table 5 Nanoparticle modifications of water chestnut starch
Type of starch |
Water absorption capacity |
Oil absorption capacity |
Average particle size (nm) |
Polydispersity index |
Zeta potential (mV) |
Relative crystallinity (%) |
Thermal characteristics |
Water vapour transmission rate (g m−2 s−1) |
Swelling power (g g−1) |
References |
Native water chestnut starch |
1.06 |
1.21 |
— |
— |
— |
26 |
— |
— |
— |
Ahmad et al., 2020 28 |
Water chestnut nanoparticles |
1.32 |
1.09 |
271.5 |
0.378 |
−20.45 |
7.96 |
— |
— |
— |
Water chestnut nanoparticles |
— |
— |
535.21 |
0.37 |
−41.29 |
5.56 |
T
0 = 52.76 °C |
— |
— |
Ahmad et al., 2020 |
T
c = 134.15 °C |
T
p = 110.23 °C |
Native starch |
— |
— |
— |
— |
— |
26 |
T
0 = 49.26 °C |
— |
— |
T
c = 124.23 °C |
T
p = 87.68 °C |
Native starch composite film |
65.2% |
25.1% |
5559 |
— |
— |
— |
— |
4.673 × 10−3 |
— |
Dularia et al., 2019 29 |
Nanostarch composite film |
— |
— |
396 |
— |
— |
— |
— |
2.221 × 10−3 |
— |
Nain et al.,150 in a separate study, reported the synthesis of starch nanoparticles from different botanical sources such as water chestnut, wheat, potatoes, mango kernels and mung beans and nanocomposites of isolated starches by coupling SNPs with zinc oxide (ZnO). The same research group also explored the biocompatibility of nanocomposites of ZnO-coupled water chestnut starch against human HeLa cells, and their cell viability was ascertained using the MTT [3-(4,5-dimethylthiazol-2-yl)-2,5-diphenyltetrazolium bromide] assay. The nanocomposites of WCS were found to be biocompatible to HeLa cells and non-toxic in nature. Therefore, they can potentially be used as drug delivery vehicles. The morphological, thermal, rheological and functional properties of starch nanoparticles exhibit remarkable changes in comparison to native starch.
7.1. Impact on particle size and zeta potential
Ahmad et al.146 reported that water chestnut starch nanoparticles had an average size of 271 nm, polydispersity index (PDI) of 0.378 and zeta potential of −20.45 mV. In another study, Ahmad et al.28 reported that WCSNPs had an average particle size of 535.21 nm, PDI of 0.37 and zeta potential of −41.29 mV. The large negative zeta potential value for WCSNPs signifies the presence of negative charges on their surfaces and electrostatic repulsive interactions between the starches, thus endowing them with stability and hindering their agglomeration due to the reduced van der Waals forces of attraction.149 Nain et al.150 observed that the particle size and distribution of ZnO-coupled nanocomposites of water chestnut starch was in the range of 506–1209 nm.
7.2. Impact on water and oil absorption capacity
The water absorption capacity (WAC) and oil absorption capacity (OAC) of water chestnut starch was found to be 65.2% ± 0.25% w/w and 25.1% ± 0.10% w/w, respectively.29 The WAC exhibited an increase with a decrease in the size of WCSNPs, as reported by Ahmad et al.146 The WAC of water chestnut starch showed an increase from 1.06 to 1.32 after ball milling. In contrast, its oil absorption capacity (OAC) showed the opposite trend, where it decreased from 1.35 to 1.15 after ball milling. The WAC exhibited an increase possibly due to the increase in surface area and exposure of the water-loving binding sites of starch granules produced by the disruption of amylose and amylopectin chains on ball milling.51 Ahmad et al.28 reported that WCSNPs showed a much higher WAC than the native starch, whereas the OAC of these nanoparticles was much lower than that of the native unmodified WCS. The alkaline hydrolysis and ultrasonication conditions used in the preparation of WCSNPs break or reduce the crystalline regions of amylopectin, and also permit the hydrogen bonding sites created by cleavage of intermolecular bonds of starch to interact with a greater number of water molecules, thereby increasing their WAC. Similarly, the OAC decreased upon ball milling owing to the reduction in capillary forces caused by the disruption of the water chestnut starch granules.151 According to Ahmad et al.,28 the decrease in the oil absorption capacity of WCSNPs was because of their smaller sizes and the variations caused in the polymeric framework, which affected the hydrophobicity of the water chestnut starch nanoparticles.
7.3. Impact on morphology
The micrographs of native water chestnut starch particles as taken by SEM indicated oval/round shapes together with smooth/porous surfaces.28 Forces such as shear, compression and impact used in the ball milling process make the granules smaller with cracked and broken surfaces.28,152 A couple of starch granules also adhered to each another. WCSNPs formed by the combined effect of alkaline hydrolysis and ultrasonication were smaller in size and had eroded surfaces containing grooves with broken cell walls.153 The SNPs had irregular shapes and no uniform pattern in their sizes. However, the native WCS granules were observed to have smooth polygonal surfaces and size in the range of 2 to 5 μm.28 In contrast, Dularia et al.29 monitored the different shapes of native and nano starch granules employing field emission-scanning electron microscopy (FE-SEM). The WCS granules were found to have oval, ellipsoidal and spherical shapes with smooth and crack-free surfaces. In contrast, water chestnut starch nanoparticles (SNP) having diameters of 100 μm and 10 μm were agglomerated and had rough and irregular surfaces. It was also reported by Sun et al.130 that starch nanoparticles having average diameters in the range of 10–700 nm exhibit irregular morphologies.
Nain et al.150 observed that morphologically, water chestnut starch-coupled nanocomposites existed as agglomerates of irregular shapes. The agglomeration resulted from the initial complex formation of starch with divalent Zn2+ metal ion. This was followed by the formation of supramolecular assemblies, supplemented by the formation of intramolecular and intermolecular hydrogen-bonds, which served as a template for NP growth.
7.4. Impact on crystallinity
The XRD diffraction patterns of native water chestnut starch and starch nanoparticles were compared. Both the native WCS and WCSNPs were observed to possess an A-type crystalline pattern. The size reduction in ball milling was accompanied by a decrease in peak intensity and decrease in relative crystallinity (RC) from 26.16 to 7.98 in the water chestnut starch nanoparticles. The decrease in relative crystallinity is probably due to the formation of a larger proportion of amorphous regions upon the breakdown of the crystalline regions of amylopectin during ball milling. Starch molecules generally exist in two crystalline forms, i.e., A and B. The crystalline form A is a monoclinic unit cell associated with 8 inter-helical water molecules inside it, whereas the crystalline form B is an open packed structure containing 36 interhelical water molecules.146,150 The larger number of water molecules in the latter makes it resistant to mechanical stress, as reported by Tan et al.154 Thus, the crystalline form A is damaged upon ball milling. However, in another study, Ahmad et al.28 observed a decrease in RC from 26 in native WCS to 5.56% w/w in water chestnut starch nanoparticles. The decrease in RC was attributed to the increased amorphous content of starch in the small-sized starch particles, with diminished or narrow diffraction peaks shown by the nano-sized water chestnut starch particles.
7.5. Impact on thermal properties
Ahmad et al.146 investigated the thermal properties including phase transitions of starch nanoparticles (SNPs) of water chestnut starch by differential scanning calorimetry (DSC). The starch nanoparticles of water chestnut exhibited a broad endothermic peak at 85.51 at a higher transition onset temperature (To) compared to the native starch particles, which is associated with the melting of the smaller crystallites. The increase in the transition temperature of SNPs can be attributed to their smaller granular size because of the ball-milling process. Similar reports of an increase in gelatinisation temperature with a decrease in granular size are also available in the literature.155
Comparatively, Ahmad et al.28 performed the thermal characterization of native starch and starch nanoparticles from different plant varieties and significant differences in initial temperature (To) and final temperature (Tc) were observed. WCS and WCSNP exhibited peaks between 49.26–124.23 °C and 52.76–134.15 °C, with the peak transition temperatures at 87.68 °C and 110.23 °C, respectively. The sharp peak at 135.80 °C for water chestnut starch nanoparticles (WCSNP) well established their crystalline nature. However, the lower transition temperatures for water chestnut native starch can be attributed to its gelatinization enthalpy. They also concluded that ultrasonication disrupted the crystalline character of amylopectin clusters and led to increased stability of hydrogen bonds and van der Waals forces of attraction. Subsequently, the melting points increased because of the increased hydrocarbon chains, which led to reduced crystallinity in WCSNPs.
7.6. Impact on rheological properties
The influence of starch granular size on rheological properties was reported by Ahmad et al.146 The reduction in the size of WCSNP upon ball milling demonstrated the solid-like behaviour of all the isolated nanoparticles in contrast to the gel-like behaviour of native starch. The viscoelastic behaviour of WCSNPs was evaluated after the reduction in their size. WCSNPs were found to have higher viscosity than the native starch. The possible reasons for this behavioural transformation can be the variation in surface area, charge, distribution of granules, amylose content and interaction between the molecules.
Ahmad et al.28 compared the storage modulus (G′) and loss modulus (G′′) of water chestnut starch nanoparticles (WCSNPs) with native unmodified starch as a function of frequency. The values of storage modulus (G′) were much higher than the loss modulus (G′′) values and the difference between these two values indicated that the gel-like behaviour of the WCSNPs increased with an increase in frequency. Also, WCSNPs exhibited more solid-type behaviour in comparison to the native starch. The water chestnut starch nanoparticles showed much higher viscosities compared to native starch, confirming the effect of the size of the granules on the rheological profile of starch. The possible reasons for this behavioural transformation can be the variation in surface area, charge, distribution of granules, amylose content and interaction between the molecules.
7.7. Structural elucidation of water chestnut starch nanoparticles by infrared (IR) and energy dispersive X-ray spectroscopy (EDX)
The structural elucidation of native starch and water chestnut starch nanoparticles (SNP) by attenuated total reflectance Fourier transform infrared (ATR-FTIR) spectroscopy was performed by Ahmad et al.,146 displaying similar IR peaks for O–H stretch, –CH2–, C–O, C–O–H and C–O–C vibrational modes of anhydrous glucose ring, and the characteristic IR peak at 1643 cm−1 signifies the presence of water molecule attached to starch; however, insignificant changes in intensity after size reduction were also observed.156 The IR peaks in the range of 3200–3300 cm−1 signify the O–H stretching frequency, whereas the bands in the range of 2920–2933 cm−1 indicate the –CH2– stretching frequency.28 Additionally, the shift in the O–H stretching peak towards a higher wavelength for WCSNPs can be ascribed to the loss of crystallinity and exposure of their O–H groups on the addition of alkali and ultrasonication.157–159 The existence of β-glycosidic linkages in WCSNPs was ascertained by the presence of characteristic absorption peaks at 855, 856 and 867 cm−1.160,161 FTIR spectroscopy was helpful in determining the crystallinity of starch by exploring the changes in the IR bands in the semi-crystalline and amorphous areas of starch granules. Moreover, the impact of the addition of alkali and ultrasonication on the physico-chemical properties of starch was further corroborated by the decrease in the ratio of absorbance bands at (1047/1018) and (995/1018) cm−1 after a reduction in its size, implying a decrease in its crystallinity and generation of an amorphous phase. However, Ahmad et al.146 observed a similar decrease in the ratio of the IR absorption bands at (1047/1018) and (995/1018) cm−1 with a decrease in the size of WCSNPs due to the ball-milling process. Nain et al.150 confirmed the presence of starch nanoparticles by energy dispersive X-ray spectroscopy (EDX) based on the appearance of peaks at 0.3 keV and 0.6 keV, signifying the existence of C and O respectively. Additionally, the existence of Zn as coupled molecule was also ascertained by EDX studies.
8. Application concerns
8.1. Food utilization
Starch is a macro-constituent in many foods, and thus its properties and interactions with other constituents are of interest in the food industry and for human nutrition. It is widely used as a food additive to maintain the consistency and smooth texture of sauces and soups. Moreover, starch is employed to hamper the breakdown of gels during processing and to enhance the shelf-life of food products. The bakery industry in India is considered one of the largest food processing industries and is ranked as the second biggest manufacturer of biscuits after the USA. Celiac disease is an autoimmune reaction caused by the interaction of gluten in a genetically inclined population,162 which is very frequent in North India where wheat is a staple food.163 In this case, only gluten-free food can restore their health completely and better the quality of life in patients with celiac disease.164 Therefore, there is a requirement for the creation of a wide range of gluten-free products, and the number of these products is increasing worldwide with the increase in the number of individuals diagnosed with celiac disease.165
Water chestnut (Trapa natans) is employed as an alternative food to cereals in the Indian subcontinent during fasting and it can be a better substitution for wheat flour (WF) regarding Celiac disease caused by the difficulty in the digestion of gluten (wheat protein).27,166 Cookies made from water chestnut flour are used as a specialized dish during Navratri and other fasting days in India. Water chestnut flour (WCF) is devoid of gluten and contains a high content of starch, which makes this product promising for the development of gluten-free bakery products. Moreover, it has a high fiber content and multiple nutritional values, which authenticates its use in the foods. Cookies made from water chestnut show a greater spreading ratio in comparison to WF cookies, causing an increase in the demand for water chestnut flour. This may be due to the higher quantity of starch present in water chestnut flour.27,166,167 Lutfi and Hasnain168 investigated the effect of modified water chestnut starch on the volume index, symmetry index, and uniformity index of sponge cake, which revealed that the addition of acetylated starch boosted the volume index of sponge cake to a remarkable extent. Acid-thinned starch at 1% w/w concentration caused an increment in the symmetry index of sponge cake. At 4–5% w/w concentration, pregelatinized and acid-thinned starch exhibited an excellent uniformity index. In another investigation, Malik et al.169 studied the physicochemical and sensory parameters of yogurt supplemented with water chestnut starch and compared them to that of yogurt enriched with stabilizer gelatin (0.5% w/w). The use of water chestnut starch gave good outcomes because of the decreased syneresis and enhanced water carrying capacity, viscosity, and all-inclusive appropriateness for all sensory characteristics. Water chestnut starch showed no effect on the taste and quality of yogurt. It was observed that water chestnut starch at 1.25% w/w and 0.75% w/w displayed efficient results for water holding capacity, synergies, viscosity, and all sensory attributes. Moreover, it was found that the shelf-life of yogurt was enhanced by up to four weeks.
Starches play a vital role in food and industrial applications because of their condensing ability. Water chestnut starch is employed in several foods to enhance their textural qualities, such as sustaining sauces and soups. The amylose content of water chestnut starch is 22.3% w/w, which it is firmly associated with amylopectin. However, it is not employed as a stabilizing agent in milk products.25 The starch purified from water chestnut (Trapa bispinosa Roxb) showed a moisture content of 7–8% w/w, protein content of 0.21–0.22% w/w, ash content of 0.03–0.04% w/w, carbohydrate content of 81–85% w/w, and energy of 385 calories per 100 g. Isolated starch was added to dahi at various concentrations and it was found that there was a remarkable alteration in the attributes of dahi. The addition of water chestnut starch to dahi caused alterations in its physical, chemical, and sensory properties based on the concentration incorporated. It was observed that water chestnut starch as a stabilizer in dahi exhibited proficient results in view of the decreasing syneresis and acceptability, even producing creamy and adhesive dahi. The incorporation of water chestnut starch revealed no adverse effects on the taste and quality of dahi. Water chestnut starch gave good results regarding pH, acidity, syneresis, and all sensory attributes. It was found that the dahi could be used for about 1 month at cold temperatures. Water-chestnut starches (native and modified) can be used as a fat alternative in mayonnaise and may remarkably decrease the calorie load given that carbohydrates possess half the calories of fats. Mayonnaise consisting of succinylated water-chestnut starch was observed to be better in view of sensory and textural properties compared to the mayonnaise containing acetylated starch.117
New bio-based packaging materials such as edible and biodegradable films have been employed for managing environmental issues and enhancing the shelf-life of food. This packaging can be ingested together with the food, giving extra nutrients, increasing sensory characteristics and quality. An edible film with microbicidal agents is one of the ways to stop the contamination of microbes on the surface of food products.170–172 Starch films and coatings have been employed for several food and medicinal applications. The film-forming capability of water chestnut starch shows a worthy future for exploration as a packaging material for food and non-food purposes.19 Mei et al.173 conducted research on improving the microbicidal activity of water chestnut starch–chitosan (WSC) films via the addition of C. officinalis extract (COE), glycerol monolaurate (GML), nisin, and pine needle essential oil (PNEO). The addition of COE reduced the pH value of the film-preparing solution, the water content, and the water absorption expansion ability (WAEA). The GML-incorporated film showed a lower WAEA, tensile strength, elongation, and puncture strength. In contrast, the nisin films revealed strong mechanical properties. All the treated films displayed lower transparency and greater water vapor permeability values. The pine needle essential oil (PNEO) caused discontinuities with the lipid droplets or holes embedded in a continuous network and the addition of GML led to abaisse-like structures. The COE, GML, nisin, PNEO, and their combinations incorporated in WSC films were observed to be potent in hindering the growth of Escherichia coli, Staphylococcus aureus, and Listeria monocytogenes at various concentrations. In another study, a biodegradable composite film was prepared using water chestnut starch and polyvinyl alcohol. It was observed that the addition of plasticizer caused a reduction in the swelling power of the films; however, it enhanced the solubility of films. The tensile strength of the composite film was found to be greater at a low concentration compared to a higher concentration of plasticizer, where the tensile strength of the film was found to be low. Furthermore, the use of plasticizers resulted in an enhancement in the biodegradability of films and made them superior for food packaging without any harmful consequences.174 Moreover, Lai and Wong175 prepared clusteroluminogenic films of starch from maize, potatoes, and water chestnut by solution casting. All the films showed homogeneity with a width of 55–65 μm. The Water chestnut starch films revealed the maximum proportion of transmittance compared to the potatoes and maize. There was no marked variation in the clusteroluminescence intensity when a starch-based packaging bag was utilized for the packing of fresh or frozen food. However, a significant decrease in the luminescence intensity was observed upon the thawing of the frozen food in the bag. This study depicted the probability of utilizing the clusteroluminescence of starch as a pointer to expose the condition of packed frozen food.
8.2. Textile industry
Starch is used in its raw state or processed by fermentation with bacteria to give bio-origin monomers, which are polymerized into bioplastics. Starch is employed in fine fabrics and the cosmetic industry. Moreover, it is used for increasing the strength of paper and printing characteristics in the paper industry and binder in the packaging industry.176,177 In addition, currently, bioplastics are made from plant-origin raw materials, such as starch. Gupta178 reported the use of starch-origin bioplastic as a packaging material. Pilla179 investigated the use of bioplastics, which is becoming widespread in the packaging sector, biomedical field, automotive industry, civil engineering, and general engineering, but mainly in consumer goods. There are two main significant facts regarding bio-origin plastic derivatives compared to their conventional versions. Firstly, they avoid the use of fossil sources because of the use of biomass, which gives the distinctive ability of carbon neutrality. Secondly, bioplastics play a major role in the decline of food waste, CO2 emissions, and dependency on fossil raw materials and CO2.180 Starch from water chestnut, Manihot esculenta, cornflour, and rice showed a yield of bioplastics of 12 g, 9 g, 8 g, and 11 g respectively.181 Starch blends derived from potatoes and water chestnut were used in water-soluble chips as spacers to save the contents of the packages and other expanded materials as an alternative to polystyrene, shopping bags, bio-waste storage bags, food packaging, and medical and cosmetics products.182
8.3. Pharmaceutical innovations
Starch is one of the most strongly demanded pharmaceutical excipients worldwide due to its abundance, biostability, and multi-faceted behaviour. In this case, maize and potato starch are the most commonly used excipients in tableting, while many scientists have attempted to design several botanical starches as tablet excipients.183–186 Binders are the compounds that provide the required adherence among the granules for adequate bonding during squeezing. The addition of binders to the formulation of a tablet gives plasticity, and hence enhances the inter particulate bonding among the tablet granules, and also boosts the rate of compaction; however, they reduce the brittle fracture liability during tableting.187 In previous studies, some researchers determined their disintegration potential in tablet dosage form.188 Diclofenac was used as the standard drug, which is used as a potent anti-inflammatory and pain-relieving agent in the cure of arthritis and acute injury. Singh et al.189 aim to determine the binding potential of water chestnut starch in diclofenac sodium-based tablets and also compare it with native starch. The diclofenac tablets formed with a greater concentration (15% w/w) of starch revealed an increase in disintegration time value, which can be due to the low diffusion of the solvent in the tablet pores due to the formation of robust bonding among the granules. The dissolution of a drug in the stomach environment is also indirectly based on the disintegration time of the tablet formulation. It was found that the drug dissolved fast at a low starch concentration, whereas at higher concentrations, the native starch resulted in the formation of a viscous mass, which hindered the seepage of the dissolution medium into the pores of the dosage form. The diclofenac sodium tablets formed with the chestnut starch exhibited favourable and comparable characteristics such as firmness, durability, high drug content, disintegration, weight variation, and dissolution compared to the native starch binders. Therefore, it was observed that water chestnut starch (T. bispinosa) displays excellent quality to be employed as a natural binder in pharmaceutical formulations. Similarly, Venkatesh et al.190 studied starch extracted from water chestnut as a substitute pharmaceutical excipient to maize starch. The tablets were designed by dry and wet granulation using paracetamol and aspirin, respectively, with water chestnut starch. Furthermore, maize starch was employed as an alternative excipient for comparison by conducting a series of pre-formulation and formulation tests. Pre-formulation studies such as flow properties, sieve analysis, micrometry, moisture content, swelling index, and compatibility studies were performed. The results observed for water chestnut (T. bispinosa) starch were found to be comparable with maize starch, indicating that it can be employed in the preparation of tablets as a pharmaceutical excipient. Similarly, Rao et al.191 monitored the starch extracted from water chestnut (Trapa natans) as a binder in the preparation of tablets. Diclofenac granules were made by wet granulation and starch was isolated from Trapa natans by maceration. The physicochemical characteristics of Trapa natans were determined and the pre-compression characteristics such as tapped density, bulk density, and Carr index revealed that the diclofenac granules formed by using Trapa natans were detected to be in the limits and similar to that formed by using native starch. Their post-compression attributes such as hardness, weight variation, disintegration, thickness, and dissolution were estimated. Water chestnut starch formulations of diclofenac showed greater hardness in comparison to the native starch. A significant increment in disintegration time was observed in the tablets prepared with the water chestnut starch in contrast to the native starch. In another study, Bae et al.192 made biodegradable films by using starches from different herbal sources, and their physical and mechanical characteristics were investigated. Among the examined samples, the potato, mung-bean, and water chestnut starches showed comparatively better mechanical and physical characteristics in contrast to the gelatin and HPMC (hydroxy propylated methyl cellulose) films. The starch films displayed better O2 barrier and enhanced H2O blockade characteristics in comparison to the gelatin and HPMC films. The water chestnut starches were employed in the design of hard capsules for the pharmaceutical industry. These capsules showed efficient molding characteristics and high quality. The starch capsules were observed to be clear, with a plain surface, and not rigid. In addition, the antioxidant activity of water chestnut starch (WCS) and its nanoparticles (WCSNP) was investigated by the DPPH scavenging assay. The DPPH radical scavenging potential of WCS and WCSNP was observed to be 17% w/w and 19.6% w/w. According to the literature, low molecular weight and monosaccharide compositions can play a major role in the antioxidant potential of polysaccharides.193 Many studies indicated that starch consists of flavonoids, which show strong radical scavenging potential.194,195 Ahmad et al.146 also demonstrated that WCSNPs displayed greater inhibitory activity against lipid peroxidation in comparison to WCS. Similarly, the curative activity of enzymatically (amylosucrase) modified WCS was evaluated in a murine model of obesity. Modification of starch by amylosucrase enhanced the percentage of resistant starch, making it inaccessible for catalysis by minor-intestinal mucosal glucosidase. The amylose/amylopectin ratio in chestnut starch was boosted through enzymatic modification. Amylosucrase-modified chestnut starch at the dosage of 1500 mg kg−1 per b wt was given orally for ten weeks to high-fat diet stimulated obese mice. It was observed that the modified chestnut starch caused a marked reduction in obesity in the mice in comparison to the control group. However, it was observed that the administration of modified chestnut starch in the mice showed a remarkable increment in cecum weight, depicting the secretion of fatty acid in the cecum. Moreover, the modified starch activated the fatty acid receptor GPR43-mediated annihilation of carbohydrate signaling (especially insulin). Thus, amylosucrase-modified starch enhances the indigestible-resistant starch and it mitigates food-stimulated obesity by the GPR43-induced decline of carbohydrate signaling, therefore causing a reduction in the accumulation of fat in white adipose tissue (Fig. 6).196 In another investigation, acetylated WCS showed a greater percentage of resistant starch (81–84% w/w) in comparison to the native WCS (58% w/w) and phosphorylated WCS (62–76% w/w). However, the amount of rapidly and slowly digested starch was found to be low in the acetylated and phosphorylated WCS. Moreover, dry heat treatment with XG of WCS showed a marked quantity of resistant starch in contrast to that by dry heat treatment or XG alone. Resistant starch can be utilized in various foods to lower the caloric value and restrain the cases of diabetes.119,197
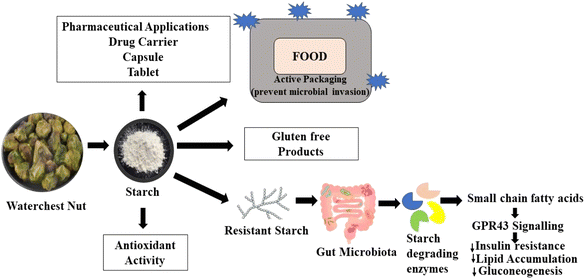 |
| Fig. 6 Applications of water chestnut starch. | |
8.4. Miscellaneous utilization as emulsions and delivery system development
In encapsulation, the inner material is filled in the socket of the wall material, which serves to save its inner components from physical, chemical, and biological degradation.142 Resveratrol is widely employed for encapsulation in several small polymeric materials such as hyaluronic acid, pectin, cyclodextrin, gelatin, albumin, gliadin, and chitosan.198–200 Recently, researchers have focused on finding economic and easily available wall materials for encapsulation. Thus, the efficiency of starch extracted from horse chestnut and water chestnut as encapsulation material was determined. The starch from these herbs will be more appealing in the future at the financial level given that it is consistent with the need for application as a food ingredient due to its gross existence at a lower price and the constitution of starch. Starch nanoparticles of resveratrol (HCSR and WCSR) enhanced the biopotential under imitated gastrointestinal environment. These nanoparticles were designed by employing the ultrasonication method without the use of any chemicals, and then screened for their encapsulation potential, zeta potential, and particle size. SEM, XRD, ATR-FTIR, and DSC methods were used for the characterization of the resveratrol–starch nanoparticles. The release nature and potential of the unbound and nano-encapsulated starch resveratrol were also investigated against diabetes and obesity. The size of HCSR and WCSR was 419 and 691 nm with a zeta potential of −16.09 and −15 and encapsulation ability of 81.5% and 79.4% w/w, respectively. The nanoparticles exhibited a film-like or porous configuration with reduced crystallinity and greater thermal transformation temperatures. The enhancement in thermal transformation temperatures indicates the efficient stability of the nano-encapsulated resveratrol. The protective efficacy of HCSR and WCSR against diabetes and obesity was monitored by determining the % inhibition of pancreatic lipase (PL), α-glucosidase, and cholesterol esterase enzymes (CE). The inhibition of pancreatic lipase was found to be 47% and 52% by HCSR and WCSR, respectively, after mimicking the gastrointestinal environment. Moreover, the percentage inhibition of cholesterol esterase was detected to be 76% and 73.2% by HCSR and WCSR, respectively, in the gastrointestinal environment. However, the free resveratrol displayed a decreased % inhibition for PE and CE, which was observed to be 26% and 53%, respectively, under the same gastrointestinal environment. This depicted the efficient anti-obesity efficacy of the nano-encapsulated form of resveratrol. Both formulations of resveratrol showed a similar pattern for the hampering of α-glucosidase. The proportion of suppression of α-glucosidase from HCSR and WCSR was observed to be 45% and 40.4%, respectively, in comparison to the free resveratrol molecules, where it was found to be 23% (Fig. 7). The PL and CE enzymes play a vital role in the control of fatness,201 and the inhibitory potential of resveratrol is associated with its high antioxidant properties.202 The nano-encapsulated resveratrol displayed high inhibitory potential against cholesterol esterase compared to PL and α-glucosidase. This depicted the restoration of the potential of resveratrol in the nano-encapsulated form in contrast to the free form. Thus, the starch chestnut nanoparticles showed better nutraceutical potential and may be used to avoid the degradation of resveratrol from the outmost environment and to enable its absorption in the colon in its pure form.203 Previous studies also displayed similar results with the nanoencapsulation of starch catechin nanoparticles.142 Thus, it can be concluded that nano-encapsulation may be a useful way to circumvent the limitation of bioavailability by employing new starch-based nanoparticles. The nanoparticles showed good bioaccumulation and cell penetrability and may protect the catechin during digestion when mixed with functional foods. It was observed that horse chestnut starch (HSC) revealed the maximum encapsulation efficiency and reduction in particle size. Alternatively, water chestnut starch (WSC) had the highest stability and also allowed the slower release of catechin from its core. Nano-encapsulation using starch-based nanoparticles protected catechin against the harsh gastric environment and helped in retaining its bioactive properties during the in vitro digestion process. Hence, we suggest that HSC and WSC are more suitable for nanoparticle preparations and the encapsulation of catechin.142
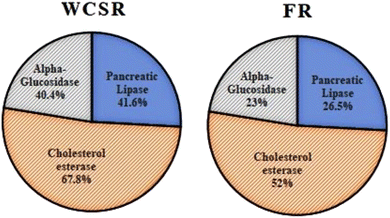 |
| Fig. 7 Bioactivity of free encapsulated and nano-encapsulated resveratrol under simulated gastrointestinal conditions.203 | |
The cytotoxic potential of water chestnut starch extract was determined on the macrophage RAW 264.7 cell line and fibroblast NIH 3T3 cell line. In addition, the acute dermal toxic effect of water chestnut starch was checked in rats at a single dose of 200, 1000, and 2000 mg kg−1 per b wt, and its oral toxic potential was monitored in rats at the concentration of 300 and 2000 mg kg−1 per b wt The in vitro results revealed that water chestnut starch was nontoxic towards macrophages and fibroblasts at the dosage of 6.3–200 μg mL−1. The acute dermal and oral toxicity studies indicated that the use of water chestnut starch is safe and showed no adverse effects up to a concentration of 2000 mg kg−1 per b wt in animals. Thus, water chestnut starch can be employed as a pharmaceutical excipient or ingredient in cosmetic products. However, its long-term toxic effects should be studied to detect its chronic safety upon continuous consumption.204 In another investigation, the cytotoxic potential of nanoparticles of WCS against HeLa cells was determined using the MTT assay. After 52 h, 92% cells were found to be viable among the cells treated with nanoparticles of WCS. This indicated the non-toxic potential of nanoparticles of WCS, which can be utilized in the biomedical field for various applications.205
9. Conclusion
This review presented an extensive literature survey on water chestnut starches and their wide application. Accordingly, it was found that starch is a good reservoir of high-molecular-weight carbohydrates, which completes the elementary dietary requirements of people. It has been utilized in the textile, paper, pharmaceutical, and food industries. However, the use of native starch is frequently restricted by its intrinsically flawed nature such as insolubility in water at room temperature, high retrogradation, and syneresis. These imperfections can be solved by various modifications such as physical, chemical, and enzymatic modifications. Thus, more studies should be conducted to evaluate the potential of starch as an important component in the food and pharmaceutical industry.
Conflicts of interest
There are no conflicts to declare.
References
- R. Rodrigues, P. C. Agarwal and N. K. Saha, Canning of water chestnut (Singhara)(Trapa bispinosa Roxb), J. Food Sci. Technol., 1964, 1, 28–31 CAS.
-
E. C. Little, Handbook of utilization of aquatic plants, A Review of World Literature, 1979 Search PubMed.
- J. Parekh and S. Chanda,
In vitro antimicrobial activity of Trapa natans L. fruit rind extracted in different solvents, Afr. J. Biotechnol., 2007, 6(6), 766–770 Search PubMed.
- A. K. Agrahari, M. Khaliquzzama and S. K. Panda, Evaluation of analgesic activity of methanolic extract of Trapa natans l. var. Bispinosa roxb. Roots, J. Curr. Pharm. Res., 2010, 1(1), 8–11 Search PubMed.
- S. Patel, D. Banji, O. J. Banji, M. M. Patel and K. K. Shah, Scrutinizing the role of aqueous extract of Trapa bispinosa as an immunomodulator in experimental animals, Int. J. Res. Pharm. Sci., 2010, 1(1), 13–19 Search PubMed.
- S. N. Das, B. Ray, S. K. Mahapatra and R. K. Pothal, Microbiological potentiality of Ipomoea sepiariaRoxb (Convolvulaceae), Int. J. Res. Pharm. Biomed. Sci., 2011, 2(2), 500–502 Search PubMed.
- A. Gani, S. S. Haq, F. A. Masoodi, A. A. Broadway and A. Gani, Physico-chemical, morphological and pasting properties of starches extracted from water chestnuts (Trapa natans) from three lakes of Kashmir, India, Braz. Arch. Biol. Technol., 2010, 53, 731–740 CrossRef CAS.
- F. N. Syed, M. H. Zakaria, J. S. Bujang and A. Christianus, Characterization, functional properties, and resistant starch of freshwater macrophytes, Int. J. Food Sci., 2021, 2021 Search PubMed.
- A. A. Wani, P. Singh, M. A. Shah, U. Schweiggert-Weisz, K. Gul and I. A. Wani, Rice starch diversity: Effects on structural, morphological, thermal, and physicochemical properties—A review, Compr. Rev. Food Sci. Food Saf., 2012, 11(5), 417–436 CrossRef CAS.
- G. D. Singh, A. S. Bawa, S. Singh and D. C. Saxena, Physicochemical, pasting, thermal and morphological characteristics of Indian water chestnut (Trapa natans) starch, Starch-Stärke, 2009, 61(1), 35–42 CrossRef CAS.
- C. Liu, S. Wang, X. Chang and S. Wang, Structural and functional properties of starches from Chinese chestnuts, Food Hydrocolloids, 2015, 43, 568–576 CrossRef CAS.
- T. M. Ali and A. Hasnain, Morphological, physicochemical, and pasting properties of modified white sorghum (Sorghum bicolor) starch, Int. J. Food Prop., 2014, 17(3), 523–535 CrossRef CAS.
- R. Hoover, Composition, molecular structure, and physicochemical properties of tuber and root starches: a review, Carbohydr. Polym., 2001, 45(3), 253–267 CrossRef CAS.
- S. N. Moorthy, Physicochemical and functional properties of tropical tuber starches: a review, Starch-Stärke, 2002, 54(12), 559–592 CrossRef CAS.
- M. M. Sánchez-Rivera, F. J. García-Suárez, M. V. Del Valle, F. Gutierrez-Meraz and L. A. Bello-Pérez, Partial characterization of banana starches oxidized by different levels of sodium hypochlorite, Carbohydr. Polym., 2005, 62(1), 50–56 CrossRef.
-
R. Fennema, Carbohydrates, in Food Chemistry, ed. J. N. BeMiller and L. Roy, Marcel Dekker, New York, NY, 1996, vol. 201 Search PubMed.
- L. Wang and Y. J. Wang, Structures and physicochemical properties of acid-thinned corn, potato and rice starches, Starch-stärke, 2001, 53(11), 570–576 CrossRef CAS.
- A. Surendra Babu, R. Parimalavalli, K. Jagannadham, J. Sudhakara Rao and R. Shalini Gaur, Fat mimicking properties of citric acid treated sweet potato starch, Int. J. Food Prop., 2016, 19(1), 139–153 CrossRef CAS.
- G. D. Singh, A. S. Bawa, C. S. Riar and D. C. Saxena, Influence of heat-moisture treatment and acid modifications on physicochemical, rheological, thermal and morphological characteristics of indian water chestnut (Trapa natans) starch and its application in biodegradable films, Starch-Stärke, 2009, 61(9), 503–513 CrossRef CAS.
- S. Hizukuri, Y. Takeda, T. Shitaozono, J. Abe, A. Ohtakara, C. Takeda and A. Suzuki, Structure and properties of water chestnut (Trapa natans L. var. bispinosa Makino) starch, Starch-Stärke, 1988, 40(5), 165–171 CrossRef CAS.
- L. Wang, B. Xie, G. Xiong, W. Wu, J. Wang, Y. Qiao and L. Liao, The effect of freeze–thaw cycles on microstructure and physicochemical properties of four starch gels, Food Hydrocolloids, 2013, 31(1), 61–67 CrossRef CAS.
- Z. Lutfi, A. Nawab, F. Alam and A. Hasnain, Morphological, physicochemical, and pasting properties of modified water chestnut (Trapabispinosa) starch, Int. J. Food Prop., 2017, 20(5), 1016–1028 CrossRef CAS.
- Z. Lutfi, A. Nawab, F. Alam, A. Hasnain and S. Z. Haider, Influence of xanthan, guar, CMC and gum acacia on functional properties of water chestnut (Trapa bispinosa) starch, Int. J. Biol. Macromol., 2017, 103, 220–225 CrossRef CAS PubMed.
- Z. Lutfi and A. Hasnain, Freeze-thaw stabilization of water chestnut (Trapa bispinosa) starch in the presence of gums and salts, Trakia J. Sci., 2013, 11(2), 163–169 Search PubMed.
- K. S. Hallale and V. V. Jadhao, Extraction of starch from water chestnut and its application Indahi as a stabilizer, J. Med. Chem. Drug Discov., 2016, 1, 265–287 Search PubMed.
- B. S. Yadav, P. Guleria and R. B. Yadav, Hydrothermal modification of Indian water chestnut starch: Influence of heat-moisture treatment and annealing on the physicochemical, gelatinization and pasting characteristics, LWT – Food Sci. Technol., 2013, 53(1), 211–217 CrossRef CAS.
- K. Gul, C. S. Riar, A. Bala and M. S. Sibian, Effect of ionic gums and dry heating on physicochemical, morphological, thermal and pasting properties of water chestnut starch, LWT – Food Sci. Technol., 2014, 59(1), 348–355 CrossRef CAS.
- M. Ahmad, A. Gani, I. Hassan, Q. Huang and H. Shabbir, Production and characterization of starch nanoparticles by mild alkali hydrolysis and ultra-sonication process, Sci. Rep., 2020, 10(1), 1 CrossRef PubMed.
- C. Dularia, A. Sinhmar, R. Thory, A. K. Pathera and V. Nain, Development of starch nanoparticles based composite films from non-conventional source-Water chestnut (Trapa bispinosa), Int. J. Biol. Macromol., 2019, 136, 1161–1168 CrossRef CAS PubMed.
- C. Cai and C. Wei, In situ observation of crystallinity disruption patterns during starch gelatinization, Carbohydr. Polym., 2013, 92(1), 469–478 CrossRef CAS PubMed.
- V. L. Murty, D. Choudhury and P. Bagchi, Physicochemical studies of water chestnut starch (Trapa bispinosa Roxb), Can. J. Chem., 1962, 40(12), 2260–2266 CrossRef CAS.
- Q. Liu, E. Weber, V. Currie and R. Yada, Physicochemical properties of starches during potato growth, Carbohydr. Polym., 2003, 51(2), 213–221 CrossRef CAS.
- W. S. Ratnayake and D. S. Jackson, Gelatinization and solubility of corn starch during heating in excess water: new insights, J. Agric. Food Chem., 2006, 54(10), 3712–3716 CrossRef CAS PubMed.
- J. Cai, C. Cai, J. Man, W. Zhou and C. Wei, Structural and functional properties of C-type starches, Carbohydr. Polym., 2014, 101, 289–300 CrossRef CAS PubMed.
- S. Y. Xu and C. F. Shoemaker, Gelatinization properties of Chinese water chestnut starch and lotus root starch, J. Food Sci., 1986, 51(2), 445–449 CrossRef CAS.
- T. Noda, S. Tsuda, M. Mori, S. Takigawa, C. Matsuura-Endo, K. Saito, W. H. Mangalika, A. Hanaoka, Y. Suzuki and H. Yamauchi, The effect of harvest dates on the starch properties of various potato cultivars, Food Chem., 2004, 86(1), 119–125 CrossRef CAS.
- W. R. Morrison, R. F. Tester and M. J. Gidley, Properties of damaged starch granules.
II. Crystallinity, molecular order and gelatinisation of ball-milled starches, J. Cereal Sci., 1994, 19(3), 209–217 CrossRef CAS.
- L. Wang, Z. Yin, J. Wu, Z. Sun and B. Xie, A study on freeze–thaw characteristics and microstructure of Chinese water chestnut starch gels, J. Food Eng., 2008, 88(2), 186–192 CrossRef CAS.
- J. Guo, L. Kong, B. Du and B. Xu, Morphological and physicochemical characterization of starches isolated from chestnuts cultivated in different regions of China, Int. J. Biol. Macromol., 2019, 130, 357–368 CrossRef CAS PubMed.
- C. Koteswara Reddy, L. Kimi and S. Haripriya, Variety difference in molecular structure, physico-chemical and thermal properties of starches from pigmented rice, Int. J. Food Eng., 2016, 12(6), 557–565 CAS.
- R. Hoover and F. Sosulski, Effect of cross-linking on functional properties of legume starches, Starch-Stärke, 1986, 38(5), 149–155 CrossRef CAS.
- L. A. Bello-Pérez, E. Agama-Acevedo, L. Sánchez-Hernández and O. Paredes-López, Isolation and partial characterization of banana starches, J. Agric. Food Chem., 1999, 47(3), 854–857 CrossRef PubMed.
- A. M. Hermansson and K. Svegmark, Developments in the understanding of starch functionality, Trends Food Sci. Technol., 1996, 7(11), 345–353 CrossRef CAS.
- J. Singh, A. Arora and S. Basu, Synthesis of coral like WO3/g-C3N4 nanocomposites for the removal of hazardous dyes under visible light, J. Alloys Compd., 2019, 808, 151734 CrossRef CAS.
- Z. Lutfi, F. Alam, A. Nawab, A. Haq and A. Hasnain, Effect of NaCl on physicochemical properties of xanthan gum–Water chestnut starch complexes, Int. J. Biol. Macromol., 2019, 131, 557–563 CrossRef CAS PubMed.
- B. Kaur, A. Fazilah and A. A. Karim, Alcoholic-alkaline treatment of sago starch and its effect on physicochemical properties, Food Bioprod. Process., 2011, 89(4), 463–471 CrossRef CAS.
- M. H. Lee, M. H. Baek, D. S. Cha, H. J. Park and S. T. Lim, Freeze–thaw stabilization of sweet potato starch gel by polysaccharide gums, Food Hydrocolloids, 2002, 16(4), 345–352 CrossRef CAS.
- R. Pongsawatmanit and S. Srijunthongsiri, Influence of xanthan gum on rheological properties and freeze–thaw stability of tapioca starch, J. Food Eng., 2008, 88(1), 137–143 CrossRef CAS.
- J. Muadklay and S. Charoenrein, Effects of hydrocolloids and freezing rates on freeze–thaw stability of tapioca starch gels, Food Hydrocolloids, 2008, 22(7), 1268–1272 CrossRef CAS.
- X. Zeng, B. Zheng, G. Xiao and L. Chen, Synergistic effect of extrusion and polyphenol molecular interaction on the short/long-term retrogradation properties of chestnut starch, Carbohydr. Polym., 2022, 276, 118731 CrossRef CAS PubMed.
- O. S. Lawal, Composition, physicochemical properties and retrogradation characteristics of native, oxidised, acetylated and acid-thinned new cocoyam (Xanthosoma sagittifolium) starch, Food Chem., 2004, 87(2), 205–218 CrossRef CAS.
- C. K. Reddy, F. Luan and B. Xu, Morphology, crystallinity, pasting, thermal and quality characteristics of starches from adzuki bean (Vigna angularis L.) and edible kudzu (Pueraria thomsonii Benth), Int. J. Biol. Macromol., 2017, 105, 354–362 CrossRef CAS PubMed.
- B. R. Cruz, A. S. Abraão, A. M. Lemos and F. M. Nunes, Chemical composition and functional properties of native chestnut starch (Castanea sativa Mill), Carbohydr. Polym., 2013, 94(1), 594–602 CrossRef CAS PubMed.
- R. P. Singh, J. K. Pandey, D. Rutot, P. Degée and P. Dubois, Biodegradation of poly (ε-caprolactone)/starch blends and composites in composting and culture environments: the effect of compatibilization on the inherent biodegradability of the host polymer, Carbohydr. Res., 2003, 338(17), 1759–1769 CrossRef CAS PubMed.
- M. J. Miles, V. J. Morris, P. D. Orford and S. G. Ring, The roles of amylose and amylopectin in the gelation and retrogradation of starch, Carbohydr. Res., 1985, 135(2), 271–281 CrossRef CAS.
- A. Abd
Karim, M. H. Norziah and C. C. Seow, Methods for the study of starch retrogradation, Food Chem., 2000, 71(1), 9–36 CrossRef.
- A. Kaur, N. Singh, R. Ezekiel and H. S. Guraya, Physicochemical, thermal and pasting properties of starches separated from different potato cultivars grown at different locations, Food Chem., 2007, 101(2), 643–651 CrossRef CAS.
- V. J. Morris, Starch gelation and retrogradation, Trends Food Sci. Technol., 1990, 1, 2–6 CrossRef CAS.
- K. Ghiasi, K. Varriano-Marston and R. C. Hoseney, Gelatinization of wheat starch. II. Starch-surfactant interaction, Cereal Chem., 1982, 59(2), 86–88 CAS.
- V. I. Lozinsky, L. G. Damshkaln, R. Brown and I. T. Norton, Study of cryostructuration of polymer systems. XVIII. Freeze–thaw influence on water-solubilized artificial mixtures of amylopectin and amylose, J. Appl. Polym. Sci., 2000, 78(2), 371–381 CrossRef CAS.
- A. C. Eliasson and H. R. Kim, Changes in rheological properties of hydroxypropyl potato starch pastes during freeze-thaw treatments I. A rheological approach for evaluation of freeze-thaw stability, J. Texture Stud., 1992, 23(3), 279–295 CrossRef.
- W. Lan, Y. Zhihua, Z. Yun, X. Bijun and S. Zhida, Morphological, physicochemical and textural properties of starch separated from Chinese water chestnut, Starch-Stärke, 2008, 60(3-4), 181–191 CrossRef.
- R. Hoover, Y. X. Li, G. Hynes and N. Senanayake, Physicochemical characterization of mung bean starch, Food Hydrocolloids, 1997, 11(4), 401–408 CrossRef CAS.
- R. B. Yadav, N. Kumar and B. S. Yadav, Characterization of banana, potato, and rice starch blends for their physicochemical and pasting properties, Cogent Food Agric., 2016, 2(1), 1127873 Search PubMed.
- S. Srichuwong and J. I. Jane, Physicochemical properties of starch affected by molecular composition and structures: a review, Food Sci. Biotechnol., 2007, 16(5), 663–674 CAS.
- T. Funami, M. Nakauma, S. Noda, S. Ishihara, I. Asai, N. Inouchi and K. Nishinari, Effects of some anionic polysaccharides on the gelatinization and retrogradation behaviors of wheat starch: Soybean-soluble polysaccharide and gum arabic, Food Hydrocolloids, 2008, 22(8), 1528–1540 CrossRef CAS.
- C. J. Keetels, T. Van Vliet and P. Walstra, Gelation and retrogradation of concentrated starch systems: 1 Gelation, Food Hydrocolloids, 1996, 10(3), 343–353 CrossRef CAS.
- C. Zhang, S. T. Lim and H. J. Chung, Physical modification of potato starch using mild heating and freezing with minor addition of gums, Food Hydrocolloids, 2019, 94, 294–303 CrossRef CAS.
- K. Zhao, B. Zhang, C. Su, B. Gong, J. Zheng, H. Jiang, G. Zhang and W. Li, Repeated heat-moisture treatment: a more effectiveway for structural and physicochemical modification of mung bean starch compared with continuous way, Food Bioprocess Technol., 2020, 13, 452–461 CrossRef CAS.
- E. da Rosa Zavareze and A. R. Dias, Impact of heat-moisture treatment and annealing in starches: A review, Carbohydr. Polym., 2011, 83(2), 317–328 CrossRef.
- Z. Guo, X. Li, D. Yang, A. Lei and F. Zhang, Structural and functional properties of chestnut starch based on high-pressure homogenization, LWT – Food Sci. Technol., 2022, 154, 112647 CrossRef CAS.
- W. Liu, W. Pan, J. Li, Y. Chen, Q. Yu, L. Rong, W. Xiao, H. Wen and J. Xie, Dry heat treatment induced the gelatinization, rheology and gel properties changes of chestnut starch, Curr. Res. Food Sci., 2022, 5, 28–33 CrossRef CAS PubMed.
- K. O. Adebowale, T. A. Afolabi and B. I. Olu-Owolabi, Hydrothermal treatments of Finger millet (Eleusine coracana) starch, Food Hydrocolloids, 2005, 19(6), 974–983 CrossRef CAS.
- R. Hormdok and A. Noomhorm, Hydrothermal treatments of rice starch for improvement of rice noodle quality, LWT – Food Sci. Technol., 2007, 40(10), 1723–1731 CrossRef CAS.
- Z. Maache-Rezzoug, I. Zarguili, C. Loisel, D. Queveau and A. Buleon, Structural modifications and thermal transitions of standard maize starch after DIC hydrothermal treatment, Carbohydr. Polym., 2008, 74(4), 802–812 CrossRef CAS.
- H. J. Chung, Q. Liu and R. Hoover, Impact of annealing and heat-moisture treatment on rapidly digestible, slowly digestible and resistant starch levels in native and gelatinized corn, pea and lentil starches, Carbohydr. Polym., 2009, 75(3), 436–447 CrossRef CAS.
- A. M. Gomes, C. E. da Silva and N. M. Ricardo, Effects of annealing on the physicochemical properties of fermented cassava starch (polvilho azedo), Carbohydr. Polym., 2005, 60(1), 1–6 CrossRef CAS.
- M. S. Varela, A. S. Navarro and D. K. Yamul, Effect of hydrocolloids on the properties of wheat/potato starch mixtures, Starch-Stärke, 2016, 68(7–8), 753–761 CrossRef CAS.
- J. N. BeMiller, Pasting, paste, and gel properties of starch–hydrocolloid combinations, Carbohydr. Polym., 2011, 86(2), 386–423 CrossRef CAS.
- K. Mahmood, H. Kamilah, P. L. Shang, S. Sulaiman and F. Ariffin, A review: Interaction of starch/non-starch hydrocolloid blending and the recent food applications, Food Biosci., 2017, 19, 110–120 CrossRef CAS.
- Y. Zhang, Z. Gu, L. Zhu and Y. Hong, Comparative study on the interaction between native corn starch and different hydrocolloids during gelatinization, Int. J. Biol. Macromol., 2018, 116, 136–143 CrossRef CAS PubMed.
- S. Jiang, L. Zou, Y. Hou, F. Qian, Y. Tuo, X. Wu, X. Zhu and G. Mu, The influence of the addition of transglutaminase at different phase on the film and film forming characteristics of whey protein concentrate-carboxymethyl chitosan composite films, Food Packag. Shelf Life, 2020, 25, 100546 CrossRef.
- S. T. Lim, J. A. Han, H. S. Lim and J. N. BeMiller, Modification of starch by dry heating with ionic gums, Cereal Chem., 2002, 79(5), 601–606 CrossRef CAS.
- Z. Lutfi, Q. Kalim, A. Shahid and A. Nawab, Water chestnut, rice, corn starches and sodium alginate. A comparative study on the physicochemical, thermal and morphological characteristics of starches after dry heating, Int. J. Biol. Macromol., 2021, 184, 476–482 CrossRef CAS PubMed.
- M. Tsakama, A. M. Mwangwela, T. A. Manani and N. M. Mahungu, Effect of heat moisture treatment on physicochemical and pasting properties of starch extracted from eleven sweet potato varieties, Int. Res. J. Agric. Sci. Soil. Sci, 2011, 1(7), 254–260 Search PubMed.
- A. Ali, T. A. Wani, I. A. Wani and F. A. Masoodi, Comparative study of the physico-chemical properties of rice and corn starches grown in Indian temperate climate, J. Saudi Soc. Agric. Sci., 2016, 15(1), 75–82 Search PubMed.
- P. L. Soni and H. W. Sharma, The Starch of Cassimiroa edulis-comparison with Maize Starch, Starch-Stärke, 1987, 39(2), 43–46 CrossRef CAS.
- T. A. Shittu, R. A. Aminu and E. O. Abulude, Functional effects of xanthan gum on composite cassava-wheat dough and bread, Food Hydrocolloids, 2009, 23(8), 2254–2260 CrossRef CAS.
- L. Day, C. Fayet and S. Homer, Effect of NaCl on the thermal behaviour of wheat starch in excess and limited water, Carbohydr. Polym., 2013, 94(1), 31–37 CrossRef CAS PubMed.
- N. Singh, M. Kaur, K. S. Sandhu and H. S. Guraya, Physicochemical, thermal, morphological and pasting properties of starches from some Indian black gram (Phaseolus mungo L.) cultivars, Starch-Stärke, 2004, 56(11), 535–544 CrossRef CAS.
- R. Hoove and T. Vasanthan, The effect of annealing on the physicochemical properties of wheat, oat, potato and lentil starches, J. Food Biochem., 1993, 17(5), 303–325 CrossRef.
- R. N. Waduge, R. Hoover, T. Vasanthan, J. Gao and J. Li, Effect of annealing on the structure and physicochemical properties of barley starches of varying amylose content, Food Res. Int., 2006, 39(1), 59–77 CrossRef CAS.
- R. C. Eerlingen, H. Jacobs, K. Block and J. A. Delcour, Effects of hydrothermal treatments on the rheological properties of potato starch, Carbohydr. Res., 1997, 297(4), 347–356 CrossRef CAS.
- A. Krüger, C. Ferrero and N. E. Zaritzky, Modelling corn starch swelling in batch systems: effect of sucrose and hydrocolloids, J. Food Eng., 2003, 58(2), 125–133 CrossRef.
- K. S. Pramodrao and C. S. Riar, Comparative study of effect of modification with ionic gums and dry heating on the physicochemical characteristic of potato, sweet potato and taro starches, Food Hydrocolloids, 2014, 35, 613–619 CrossRef CAS.
- M. Chaisawang and M. Suphantharika, Pasting and rheological properties of native and anionic tapioca starches as modified by guar gum and xanthan gum, Food Hydrocolloids, 2006, 20(5), 641–649 CrossRef CAS.
-
Gums and Stabilisers for the Food Industry 12, ed. P. A. Williams and G. O. Phillips, Royal Society of Chemistry, 2004 Search PubMed.
- B. J. Oosten, Interactions between starch and electrolytes, Starch-Stärke, 1990, 42(9), 327–330 CrossRef CAS.
- H. Zhou, C. Wang, L. Shi, T. Chang, H. Yang and M. Cui, Effects of salts on physicochemical, microstructural and thermal properties of potato starch, Food Chem., 2014, 156, 137–143 CrossRef CAS PubMed.
- S. S. Craig, C. C. Maningat, P. A. Seib and R. C. Hoseney, Starch paste clarity, Cereal Chem., 1989, 66(3), 173–182 CAS.
- O. S. Lawal, Studies on the hydrothermal modifications of new cocoyam (Xanthosoma sagittifolium) starch, Int. J. Biol. Macromol., 2005, 37(5), 268–277 CrossRef CAS PubMed.
- H. Jacobs, R. C. Eerlingen and J. A. Delcour, Factors affecting the visco-amylograph and rapid visco-analyzer evaluation of the impact of annealing on starch pasting properties, Starch-Stärke, 1996, 48(7-8), 266–270 CrossRef CAS.
- R. Stute, Hydrothermal modification of starches: The difference between annealing and heat/moisture-treatment, Starch-Stärke, 1992, 44(6), 205–214 CrossRef CAS.
- P. O. Serrano and C. M. L. Franco, Annealing and enzymatic hydrolysis of cassava starch, Braz. J. Food Technol., 2005, 8(3), 220–232 CAS.
- A. M. Gomes, C. E. da Silva, N. M. Ricardo, J. M. Sasaki and R. Germani, Impact of annealing on the physicochemical properties of unfermented cassava starch (“Polvilho Doce”), Starch-Stärke, 2004, 56(9), 419–423 CrossRef CAS.
- H. S. Lim, J. A. Han, J. N. BeMiller and S. T. Lim, Physical modification of waxy maize starch by dry heating with ionic gums, J. Appl. Glycosci., 2006, 53(4), 281–286 CrossRef CAS.
- S. Y. Sim, L. H. Cheng and A. A. Noor Aziah, Effects of selected food gums on wheat flour or dough properties, Asian J. Food Agro-Ind., 2009, 2(4), 937–947 Search PubMed.
- Q. Chen, H. Yu, L. Wang, Z. ul Abdin, Y. Chen, J. Wang, W. Zhou, X. Yang, R. U. Khan, H. Zhang and X. Chen, Recent progress in chemical modification of starch and its applications, RSC Adv., 2015, 5(83), 67459–67474 RSC.
- D. Vashisht, A. Pandey and K. J. Kumar, Physicochemical and release properties of carboxymethylated starches of Dioscorea from Jharkhand, Int. J. Biol. Macromol., 2015, 74, 523–529 CrossRef CAS PubMed.
- J. Liu, J. Ming, W. Li and G. Zhao, Synthesis, characterisation and in vitro digestibility of carboxymethyl potato starch rapidly prepared with microwave-assistance, Food Chem., 2012, 133(4), 1196–1205 CrossRef CAS.
- L. Xiao, J. Chen, X. Wang, R. Bai, D. Chen and J. Liu, Structural and physicochemical properties of chemically modified
Chinese water chestnut [Eleocharis dulcis (Burm. f.) Trin. ex Hensch] starches, Int. J. Biol. Macromol., 2018, 120, 547–556 CrossRef CAS PubMed.
- S. Wang, P. Sharp and L. Copeland, Structural and functional properties of starches from field peas, Food Chem., 2011, 126(4), 1546–1552 CrossRef CAS PubMed.
- A. Moin, T. M. Ali and A. Hasnain, Effect of succinylation on functional and morphological properties of starches from broken kernels of Pakistani Basmati and Irri rice cultivars, Food Chem., 2016, 191, 52–58 CrossRef CAS PubMed.
- C. U. Kemas, N. C. Ngwuluka, N. A. Ochekpe and E. I. Nep, Starch-based xerogels: Effect of acetylation on Physicochemical and rheological properties, Int. J. Biol. Macromol., 2017, 98, 94–102 CrossRef CAS PubMed.
- B. Zhang, X. Li, Q. Xie, H. Tao, W. Wang and H. Q. Chen, Preparation and characterization of non-crystalline granular starch and corresponding carboxymethyl starch, Int. J. Biol. Macromol., 2017, 103, 656–662 CrossRef CAS PubMed.
- L. A. Bello-Pérez, E. Agama-Acevedo, P. B. Zamudio-Flores, G. Mendez-Montealvo and S. L. Rodriguez-Ambriz, Effect of low and high acetylation degree in the morphological, physicochemical and structural characteristics of barley starch, LWT – Food Sci. Technol., 2010, 43(9), 1434–1440 CrossRef.
- L. Ansari, T. M. Ali and A. Hasnain, Effect of chemical modifications on morphological and functional characteristics of water-chestnut starches and their utilization as a fat-replacer in low-fat mayonnaise, Starch-Stärke, 2017, 69(1–2), 1600041 CrossRef.
- S. K. Sathe and D. K. Salunkhe, Isolation, partial characterization and modification of the great northern bean (Phaseolus vulgaris L.) starch, J. Food Sci., 1981, 46(2), 617–621 CrossRef CAS.
- C. Liu, H. Yan, S. Liu and X. Chang, Influence of Phosphorylation and Acetylation on Structural, Physicochemical and Functional Properties of Chestnut Starch, Polymers, 2022, 14(1), 172 CrossRef CAS PubMed.
- O. O. Olayinka, B. I. Olu-Owolabi and K. O. Adebowale, Effect of succinylation on the physicochemical, rheological, thermal and retrogradation properties of red and white sorghum starches, Food Hydrocolloids, 2011, 25(3), 515–520 CrossRef CAS.
- P. Van Hung and N. Morita, Physicochemical properties of hydroxypropylated and cross-linked starches from A-type and B-type wheat starch granules, Carbohydr. Polym., 2005, 59(2), 239–246 CrossRef CAS.
- S. Mehboob, T. M. Ali, F. Alam and A. Hasnain, Dual modification of native white sorghum (Sorghum bicolor) starch via acid hydrolysis and succinylation, LWT – Food Sci. Technol., 2015, 64(1), 459–467 CrossRef CAS.
- R. Colussi, V. Z. Pinto, S. L. El Halal, N. L. Vanier, F. A. Villanova, R. M. Silva, E. da Rosa Zavareze and A. R. Dias, Structural, morphological, and physicochemical properties of acetylated high-, medium-, and low-amylose rice starches, Carbohydr. Polym., 2014, 103, 405–413 CrossRef CAS PubMed.
- A. Gunaratne and H. Corke, Effect of hydroxypropylation and alkaline treatment in hydroxypropylation on some structural and physicochemical properties of heat-moisture treated wheat, potato and waxy maize starches, Carbohydr. Polym., 2007, 68(2), 305–313 CrossRef CAS.
- N. W. Cheetham and L. Tao, Variation in crystalline type with amylose content in maize starch granules: an X-ray powder diffraction study, Carbohydr. Polym., 1998, 36(4), 277–284 CrossRef CAS.
- S. Wang, J. Yu, Q. Zhu, J. Yu and F. Jin, Granular structure and allomorph position in C-type Chinese yam starch granule revealed by SEM, 13C CP/MAS NMR and XRD, Food Hydrocolloids, 2009, 23(2), 426–433 CrossRef CAS.
- X. Li, W. Y. Gao, L. J. Huang, Y. L. Wang, L. Q. Huang and C. X. Liu, Preparation and physicochemical properties of carboxymethyl Fritillaria ussuriensis Maxim. starches, Carbohydr. Polym., 2010, 80(3), 768–773 CrossRef CAS.
- V. E. Fernandez, G. G. Palazolo, N. A. Bosisio, L. M. Martínez and J. R. Wagner, Rheological properties and stability of low-in-fat dressings prepared with high-pressure homogenized yeast, J. Food Eng., 2012, 111(1), 57–65 CrossRef.
- R. Moreira, F. Chenlo, M. D. Torres and J. Glazer, Rheological properties of gelatinized chestnut starch dispersions: Effect of concentration and temperature, J. Food Eng., 2012, 112(1–2), 94–99 CrossRef CAS.
- S. Sun, G. Zhang and C. Ma, Preparation, physicochemical characterization and application of acetylated lotus rhizome starches, Carbohydr. Polym., 2016, 135, 10–17 CrossRef CAS PubMed.
- Z. Lutfi and A. Hasnain, Effect of different hydrocolloids on pasting behavior of native water chestnut (Trapa bispinosa) starch, Agric. Conspec. Sci., 2009, 74(2), 111–114 Search PubMed.
- M. A. El-Sheikh, New technique in starch nanoparticles synthesis, Carbohydr. Polym., 2017, 176, 214–219 CrossRef CAS PubMed.
- J. Castaño, R. Bouza, S. Rodríguez-Llamazares, C. Carrasco and R. V. Vinicius, Processing and characterization of starch-based materials from pehuen seeds (Araucaria araucana (Mol) K. Koch), Carbohydr. Polym., 2012, 88(1), 299–307 CrossRef.
- E. Ojogbo, J. Jardin and T. H. Mekonnen, Robust and sustainable starch ester nanocomposite films for packaging applications, Ind. Crops Prod., 2021, 160, 113153 CrossRef CAS.
- A. S. Abreu, M. Oliveira, A. de Sá, R. M. Rodrigues, M. A. Cerqueira, A. A. Vicente and A. V. Machado, Antimicrobial nanostructured starch based films for packaging, Carbohydr. Polym., 2015, 129, 127–134 CrossRef CAS PubMed.
- M. S. Khalil, Z. S. Ahmed and A. S. Elnawawy, Evaluation of the physicochemical properties and antimicrobial activities of bioactive biodegradable films, Jordan J. Biol. Sci., 2013, 147(623), 1 Search PubMed.
- A. M. Shi, L. J. Wang, D. Li and B. Adhikari, Characterization of starch films containing starch nanoparticles: Part 1: Physical and mechanical properties, Carbohydr. Polym., 2013, 96(2), 593–601 CrossRef CAS PubMed.
- D. Le Corre and H. Angellier-Coussy, Preparation and application of starch nanoparticles for nanocomposites: A review, React. Funct. Polym., 2014, 85, 97–120 CrossRef CAS.
- E. Y. Park, M. J. Kim, M. Cho, J. H. Lee and J. Y. Kim, Production of starch nanoparticles using normal maize starch via heat-moisture treatment under mildly acidic conditions and homogenization, Carbohydr. Polym., 2016, 151, 274–282 CrossRef CAS PubMed.
- J. H. Kim, D. H. Park and J. Y. Kim, Effect of heat-moisture treatment under mildly acidic condition on fragmentation of waxy maize starch granules into nanoparticles, Food Hydrocolloids, 2017, 63, 59–66 CrossRef CAS.
- M. P. Herrera and T. Vasanthan, Rheological characterization of gum and starch nanoparticle blends, Food Chem., 2018, 243, 43–49 CrossRef PubMed.
- M. Ahmad, P. Mudgil, A. Gani, F. Hamed, F. A. Masoodi and S. Maqsood, Nano-encapsulation of catechin in starch nanoparticles: Characterization, release behavior and bioactivity retention during simulated in-vitro digestion, Food Chem., 2019, 270, 95–104 CrossRef CAS PubMed.
- M. Ahmad, A. Gani, F. Hamed and S. Maqsood, Comparative study on utilization of micro and nano sized starch particles for encapsulation of camel milk derived probiotics (Pediococcus acidolactici), LWT – Food Sci. Technol., 2019, 110, 231–238 CrossRef CAS.
- Y. Chang, X. Yan, Q. Wang, L. Ren, J. Tong and J. Zhou, High efficiency and low cost preparation of size controlled starch nanoparticles through ultrasonic treatment and precipitation, Food Chem., 2017, 227, 369–375 CrossRef CAS PubMed.
- N. M. da Silva, P. R. Correia, J. I. Druzian, F. M. Fakhouri, R. L. Fialho and E. C. de Albuquerque, PBAT/TPS composite films reinforced with starch nanoparticles produced by ultrasound, Int. J. Polym. Sci., 2017, 2017 Search PubMed.
- M. Ahmad, A. Gani, F. A. Masoodi and S. H. Rizvi, Influence of ball milling on the production of starch nanoparticles and its effect on structural, thermal and functional properties, Int.
J. Biol. Macromol., 2020, 151, 85–91 CrossRef CAS PubMed.
- N. Li, M. Niu, B. Zhang, S. Zhao, S. Xiong and F. Xie, Effects of concurrent ball milling and octenyl succinylation on structure and physicochemical properties of starch, Carbohydr. Polym., 2017, 155, 109–116 CrossRef CAS PubMed.
- L. C. González, M. A. Loubes and M. P. Tolaba, Incidence of milling energy on dry-milling attributes of rice starch modified by planetary ball milling, Food Hydrocolloids, 2018, 82, 155–163 CrossRef.
- L. Dai, C. Li, J. Zhang and F. Cheng, Preparation and characterization of starch nanocrystals combining ball milling with acid hydrolysis, Carbohydr. Polym., 2018, 180, 122–127 CrossRef CAS PubMed.
- V. Nain, M. Kaur, K. S. Sandhu, R. Thory and A. Sinhmar, Development, characterization, and biocompatibility of zinc oxide coupled starch nanocomposites from different botanical sources, Int. J. Biol. Macromol., 2020, 162, 24–30 CrossRef CAS PubMed.
- J. E. Kinsella and N. Melachouris, Functional properties of proteins in foods: a survey, Crit. Rev. Food Sci. Nutr., 1976, 7(3), 219–280 CrossRef CAS.
- S. He, Y. Qin, E. Walid, L. Li, J. Cui and Y. Ma, Effect of ball-milling on the physicochemical properties of maize starch, Biotechnol. Rep., 2014, 3, 54–59 CrossRef PubMed.
- X. Zhu, Y. Cheng, P. Chen, P. Peng, S. Liu, D. Li and R. Ruan, Effect of alkaline and high-pressure homogenization on the extraction of phenolic acids from potato peels, Innovative Food Sci. Emerging Technol., 2016, 37, 91–97 CrossRef CAS.
- X. Tan, B. Zhang, L. Chen, X. Li, L. Li and F. Xie, Effect of planetary ball-milling on multi-scale structures and pasting properties of waxy and high-amylose corn starches, Innovative Food Sci. Emerging Technol., 2015, 30, 198–207 CrossRef CAS.
- R. Kumar and B. S. Khatkar, Thermal, pasting and morphological properties of starch granules of wheat (Triticum aestivum L.) varieties, J. Food Sci. Technol., 2017, 54, 2403–2410 CrossRef CAS PubMed.
- M. Ahmad, B. Ashraf, A. Gani and A. Gani, Microencapsulation of saffron anthocyanins using β glucan and β cyclodextrin: Microcapsule characterization, release behaviour & antioxidant potential during in vitro digestion, Int. J. Biol. Macromol., 2018, 109, 435–442 CrossRef CAS PubMed.
- M. Mondragón, L. A. Bello-Pérez, E. Agama-Acevedo, D. Betancur-Ancona and J. L. Peña, Effect of cooking time, steeping and lime concentration on starch gelatinization of corn during nixtamalization, Starch-Stärke, 2004, 56(6), 248–253 CrossRef.
- A. Hebeish, M. H. El-Rafie, M. A. El-Sheikh and M. E. El-Naggar, Ultra-fine characteristics of starch nanoparticles prepared using native starch with and without surfactant, J. Inorg. Organomet. Polym. Mater., 2014, 24, 515–524 CrossRef CAS.
- A. Chun, H. J. Lee, B. R. Hamaker and S. Janaswamy, Effects of ripening temperature on starch structure and gelatinization, pasting, and cooking properties in rice (Oryza sativa), J. Agric. Food Chem., 2015, 63(12), 3085–3093 CrossRef CAS PubMed.
- M. Ahmad, S. Qureshi, S. Maqsood, A. Gani and F. A. Masoodi, Micro-encapsulation of folic acid using horse chestnut starch and β-cyclodextrin: Microcapsule characterization, release behavior & antioxidant potential during GI tract conditions, Food Hydrocolloids, 2017, 66, 154–160 CrossRef CAS.
- X. Liu, Y. Chen, L. Wu, X. Wu, Y. Huang and B. Liu, Optimization of polysaccharides extraction from Dictyophora indusiata and determination of its antioxidant activity, Int. J. Biol. Macromol., 2017, 103, 175–181 CrossRef CAS PubMed.
- M. N. Marsh, Gluten, major histocompatibility complex, and the small intestine: a molecular and immunobiologic approach to the spectrum of gluten sensitivity (‘celiac sprue’), Gastroenterology, 1992, 102(1), 330–354 CrossRef CAS.
- N. A. Mir, K. Gul and C. S. Riar, Physicochemical,
pasting and thermal properties of water chestnut flours: A comparative analysis of two geographic sources, J. Food Process. Preserv., 2015, 39(6), 1407–1413 CrossRef CAS.
-
Frontiers in Celiac Disease, ed. A. Fasano, R. Troncone and D. Branski, Karger Medical and Scientific Publishers, 2008 Search PubMed.
-
E. K. Arendt and F. Dal Bello, Functional cereal products for those with gluten intolerance, in Technology of Functional Cereal Products, Woodhead Publishing, 2008, pp. 446–475 Search PubMed.
- N. A. Mir, K. Gul and C. S. Riar, Techno functional and nutritional characterization of gluten-free cakes prepared from water chestnut flours and hydrocolloids, J. Food Process. Preserv., 2015, 39(6), 978–984 CrossRef CAS.
- G. D. Singh, C. S. Riar, C. Saini, A. S. Bawa, D. S. Sogi and D. C. Saxena, Indian water chestnut flour-method optimization for preparation, its physicochemical, morphological, pasting properties and its potential in cookies preparation, LWT – Food Sci. Technol., 2011, 44(3), 665–672 CrossRef CAS.
- Z. Lutfi and A. Hasnain, Effect of Modified Water Chestnut (Trapa bispinosa) Starch on Physical and Sensory Properties of Sponge Cakes: Effect of Water Chestnut Starch on Cakes, Pak. J. Sci. Ind. Res. B: Biol. Sci., 2009, 52(3), 146–150 CAS.
- A. H. Malik, M. Faqir, S. Ayesh, I. Muhammad and S. Muhammad, Extraction of starch from Water Chestnut (Trapa bispinosa Roxb) and its application in yogurt as a stabilizer, Pak. J. Food Sci., 2012, 22(4), 209–218 Search PubMed.
- C. Han, Y. Zhao, S. W. Leonard and M. G. Traber, Edible coatings to improve storability and enhance nutritional value of fresh and frozen strawberries (Fragaria × ananassa) and raspberries (Rubus ideaus), Postharvest Biol. Technol., 2004, 33(1), 67–78 CrossRef CAS.
- M. A. Rojas-Graü, R. M. Raybaudi-Massilia, R. C. Soliva-Fortuny, R. J. Avena-Bustillos, T. H. McHugh and O. Martín-Belloso, Apple puree-alginate edible coating as carrier of antimicrobial agents to prolong shelf-life of fresh-cut apples, Postharvest Biol. Technol., 2007, 45(2), 254–264 CrossRef.
- C. Y. Basch, R. J. Jagus and S. K. Flores, Physical and antimicrobial properties of tapioca starch-HPMC edible films incorporated with nisin and/or potassium sorbate, Food Bioprocess Technol., 2013, 6, 2419–2428 CrossRef CAS.
- J. Mei, Y. Yuan, Q. Guo, Y. Wu, Y. Li and H. Yu, Characterization and antimicrobial properties of water chestnut starch-chitosan edible films, Int. J. Biol. Macromol., 2013, 61, 169–174 CrossRef CAS PubMed.
- K. Zehra, A. Nawab, F. Alam, A. Hadi and M. Raza, Development of novel biodegradable water chestnut starch/PVA composite film. Evaluation of plasticizer effect over physical, barrier, and mechanical properties, J. Food Process. Preserv., 2022, 46(3), e16334 CAS.
- W. F. Lai and W. T. Wong, Edible clusteroluminogenic films obtained from starch of different botanical origins for food packaging and quality management of frozen foods, Membranes, 2022, 12(4), 437 CrossRef CAS PubMed.
- K. O. Falade and C. A. Okafor, Physicochemical properties of five cocoyam (Colocasia esculenta and Xanthosoma sagittifolium) starches, Food Hydrocolloids, 2013, 30(1), 173–181 CrossRef CAS.
-
Materials Experience: Fundamentals of Materials and Design, ed. E. Karana, O. Pedgley and V. Rognoli, Butterworth-Heinemann, 2013 Search PubMed.
- K. M. Gupta, Starch based composites for packaging applications, Handb. Bioplast. Biocompos. Eng. Appl., 2011, 24, 189 Search PubMed.
- S. Pilla, Engineering applications of bioplastics and biocomposites-An overview, Handb. Bioplast. Biocompos. Eng. Appl., 2011, 1–5 Search PubMed.
-
European Bioplastics, Considerations of European Bioplastics Concerning, Bioplastics and the Circular Economy, 2015, available from: http://www.en.european-bioplastics.org/wpcontent/uploads/2015/publications/EUBP_Considerations_Circular_Economy_Proposal_2015.pdf Search PubMed.
- V. Kulkarni and S. Badwe, Comparative analysis by using greener route on the yield and colour of Bioplastic from edible precursors, Int. J. Creat. Res. Thoughts, 2020, 8(2), 2320–2882 Search PubMed.
- D. Rusu, S. Boyer, M. F. Lacrampe and P. Krawczak, Bioplastics for automotive applications, Handb. Bioplast. Biocompos. Eng. Appl., 2011, 81, 397 Search PubMed.
-
R. F. Shangraw, International Harmonization of Compendia Standards for Pharmaceutical Excipients, Topics in Pharmaceutical Sciences, 1992, pp. 205–223 Search PubMed.
- E. C. Ibezim, S. I. Ofoefule, E. O. Omeje, V. I. Onyishi and U. E. Odoh, The role of ginger starch as a binder in acetaminophen tablets, Sci. Res. Essays, 2008, 3(2), 046–050 Search PubMed.
- H. Musa, J. Muazu and P. G. Bhatia, Evaluation of fonio (Digitaria exilis) starch as a binder in paracetamol tablets, Niger. J. Pharm. Sci., 2008, 7(1), 56–66 Search PubMed.
- A. Okunlola and O. A. Odeku, Evaluation of starches obtained from four Dioscorea species as binding agent in chloroquine phosphate tablet formulations, Saudi Pharm. J., 2011, 19(2), 95–105 CrossRef CAS PubMed.
- M. U. Uhumwangho, R. S. Okor, F. E. Eichie and C. M. Abbah, Influence of some starch binders on the brittle fracture tendency of paracetamol tablets, Afr. J. Biotechnol., 2006, 5(20), 1950–1953 CAS.
- A. S. Bal and S. B. Joshi,
Trapa bispinosa starch as a tablet disintegrant, J. Pharm. Sci., 1974, 63(3), 473–474 CrossRef CAS PubMed.
- A. V. Singh, A. Singh, L. K. Nath and N. R. Pani, Evaluation of Trapa bispinosa Roxb. starch as pharmaceutical binder in solid dosage form, Asian Pac. J. Trop. Biomed., 2011, 1(1), S86–S89 CrossRef.
- S. Venkatesh, B. N. Babu, K. Latha, R. Alvala, M. B. Reddy and R. Mullangi, Evaluation of Trapa bispinosa Starch as an Alternative Tablet Excipient to Maize Starch: Assessment by Preformulation and Formulation Studies, Indian Drugs, 2012, 49(08), 27–32 CrossRef.
- K. N. Rao, P. Sudha, K. R. Vinod and D. Banji, Evaluation of Trapa natans starch as an excipient in tablet formulation, Res. J. Pharm., Biol. Chem. Sci., 2011, 2(1), 173–179 CAS.
- H. J. Bae, D. S. Cha, W. S. Whiteside and H. J. Park, Film and pharmaceutical hard capsule formation properties of mungbean, waterchestnut, and sweet potato starches, Food Chem., 2008, 106(1), 96–105 CrossRef CAS.
- C. Zhou, Y. Wang, H. Ma and R. He, Effect of ultrasonic degradation on in vitro antioxidant activity of polysaccharides from Porphyra yezoensis (Rhodophyta), Food Sci. Technol. Int., 2008, 14(6), 479–486 CrossRef CAS.
- Z. Hajdú, J. Hohmann, P. Forgo, T. Martinek, M. Dervarics, I. Zupkó, G. Falkay, D. Cossuta and I. Máthé, Diterpenoids and flavonoids from the fruits of Vitex agnus-castus and antioxidant activity of the fruit extracts and their constituents, Phytother Res., 2007, 21(4), 391–394 CrossRef PubMed.
- A. Kabouche, Z. Kabouche, M. Öztürk, U. Kolak and G. Topçu, Antioxidant abietane diterpenoids from Salvia barrelieri, Food Chem., 2007, 102(4), 1281–1287 CrossRef CAS.
- E. S. Lee, B. H. Lee, D. U. Shin, M. Y. Lim, W. H. Chung, C. S. Park, M. Y. Baik, Y. D. Nam and D. H. Seo, Amelioration of obesity in high-fat diet-fed mice by chestnut starch modified by amylosucrase from Deinococcus geothermalis, Food Hydrocolloids, 2018, 75, 22–32 CrossRef CAS.
- W. Liu, Y. Zhang, R. Wang, J. Li, W. Pan, X. Zhang, W. Xiao, H. Wen and J. Xie, Chestnut starch modification with dry heat treatment and addition of xanthan gum: Gelatinization, structural and functional properties, Food Hydrocolloids, 2022, 124, 107205 CrossRef CAS.
- G. Davidov-Pardo and D. J. McClements, Resveratrol encapsulation: Designing delivery systems to overcome solubility, stability and bioavailability issues, Trends Food Sci. Technol., 2014, 38(2), 88–103 CrossRef CAS.
- J. Chen, N. Wei, M. Lopez-Garcia, D. Ambrose, J. Lee, C. Annelin and T. Peterson, Development and evaluation of resveratrol, Vitamin E, and epigallocatechin gallate loaded lipid nanoparticles for skin care applications, Eur. J. Pharm. Biopharm., 2017, 117, 286–291 CrossRef CAS PubMed.
- X. Huang, Y. Liu, Y. Zou, X. Liang, Y. Peng, D. J. McClements and K. Hu, Encapsulation of resveratrol in zein/pectin core-shell nanoparticles: Stability, bioaccessibility, and antioxidant capacity after simulated gastrointestinal digestion, Food Hydrocolloids, 2019, 93, 261–269 CrossRef CAS.
- A. Podsedek, I. Majewska, M. Redzynia, D. Sosnowska and M. Koziołkiewicz,
In vitro inhibitory effect on digestive enzymes and antioxidant potential of commonly consumed fruits, J. Agric. Food Chem., 2014, 62(20), 4610–4617 CrossRef CAS PubMed.
- C. Carpéné, F. Les, G. Cásedas, C. Peiro, J. Fontaine, A. Chaplin, J. Mercader and V. López, Resveratrol anti-obesity effects: Rapid inhibition of adipocyte glucose utilization, Antioxidants, 2019, 8(3), 74 CrossRef PubMed.
- M. Ahmad and A. Gani, Ultrasonicated resveratrol loaded starch nanocapsules: Characterization, bioactivity and release behaviour under in vitro digestion, Carbohydr. Polym., 2021, 251, 117111 CrossRef CAS PubMed.
- S. Thungmungmee and N. Wisidsri,
In vitro and in vivo toxicity evaluation of Trapa bispinosa roxb. Starch, Int. J. Appl. Pharm., 2021, 13(1), 58–62, DOI:10.22159/ijap.2021.v13s1.Y0109.
- V. Nain, M. Kaur, K. S. Sandhu, R. Thory and A. Sinhmar, Development of Starch Nanoparticle from Mango Kernel in Comparison with Cereal, Tuber, and Legume Starch Nanoparticles: Characterization and Cytotoxicity, Starch-Stärke, 2022, 74(3–4), 2100252 CrossRef CAS.
|
This journal is © The Royal Society of Chemistry 2023 |
Click here to see how this site uses Cookies. View our privacy policy here.