Efficient mercury removal in 30 seconds by designing a dithiocarbamate-based organic polymer with customizable functionalities and tunable properties†
Received
19th September 2022
, Accepted 21st November 2022
First published on 22nd November 2022
Abstract
A novel class of organic polymer (OP) with customizable functional groups in the backbone and side-chain was designed and synthesized to remove toxic Hg2+ ions from contaminated water within 30 seconds using a simple spin column technique. The two-step synthetic strategy, which takes only one hour to synthesize a pure polymer from low-cost starting materials, effectuate in use for real-world applications. By changing the backbone and side-chain functional groups, their physical, chemical, thermal, morphological, and porous properties were tuned. The key functional group ‘dithiocarbamate’ was strategically incorporated in the polymeric backbone to remove toxic Hg2+. From the screening of synthesized materials, one candidate was effective in removing 99.9% Hg2+ ions from a wide range of contamination (5–400 ppm) via adsorption. By spin column technique, Hg2+ ions were removed in 30 s. The material was completely recyclable and thus reusable up to three cycles. It was also effective in purifying contaminated real water samples, including lake water and tap water. Taken together, this class of material has the potential to be exploited as an economically friendly candidate for various environmental related problems by tuning the structure as per the prerequisites for the application.
Water impact
There is a perpetual need of materials for the effective removal of highly toxic mercuric ions from contaminated water with a balance between performance, economy and labour costs. This work reports a class of material that can be synthesized quickly and can efficiently remove mercury using a novel adsorptive separation technique (spin column) within 30 seconds even from real water samples.
|
1. Introduction
Organic polymers (OP) are an imminent class of materials often expended primely to solve environmental and energy-related problems by removing heavy metal contaminants,1–4 organic dyes and pigments,5–7 volatile organic compounds,8,9 gaseous pollutants such as iodine,10–14 CO2
15–19 and by gas storage20–22 due to their high adsorption capacity. Hyper-crosslinked polymers, polymers of intrinsic microporosity, covalent organic framework, conjugated microporous polymer, porous aromatic framework etc. included in the family of OP.23 When compared with similar advanced materials such as the metal–organic framework or natural adsorbent materials, OP is superior in various properties, among which functionalization and tuneability are of paramount importance. The tunable functionalization of a material is an indispensable property when dealing with the use of materials for multiple applications. The scope of tunable functionalization of the material aids in modulating the physical and chemical properties of the material based on the requirement for application. Motivated by this, we have developed a novel class of OP that has access to tuning its physical, chemical, thermal and mechanical properties via customizing on-demand functional groups in both the backbone and side-chain. This class of material is stable and insoluble in water, and thereupon can be widely exploited for the decontamination of water.
The advanced technologies and industrial development for human perks have harmed the environment, causing air and water to be polluted with toxic heavy metals. Mercury has acute toxic effects that seriously harm the central nervous system, cardiovascular system24 and can cause congenital disabilities25 when exposed to its metallic and ionic forms. Mercury existence in air, water and soil is classified mainly in three forms: (i) metallic mercury or elemental mercury (Hg0), (ii) inorganic mercury (Hg+ and Hg2+), and (iii) organic mercury (methyl or ethyl mercury). The environmental condition is also a factor of mercuric forms. In oxidation conditions, HgCl42− and HgOH− prevail. Moreover, in reduction conditions, sulphur forms dominate (HgS2− and CH3HgS−) and alkyl forms exist in intermediate conditions. Unlike other toxic heavy metals, mercuric ions form methylmercury biochemically, which is highly toxic and easily absorbed by the body, even causing death.26 Hence, it is highly recommended to remove mercuric ions before conversion to methylmercury. Chemical precipitation,27 electrolysis,28 ion exchange method29etc. are a few techniques to remove mercury. Adsorption by physical and chemical methods is an easily accomplishable and cost-effective method for metal removal.30 MOFs are suitable for easy modification, greater recyclability and selectivity, and effective adsorption, but they have low or moderate stability. At the same time, COFs have limited modification ability even though they have good adsorption, improved stability, and sensitivity.31 In comparison, OP offers high removal capacity, tunable surface area and pore size, room for modifications for various structural changes, stability, and so on.32 The most important property of a material for use in a real-world application is balancing the cost and activity. A material with all of these properties is still a long-term goal in polymer and material chemistry, and our system is on its way to achieve this.
The soft-soft interaction between sulphur and mercury resulted in many thiol-functionalized materials for mercury removal. Porous materials with high surface area and high mercury uptake capacity were reported.33,34 These materials showed good removal properties, which was worth the comparatively tedious synthetic approach and the presence of a harmful thiol group. Herein, we put forth a de novo, fast, two-step, cost-effective and less laborious strategy to synthesize functionalized OP, which can effectively remove toxic mercury ions up to 99.9% in 30 seconds. Unlike other removal systems, dithiocarbamate esters have been functionalized in the polymer's backbone to interact with Hg2+ ions. A unique advantage of these systems is that a wide range of functional groups can be incorporated in both the backbone and side-chain to generate the material with on-demand property based on any required application. This material is an example of a functionally tunable organic polymer, which can be used as a highly efficient and rapid mercury removal system for fast, low-cost and large-scale production.
2. Results and discussion
The persistent demand for cost-effective and quickly synthesizable materials with tunable properties was considered for designing and synthesizing a novel class of functionalized OP. A two-step, catalyst-free synthetic strategy was implemented to synthesize OP using low-cost, readily available reagents with minimum labor cost and time. Owing to the importance of functionally tunable materials, the system has been strategically designed in such a way that any functional groups can be attached along with the freedom of varying the monomeric chain lengths. The reaction between a diamine of varying chain lengths (1, Scheme 1) and excess chloroacetyl chloride (2, Scheme 1) under room temperature was carried out as the first step. This reaction occurred in quantitative yield in N,N-dimethyl formamide (DMF) as the solvent, and the reaction was completed within 15 minutes (Fig. S1 and S2†). The second reaction is the polymerization step, a three-component reaction between the amide formed (3, Scheme 1) in the previous step, carbon disulfide (4, Scheme 1), and a separate diamine of the desired functional group and chain length (5, Scheme 1). The green solvent polyethylene glycol-200 (PEG-200) was used as the solvent for this reaction, resulting in the products in moderate to good yields (65–89%). The synthesis of these materials is quite simple, efficient, cost-effective and fast. As both the reactions were completed in 15 minutes, the total time required to obtain the polymer was only about 1 hour. At the same time, they are highly scalable and can be synthesized on the multi-gram scale.
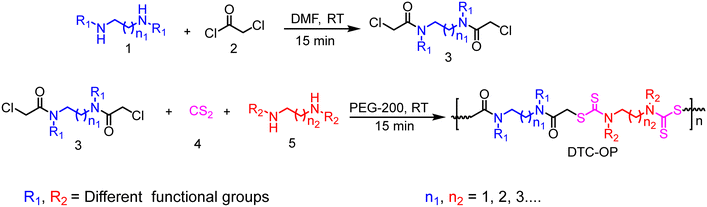 |
| Scheme 1 Two-step synthesis of DTC-OPs (DMF: N,N-dimethyl formamide, RT: room temperature, PEG: polyethylene glycol). | |
Interestingly, the polymerization worked well when the substituents on the N atom of the diamine is an aromatic group, which could be attributed to the ease of polymer formation due to the probable alignment of the monomeric units by pi-pi interaction. At the same time, the second reaction was more versatile such that any alkyl, aryl or hydrogen substituted diamines can be utilized based on the property required. Therefore, the pendant functional groups (R1, Scheme 1) to synthesize the chloroacetylated diamide were considered as benzyl and naphthyl. Meanwhile, hydrogen, methyl and benzyl were the functional groups in the diamine (R2, Scheme 1) used in the polymerization reaction. These amines can be of different lengths, so the polymer's chain length and other properties will differ. An important functional moiety, dithiocarbamate (DTC), was also strategically tailored to the OP for various applications in material and biomedical sciences.27,35–37 For the proof-of-concept, we have synthesized four polymers with varying side-chain functional groups and monomeric chain lengths (Fig. 1). DTC-OP1 and DTC-OP2 were obtained as powders, whereas DTC-OP3 and DTC-OP4 turned out to be a gummy elastic material, which became glassy in nature after drying them in a hot air oven at 100 °C. This glassy material was powdered using a mortar and pestle for further studies (Fig. S3†).
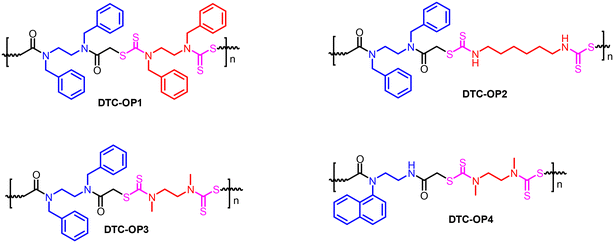 |
| Fig. 1 Structure of the synthesized materials DTC-Ops. | |
The material characterization was carried out by infrared (IR) spectroscopy, 1H nuclear magnetic resonance (NMR) spectroscopy and energy dispersive X-Ray analysis (EDAX). In the IR spectroscopy, the formation of the products was confirmed by the appearance of new peaks in the vicinity of 1050 and 1100 cm−1, which were absent in the starting material (Fig. 2a). These peaks are attributed to the symmetric and asymmetric stretching of thiocarbonyls. The product also showed characteristic peaks at 2923 cm−1 (stretching, C–H), 1640 cm−1 (stretching, amide C
O) and 1435 cm−1 (stretching, C–N) (Fig. 2a). The peak of C–Cl present at 550 cm−1 in the starting material was also absent in the products, confirming the formation of the material. 1H NMR also confirmed the formation of products by the appearance of signals corresponding to the protons present in the material (Fig. 2b). The EDAX data provided the ratio of each element present in the material, confirming the given DTC-OPs (Fig. S4–S7†). Elemental analysis was also carried out, and it was observed that the% composition matches with the calculated% with a few deviations (Table S1†).
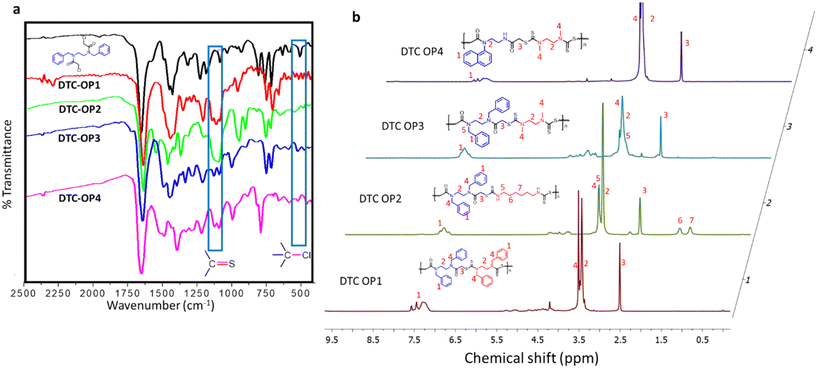 |
| Fig. 2 a) IR spectrum of the starting material and DTC-OP. b) Stacked 1H-NMR (400 MHz, DMSO-d6) of DTC-OP. | |
The porous nature of the materials was studied by nitrogen sorption analysis, and the Brunauer–Emmett–Teller (BET) surface area at 77 K was calculated from the isotherm obtained in physisorption analysis. Pore volume and average pore size distribution were also calculated from the analysis (Table 1). It was found that the order of the surface area of the materials is as follows: DTC-OP1 (91.49 m2 g−1) > DTC-OP4 (35.83 m2 g−1) > DTC-OP2 (27.20 m2 g−1) > DTC-OP3 (10.41 m2 g−1). The isotherm of all of the synthesized materials showed a hysteresis loop that indicated the hierarchical porosity. The nitrogen sorption isotherm of DTC-OP1, which showed the highest surface area and highest pore size distribution by non-local density functional theory (NLDFT), is given in Fig. 3 and in Fig. S8–S10† for other materials. It could be observed that these nanoporous materials contain maximum pores of the size 2 nm. At the same time, they also possess pores of the size ranging from 2 nm to 15 nm. This hierarchical porosity provides better reachability of the adsorbing material towards the chelating sites. The hierarchical porous nature of the materials was evident from the scanning electron microscopy (SEM) analysis. The SEM image of DTC-OP1 and DTC-OP2 differed from the SEM image of DTC-OP3 and DTC-OP4 (Fig. S11†). As mentioned above, the appearances of the former DTC-OPs were different. Thus, the morphological study by SEM appeared distinctive. The powder X-ray diffraction (XRD) studies of the former and latter sets of materials also showed variation. The powder samples of DTC-OP1 and DTC-OP2 appeared to be semicrystalline in nature, whereas the glassy materials of DTC-OP3 and DTC-OP4 appeared to be absolutely amorphous in nature (Fig. S12†). The above results indicate that different physical properties were obtained by modulating the pendant functional group and monomeric chain lengths of the materials.
Table 1 Porosity properties of DTC-OP (specific surface area was calculated from the nitrogen adsorption isotherm using the BET method in the relative pressure (P/P0) range from 0.1 to 0.3; the pore volume was calculated at P/P0 = 0.99)
Material |
BET surface area (m2 g−1) |
Pore volume (cc g−1) |
Pore size (nm) |
DTC-OP1 |
91.492 |
0.0719 |
1.7055 |
DTC-OP2 |
27.198 |
0.0414 |
3.114 |
DTC-OP3 |
10.406 |
0.0137 |
2.508 |
DTC-OP4 |
35.828 |
0.0375 |
2.173 |
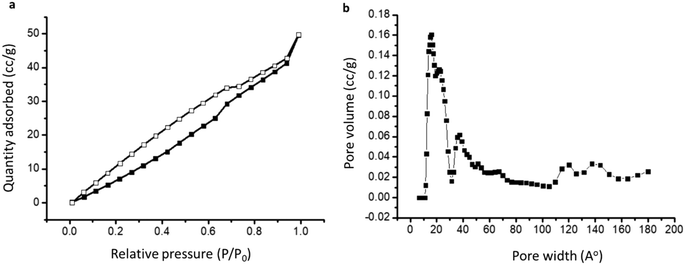 |
| Fig. 3 a) Nitrogen sorption isotherm of DTC-OP1 at 77 K. The solid shapes represent adsorption, and the hollow shapes represent desorption. b) NLDFT pore size distribution of DTC-OP1. | |
The thermal properties of the materials were studied by thermogravimetric analysis (TGA) and differential scanning calorimetry (DSC). From the TGA, it was observed that the materials were stable up to 214 °C to 246 °C based on their structure (Fig. 4). The glass transition temperature (Tg) of each DTC-OP was calculated from DSC analysis, and DTC-OP1 showed the highest Tg of 177.9 °C followed by DTC-OP2 (137.5 °C). DTC-OP3 and DTC-OP4 indicated their distinctiveness by the lower Tg values (Fig. S13†) of 121.9 °C and 123.2 °C, respectively, as expected. Unlike the other reported OP, the synthesized DTC-OP3 and DTC-OP4 exhibited an elastic property before drying. To explore the elastic property of these materials for various applications, the storage modulus and loss modulus of these materials were calculated. It was found that these exhibited suitable elastic properties (Fig. S14†).
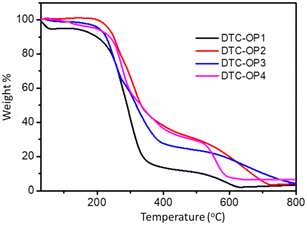 |
| Fig. 4 Thermogravimetric analysis plot of DTC-OP. | |
The mercury existence in air, water and soil is classified mainly in three forms: (i) metallic mercury or elemental mercury (Hg0), (ii) inorganic mercury (Hg+ and Hg2+), (iii) organic mercury (methyl or ethyl mercury). The environmental condition is also a factor of mercuric forms, such that in oxidation conditions, HgCl42− and HgOH− prevail. Meanwhile, in reduction conditions, the sulphur forms dominates (HgS2− and CH3HgS−) and the alkyl forms exist under intermediate conditions.38 The dithiocarbamate (DTC) units in the backbone and the porosity of the materials were utilized for the removal of Hg2+ ions from the contaminated water samples. The soft–soft interaction between the sulfur in the DTC with Hg2+ ions brings up the ions towards the material, and their porous nature aids in the accessibility of the ions towards the chelating sites. Preliminary screening of the adsorption study was carried out with all the synthesized materials with 5 mg of the adsorbent and 400 ppm Hg2+ solutions. It was observed that DTC-OP2 showed the highest uptake of Hg2+ from the solutions, followed by removing 99.9% of Hg2+ as measured by inductively coupled plasma-optical emission spectroscopy (ICP-OES) (Table 2). Even though DTC-OP1 showed the highest BET surface area, the highest removal was showcased by DTC-OP2, which may be ascribed to the largest pore size of DTC-OP2 compared to the other materials (Table 1). DTC-OP1 showed the lowest uptake of Hg2+ (41.8%) at this given concentration, which also showed the smallest pore size in the physisorption experiment. Thus, DTC-OP2 was set as the best material among the four for the effective removal of Hg2+-containing water samples, and further studies were carried out with this material.
Table 2 Hg2+ removal capacity of DTC-OPs (concentration of Hg2+ ions were calculated by ICP-OES)
Material |
Mass of the material taken (mg) |
Volume of the Hg2+ solution taken (ml) |
Concentration of the Hg2+ solution used (ppm) |
Concentration of the Hg2+ solution after adsorption (ppm) |
Uptake capacity (mg g−1) |
% removal |
DTC-OP1 |
5 |
4 |
400 |
232.8 |
133.76 |
41.80 |
DTC-OP2
|
5
|
4
|
400
|
0.286
|
319.77
|
99.92
|
DTC-OP3 |
5 |
4 |
400 |
182.92 |
173.66 |
54.27 |
DTC-OP4 |
5 |
4 |
400 |
122.44 |
222.05 |
69.39 |
The experimental set-up for the Hg2+ removal was prepared relatively simpler such that a mere stirring of the contaminated water with the material resulted in the removal of the toxic ions from the water. Adsorption analysis was carried out with the best material DTC-OP2 at various concentrations of Hg2+ ranging from 50 ppm to 800 ppm. The uptake capacity corresponding to each concentration was calculated. The uptake capacity increased linearly up to 500 ppm and was saturated at 800 ppm (Fig. 5a). Thus, the maximum uptake capacity was 413.7 mg g−1, outperforming many reported materials. The removal capacity was revealed to be 99.9%, and the instrument sensitivity did not permit to measure beyond that (Fig. 5b). The obtained data were fitted with the Langmuir adsorption isotherm by non-linear fitting with a correlation coefficient = 0.999 (Fig. 5a inset). Thus, the adsorption was completely mono layered in nature. The SEM image showed the Hg2+ ions adsorbed on the material as white particles (Fig. S15†), and the EDAX spectrum confirmed the presence of Hg2+ on the surface (Fig. S16†). The material was regenerated after an acidic wash with conc. HCl, followed by a water wash to make it neutral again, making it ready for further adsorption of Hg2+. The material DTC-OP2 showed 99.9% removal in the first and second cycles, and 94.1% in the third cycle (Fig. 5c). So, DTC-OP2 proved to be a potential recyclable candidate for removing toxic Hg2+ from the water samples.
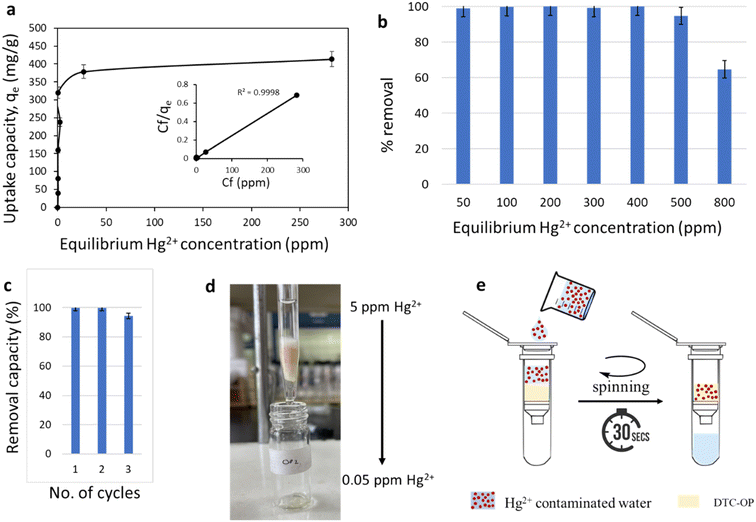 |
| Fig. 5 a) Mercuric ion adsorption isotherm for DTC-OP2; inset: nonlinear fitting with Langmuir adsorption. b) % removal of Hg2+ ions with DTC-OP2. c) Recyclability of DTC-OP2. d) Set up for column purification of Hg2+-contaminated water. On adsorption, 5 ppm Hg2+ concentration was reduced to 0.05 ppm, which is the detection limit of the instrument. e) Spin column set up for the purification of Hg2+-contaminated water. | |
The fast and highly efficient removal capacity and the reusability of the material directed us to design the column separation of the Hg2+ by using DTC-OP2 as the adsorbent. We tightly packed the material fitted in between cotton wool in a glass column, and added the mercury-containing water through it (Fig. 5d). The Hg2+-contaminated water that eluted through the material was collected and analyzed for Hg2+ ions by ICP-OES. It was observed that the eluted water was devoid of Hg2+ in a short time with a removal capacity of 99.7%. The reusability of the column after the wash increases the applicability of the system (Fig. 5d). This column set-up was advanced by the use of a spin column, where the contaminated Hg2+ was passed through the material loaded in a spin column fitted with a frit. The solution was eluted through this column in 30 seconds by spinning and showed 99.9% Hg2+ removal. Subsequently, improvement was made in terms of the time, labor and high-throughput. When compared to the stirring technique followed by filtration, the spin column is advantageous in terms of time by reducing an extra step of vacuum filtration. The regeneration of the material is also much easier, as the washing and drying can be done repeatedly within a short time. As they are available in different sizes, the selection can be made based on the requirement. To the best of our knowledge, this is the first report for the utilization of a spin column for metal removal studies. We are also the first to report the complete removal of toxic metal ions from water in 30 seconds, which includes the filtration of the material. The competitivity studies were also carried out with Hg2+ and other essential non-toxic elements. The material has shown to be effective for the removal of Hg2+ selectively without removing essential elements like K+, Na+ and Ca2+ (Table S3†). DTC-OP2 was found to be capable of removing 99.9% Hg2+ from the solutions of acidic and basic pH as well (pH = 4 and pH = 9) (Table S4†). For the removal of Hg2+, the studies were carried out in real samples (lake water and tap water), which contained all types of contaminants and competing ions and compounds. The material showed >98% removal (confirmed by ICP-OES) in real lake water samples and tap water (Table S5†). The scalability for real applications was also studied by carrying out the adsorption experiment in a higher volume (40 mL) with the lake water sample.
3. Conclusion
A novel synthetic platform for a dithiocarbamate-based organic polymer with customizable functional groups and tunable physical and chemical properties has been uncovered. This scheme is a robust example of a rapid, less laborious and economically viable synthesis for this kind of organic polymers. The finest advantage of this system is that any functional group, according to the on-demand property, can be attached to both the backbone and side-chain of the material, so that various functions can be achieved. These materials showed different levels of porosity, thermal, physical and mechanical properties upon changing their backbone and side-chain functional groups. The material DTC-OP showed exceptional mercury removal property (99.9%) from contaminated water within a very short time of 30 seconds over a wide range of contamination. The material showed good recyclability, and can also be used as a reusable material for the real-time column purification of Hg2+-contaminated water samples. The material removed the Hg2+ ion from different pH solutions, and showed good selectivity towards Hg2+ without removing the essential elements. It is also efficient in removing real water samples, including lake water and tap water. Currently, this DTC-OP platform is being explored for various other environmental and energy-related applications via tuning the functional groups at the backbone and side-chain of the material.
4. Experimental section
4.1. Materials and methods
All of the chemicals were purchased from Sigma Aldrich, Alfa Aesar, Spectrochem and TCI, and used without further purification. 1H NMR spectra were recorded on a Bruker AV III 500 MHz, and the data were analyzed by MestReNova (version 8.1.1). 1H NMR shifts are reported in units of ppm relative to tetramethyl silane. Fourier transform IR spectroscopy (FT-IR) was recorded in a Shimadzu IR Tracer 100 in KBr pellet method, and the spectra were plotted in OriginPro 8.5.1. SEM images were taken using Carl Zeiss – Gemini SEM 300 with 2
000
000× magnification. The quantitative analysis of mercury was done using an inductively coupled plasma-optical emission spectroscopy (ICP-OES) instrument (Perkin Elmer Optima 5300 DV). Thermal gravimetry analysis was carried out in a Perkin Elmer Inc Thermal Analyzer STA 8000. Differential scanning calorimetry was performed in a NETZSCH DSC 204F1 Phoenix. X-Ray diffraction studies were carried out in a Rigaku XRD Smart lab, 9 kW system. The rheometric analysis was carried out in Ares G2, TA instruments. Physisorption analysis was carried out in Quantachrome ASi Qwin, version 5.21.
4.2. Synthesis of chloroacetylated diamides
Chloroacetylated diamides (3) were synthesized from the reaction of diamines (1) and chloroacetyl chloride in the solvent DMF under room temperature. The reaction was monitored by TLC in a 7
:
3 system of ethyl acetate and hexane, and visualized under UV light. After completion of the reaction (15 min), the excess chloroacetyl chloride was quenched by the addition of sodium bicarbonate solution until the evolution of CO2 ceased. The reaction mixture was extracted by ethyl acetate and water, and the organic layer was passed through anhydrous Na2SO4. The solvent was removed under low pressure, and the product was obtained with high purity.
4.3. Synthesis of DTC-OP
The chloroacetylated diamides (3) were dissolved in the solvent PEG-200 and carbon disulfide, followed by the slow addition of diamines (5). The reaction is exothermic. After 15 minutes, the consumption of choroacetylated diamide was confirmed by TLC eluted in 70% ethyl acetate and water. The reaction was quenched with water, and ethyl acetate was added to this. A precipitate of DTC-OP was formed, and was filtered through Whatman filter paper. It was then washed many times with water and ethyl acetate until the organic layer was free from any compounds. The product was then dried in a hot air oven to give the DTC-OP.
4.4. Adsorption studies
A stock solution of 800 ppm Hg2+ was prepared by dissolving 27.1 mg of mercuric chloride (HgCl2) in 25 ml of water, and other concentrations were made by diluting this stock. The inductively coupled plasma-optical emission spectroscopy (ICP-OES) technique was used to calculate the Hg2+ ion concentration in all cases. For the adsorption isotherm, 5 mg of the material was mixed with 4 ml Hg2+ solutions of varied concentrations. The solution was sonicated for 1 minute for a uniform suspension of the material in the solution. This solution was stirred overnight for Hg2+ adsorption. Later, the solution was filtered through a 0.45 μm PVDF filer and the filtrate was given for ICP OES. The uptake capacity was calculated by using the given equation:
where Ci is the initial Hg2+ ion concentration and Cf is the final Hg2+ ion concentration in ppm, V is the volume of the solution used in liters, and m is the mass of the adsorbent in grams. The percentage removal was calculated by the equation:
4.5. Recyclability studies
About 20 mg of DTC-OP2 after Hg2+ adsorption was stirred with conc. HCl multiple times, stirred, and centrifuged. The solid was collected after centrifugation, and was washed with deionized water until the filtrate was neutral in pH, as indicated by pH paper. The product was dried and reused for the next round of Hg2+ removal.
4.6. Competitivity studies
A 50 ppm solution of Hg2+, Na+, K+ and Ca2+ was prepared and added to 5 mg of DTC-OP2. The solution was stirred overnight and filtered. The Hg2+ concentration in the filtrate was calculated by ICP-OES.
4.7. pH studies
Two 50 ppm solutions of Hg2+ (4 ml) at pH = 4 and pH = 9 were separately added to 5 mg of DTC-OP2, and stirred overnight and filtered. The Hg2+ concentration in the filtrate was calculated by ICP-OES.
4.8. Conventional column based Hg2+ removal
50 mg of DTC-OP2 was tightly packed in a glass column of 0.5 mm diameter in between the cotton wool plug. 400 ppm Hg2+ solution was added into the column, and the solution that passed through the material was collected in a vial. The flow rate was 33 μl min−1. The Hg2+ concentration in the collected solution was calculated by ICP-OES.
4.9. Spin column-based Hg2+ removal
50 mg of DTC-OP2 was tightly packed in the mini spin column, which is fitted by a silica-based frit. 750 μl 400 ppm solution was added into the column, and was spun for 30 seconds in 1000 RPM. The solution that passed through the material was collected in a vial, and the Hg2+ concentration in the collected solution was calculated by ICP-OES.
4.10. Hg2+ removal from real water samples
To the spin column loaded with 50 mg of DTC-OP2, lake water and tap water spiked with 50 ppm of Hg2+ was added and spun for 30 seconds. The solution that passed through the material was collected in a vial, and the Hg2+ concentration in the collected solution was calculated by ICP-OES. For the scalability study, 40 ml of lake water was spiked with 5 ppm mercuric chloride.
Conflicts of interest
The authors declare no conflict of interest.
Acknowledgements
We sincerely acknowledge the Central Instrumentation Facility (CIF) at Indian Institute of Technology Palakkad, SAIF at IIT Madras and IIT Bombay, India for technical support. We thank Indian Institute of Technology Palakkad, India; the Ramanujan Fellowship, Science and Engineering Research Board, Department of Science and Technology, India; the Core Research Grant, Science and Engineering Research Board, Department of Science and Technology, India and Scheme for Transformational and Advanced Research in Sciences, Ministry of Education and Technology, India for funding.
References
- L. Huang, R. Liu, J. Yang, Q. Shuai, B. Yuliarto, Y. V. Kaneti and Y. Yamauchi, Nanoarchitectured porous organic polymers and their environmental applications for removal of toxic metal ions, Chem. Eng. J., 2021, 408, 127991 CrossRef CAS.
- Y. He, Q. Liu, J. Hu, C. Zhao, C. Peng, Q. Yang, H. Wang and H. Liu, Efficient removal of Pb (II) by amine functionalized porous organic polymer through post-synthetic modification, Sep. Purif. Technol., 2017, 180, 142–148 CrossRef CAS.
- S. Ravi, P. Puthiaraj, K. H. Row, D.-W. Park and W.-S. Ahn, Aminoethanethiol-grafted porous organic polymer for Hg2+ removal in aqueous solution, Ind. Eng. Chem. Res., 2017, 56, 10174–10182 CrossRef CAS.
- R. Peng, G. Chen, F. Zhou, R. Man and J. Huang, Catalyst-free synthesis of triazine-based porous organic polymers for Hg2+ adsorptive removal from aqueous solution, Chem. Eng. J., 2019, 371, 260–266 CrossRef CAS.
- Y. Zhang, X. Hong, X.-M. Cao, X.-Q. Huang, B. Hu, S.-Y. Ding and H. Lin, Functional porous organic polymers with conjugated triaryl triazine as the core for superfast adsorption removal of organic dyes, ACS Appl. Mater. Interfaces, 2021, 13, 6359–6366 CrossRef CAS PubMed.
- X. Shen, S. Ma, H. Xia, Z. Shi, Y. Mu and X. Liu, Cationic porous organic polymers as an excellent platform for highly efficient removal of pollutants from water, J. Mater. Chem. A, 2018, 6, 20653–20658 RSC.
- L. Huang, M. He, B. Chen, Q. Cheng and B. Hu, Facile green synthesis of magnetic porous organic polymers for rapid removal and separation of methylene blue, ACS Sustainable Chem. Eng., 2017, 5, 4050–4055 CrossRef CAS.
- M. Dressler, Extraction of trace amounts of organic compounds from water with porous organic polymers, J. Chromatogr. A, 1979, 165, 167–206 CrossRef CAS.
- S. Lu, Q. Liu, R. Han, M. Guo, J. Shi, C. Song, N. Ji, X. Lu and D. Ma, Potential applications of porous organic polymers as adsorbent for the adsorption of volatile organic compounds, J. Environ. Sci., 2021, 105, 184–203 CrossRef CAS PubMed.
- X. Pan, C. Ding, Z. Zhang, H. Ke and G. Cheng, Functional porous organic polymer with high S and N for reversible iodine capture, Microporous Mesoporous Mater., 2020, 300, 110161 CrossRef CAS.
- Z. Li, H. Li, D. Wang, A. Suwansoontorn, G. Du, Z. Liu, M. M. Hasan and Y. Nagao, A simple and cost-effective synthesis of ionic porous organic polymers with excellent porosity for high iodine capture, Polymer, 2020, 204, 122796 CrossRef CAS.
- M. A. Sabri, M. H. Al-Sayah, S. Sen, T. H. Ibrahim and O. M. El-Kadri, Fluorescent aminal linked porous organic polymer for reversible iodine capture and sensing, Sci. Rep., 2020, 10, 1–11 CrossRef PubMed.
- X. Qian, B. Wang, Z.-Q. Zhu, H.-X. Sun, F. Ren, P. Mu, C. Ma, W.-D. Liang and A. Li, Novel N-rich porous organic polymers with extremely high uptake for capture and reversible storage of volatile iodine, J. Hazard. Mater., 2017, 338, 224–232 CrossRef CAS PubMed.
- Z. Guo, P. Sun, X. Zhang, J. Lin, T. Shi, S. Liu, A. Sun and Z. Li, Amorphous Porous Organic Polymers Based on Schiff-Base Chemistry for Highly Efficient Iodine Capture, Chem. – Asian J., 2018, 13, 2046–2053 CrossRef CAS.
- Z.-W. Liu and B.-H. Han, Ionic porous organic polymers for CO2 capture and conversion, Curr. Opin. Green Sustainable Chem., 2019, 16, 20–25 CrossRef.
- Y. Yang, C. Y. Chuah and T.-H. Bae, Polyamine-appended porous organic polymers for efficient post-combustion CO2 capture, Chem. Eng. J., 2019, 358, 1227–1234 CrossRef CAS.
- L. Zou, Y. Sun, S. Che, X. Yang, X. Wang, M. Bosch, Q. Wang, H. Li, M. Smith and S. Yuan, Porous organic polymers for post-combustion carbon capture, Adv. Mater., 2017, 29, 1700229 CrossRef PubMed.
- L. Xie and M. P. Suh, High CO2-Capture Ability of a Porous Organic Polymer Bifunctionalized with Carboxy and Triazole Groups, Chem. – Eur. J., 2013, 19, 11590–11597 CrossRef CAS PubMed.
- H. Gao, Q. Li and S. Ren, Progress on CO2 capture by porous organic polymers, Curr. Opin. Green Sustainable Chem., 2019, 16, 33–38 CrossRef.
- W.-C. Song, X.-K. Xu, Q. Chen, Z.-Z. Zhuang and X.-H. Bu, Nitrogen-rich diaminotriazine-based porous organic polymers for small gas storage and selective uptake, Polym. Chem., 2013, 4, 4690–4696 RSC.
- Z. Chang, D.-S. Zhang, Q. Chen and X.-H. Bu, Microporous organic polymers for gas storage and separation applications, Phys. Chem. Chem. Phys., 2013, 15, 5430–5442 RSC.
- K. Cousins and R. Zhang, Highly porous organic polymers for hydrogen fuel storage, Polymers, 2019, 11, 690 CrossRef CAS.
- S. Das, P. Heasman, T. Ben and S. Qiu, Porous Organic Materials: Strategic Design and Structure-Function Correlation, Chem. Rev., 2017, 117, 1515–1563 CrossRef CAS PubMed.
- B. Fernandes Azevedo, L. Barros Furieri, F. M. Peçanha, G. A. Wiggers, P. Frizera Vassallo, M. Ronacher Simões, J. Fiorim, P. Rossi de Batista, M. Fioresi and L. Rossoni, Toxic effects of mercury on the cardiovascular and central nervous systems, J. Biomed. Biotechnol., 2012, 2012, 949048 Search PubMed.
- M. Vigeh, E. Nishioka, K. Ohtani, Y. Omori, T. Matsukawa, S. Koda and K. Yokoyama, Prenatal mercury exposure and birth weight, Reprod. Toxicol., 2018, 76, 78–83 CrossRef CAS.
- Y. S. Hong, Y.-M. Kim and K.-E. Lee, Methylmercury exposure and health effects, J. Prev. Med. Public Health, 2012, 45, 353 CrossRef.
- A. Jose, P. Nanjan and M. Porel, Sequence-defined oligomer as a modular platform for selective sub-picomolar detection and removal of Hg 2+, Polym. Chem., 2021, 12, 5201–5208 RSC.
- R. Arora, N. Singh, K. Balasubramanian and P. Alegaonkar, Electroless nickel coated nano-clay for electrolytic removal of Hg (ii) ions, RSC Adv., 2014, 4, 50614–50623 RSC.
- S. Chiarle, M. Ratto and M. Rovatti, Mercury removal from water by ion exchange resins adsorption, Water Res., 2000, 34, 2971–2978 CrossRef CAS.
- M. Xia, Z. Chen, Y. Li, C. Li, N. M. Ahmad, W. A. Cheema and S. Zhu, Removal of Hg (II) in aqueous solutions through physical and chemical adsorption principles, RSC Adv., 2019, 9, 20941–20953 RSC.
- D. Dai, J. Yang, Y. Wang and Y. Yang, Recent progress in functional materials for selective detection and removal of mercury (II) ions, Adv. Funct. Mater., 2021, 31, 2006168 CrossRef CAS.
- S. Das, P. Heasman, T. Ben and S. Qiu, Porous organic materials: strategic design and structure–function correlation, Chem. Rev., 2017, 117, 1515–1563 CrossRef CAS PubMed.
- B. Li, Y. Zhang, D. Ma, Z. Shi and S. Ma, Mercury nano-trap for effective and efficient removal of mercury (II) from aqueous solution, Nat. Commun., 2014, 5, 1–7 Search PubMed.
- B. Aguila, Q. Sun, J. A. Perman, L. D. Earl, C. W. Abney, R. Elzein, R. Schlaf and S. Ma, Efficient mercury capture using functionalized porous organic polymer, Adv. Mater., 2017, 29, 1700665 CrossRef PubMed.
- D. S. Pal, D. K. Mondal and R. Datta, Identification of metal dithiocarbamates as a novel class of antileishmanial agents, Antimicrob. Agents Chemother., 2015, 59, 2144–2152 CrossRef CAS.
- R. D. Li, H. L. Wang, Y. B. Li, Z. Q. Wang, X. Wang, Y. T. Wang, Z. M. Ge and R. T. Li, Discovery and optimization of novel dual dithiocarbamates as potent anticancer agents, Eur. J. Med. Chem., 2015, 93, 381–391 CrossRef CAS.
- M. V. Stasevich, V. I. Zvarich, V. P. Novikov, S. D. Zagorodnyaya, O. Y. Povnitsa, M. A. Chaika, M. V. Nesterkina, I. A. Kravchenko, D. S. Druzhilovskii and V. V. Poroikov, 9, 10-Anthraquinone Dithiocarbamates as Potential Pharmaceutical Substances with Pleiotropic Actions: Computerized Prediction of Biological Activity and Experimental Validation, Pharm. Chem. J., 2020, 1–9 Search PubMed.
- B. Gworek, O. Bemowska-Kałabun, M. Kijeńska and J. Wrzosek-Jakubowska, Mercury in marine and oceanic waters—a review, Water, Air, Soil Pollut., 2016, 227(10), 1–19 CrossRef CAS PubMed.
|
This journal is © The Royal Society of Chemistry 2023 |
Click here to see how this site uses Cookies. View our privacy policy here.