Highly efficient photocatalytic degradation over rose-like 1D/2D La(OH)3/(BiO)2OHCl heterostructures boosted by rich oxygen vacancies and enhanced interfacial charge transfer†
Received
28th August 2022
, Accepted 3rd December 2022
First published on 8th December 2022
Abstract
In this work, novel rose-like photocatalysts were successfully fabricated by constructing 1D/2D La(OH)3 nanorod/(BiO)2OHCl nanosheet heterojunctions. The optimal sample, BOL-2, synthesized with a Bi/La molar ratio of 4
:
1 showed significantly boosted visible-light-induced photocatalytic activity. Its kinetic rate constant (k) in the photocatalytic degradation of tetracycline (TC) and o-nitrophenol was 3.5 and 6.4 times that of (BiO)2OHCl. The existence of rich surface oxygen vacancies (OVs) and 1D/2D heterojunctions together contributes to enhanced photocatalytic performance. The OVs broadened the light absorption range and enhanced visible light absorbance, while the formation of the La(OH)3/(BiO)2OHCl heterojunction by close 1D/2D interface contact improved the charge separation and transfer efficiency of BOL-2. The free radical scavenging tests and DMPO spin-trapping technology further affirmed that more ˙O2− and ˙OH as active species were generated in the presence of BOL-2 than in the presence of (BiO)2OHCl, thereby leading to remarkably improved photocatalytic performance. BOL-2 also exhibited excellent stability in photocatalytic degradation, and the degradation efficiency only declined by 2.5% in the fourth cycle. Possible photocatalytic mechanisms as well as degradation pathways of TC over BOL-2 were explored. This work will provide new insights into the rational design of efficient photocatalysts by 1D/2D heterojunction construction and OV engineering for wastewater remediation.
Environmental significance
Antibiotics are frequently detected in natural water resources due to the discharge of a large amount of wastewater containing antibiotics. The long-term presence of antibiotics in a natural environment largely accelerates the occurrence of antibiotic-resistant genes/bacteria and the spread of antibiotic drug resistance, which poses a serious threat to the security of ecological systems. Therefore, it is urgent to explore an efficient way to remove antibiotics from wastewater. This work has reported the fabrication of novel rose-like La(OH)3 nanorod/(BiO)2OHCl nanosheet photocatalysts by 1D/2D heterojunction construction and OV engineering for highly efficient removal of antibiotics from wastewater.
|
Introduction
Recently, frequent detection of various antibiotics in natural water resources, e.g., surface water and ground water, has attracted extensive public attention.1 Most of these antibiotics cannot be completely degraded by traditional treatment processes in wastewater treatment plants, leading to inevitable discharge of antibiotics into natural water bodies.2,3 The long-term presence of antibiotics in a natural environment largely accelerates the occurrence of antibiotic-resistant genes/bacteria and spread of antibiotic drug resistance, which becomes a serious threat to the security of ecological systems and even the health and safety of human beings.3,4 Therefore, it is urgent to explore an efficient way to remove antibiotics from wastewater.
Various technologies including membrane processes, microbial/enzymatic degradation, ozonation, and Fenton process have been studied to remove antibiotics.5 Among them, microbial/enzymatic degradation processes can decompose antibiotics into small harmless molecules, such as H2O and CO2, using microbial communities or enzymes. However, they show quite limited antibiotic removal efficiency at 9–20% in biological wastewater treatment.6 The membrane process shows high removal efficiency of antibiotics; however, it has disadvantages of high energy consumption and high operational cost.7 By contrast, semiconductor-based photocatalysis with the utilization of renewable solar energy is considered as one of the green and effective wastewater treatment strategies.1,4,8 Bismuth oxychloride (BiOCl) has been regarded as an attractive semiconductor with distinctive features of good photocatalytic activity, high chemical stability, low toxicity and corrosion resistance.8,9 The unique lamellar structure of BiOCl featured by [Bi2O2]2+ slabs sandwiched between two Cl− slabs enables the formation of internal static electric fields between the layers.8 When Cl− ions in BiOCl are partially replaced with OH− ions, a new material, (BiO)2(OH)Cl, is generated; it has stronger light absorption capability and a narrower band gap than bare BiOCl.10,11 These substituted OH− anions can not only capture photo-generated holes to form hydroxyl radicals preferentially but also enhance the internal static electric fields in (BiO)2OHCl, thus improving the photocatalytic activity.10 Nevertheless, the visible light absorption ability of (BiO)2OHCl still needs improvement.
Due to the unique electronic structure and low energy of surface Bi–O bonds, surface oxygen vacancy (OV) engineering has been regarded as an outstanding strategy to improve the light absorption capacities of Bi− based photocatalysts and promote their photocatalytic activity.12,13 The introduction of surface OVs can create a new discrete energy band between its conduction band (CB) and valence band (VB), which enables the OV-rich photocatalysts to harvest the light with a longer wavelength.14 Moreover, the OVs can not only act as adsorption centers for benefiting O2 adsorption onto the catalysts, but also act as electron (e−) traps for accelerating the production of superoxide radicals (˙O2−) by reacting adsorbed O2 with the trapped e−.13 The above-mentioned process leads to the extremely enhanced separation of photogenerated electrons and holes, and in turn, boosts the photocatalytic performance of OV-rich photocatalysts.
It is noted that morphology control has been widely selected to improve the photocatalytic performances of semiconductors.15–17 Among various architectures, the flower-like microstructure stands out for enhanced photocatalytic activity due to some extraordinary advantageous features including abundant porous and channel-rich structure, large specific surface area, and multiple reflection of light.16,17 These are beneficial features to promote the mass transfer of contaminants, provide more exposed active sites, and enhance the light absorption of flower-like photocatalysts.18,19 However, the construction of heterojunctions with two semiconductors having matched band energy structures can dramatically promote the spatial separation and transfer of photogenerated electron–hole pairs, thereby enhancing the photocatalytic activity.20–24 In particular, flower-like heterostructures assembled by binary or ternary semiconductor heterojunctions have gained great research interest due to the joint advantages from both morphology control and heterojunction construction.16,25 For example, Etogo et al. controllably synthesized BiOCl/(BiO)2CO3/Bi2O3 heterostructures with a flower-like morphology, which showed remarkably enhanced photocatalytic degradation efficiency towards organic pollutants, as compared with the mechanical mixture of (BiO)2CO3, Bi2O3, and BiOCl.25 However, until now, only a few types of (BiO)2OHCl-based heterojunction catalysts such as (BiO)2OHCl@Bi24O31Cl10 (ref. 26) and g-C3N4/(BiO)2(OH)xCl2−x (ref. 27) have been reported with enhanced photocatalytic performance.
As a typical rare earth hydroxide, 1D La(OH)3 has a unique electronic structure and a controllable morphology with a large specific surface area, which has been regarded as an emergent photocatalyst, and then drawn increasing research interest.11,23 The incorporation of La(OH)3 into heterojunctions has attracted significant attention because of its eminent properties such as reduction of band gap, generation of highly active hydroxyl radicals, and remarkable pollutant adsorption during the photocatalytic process.28 Various heterojunctions such as La(OH)3/BiOCl,11 La(OH)3/BiOI,23 La(OH)3@BaTiO3,24 and La(OH)3/sGO-Ag3VO4/Ag (ref. 28) have been fabricated with La(OH)3, and they showed boosted photocatalytic performances.
In this work, novel rose-like heterostructures were fabricated via constructing 1D/2D La(OH)3 nanorod/(BiO)2OHCl nanosheets by a facile and template-free hydrothermal method. The formed heterojunctions featuring with a multi-dimensional interface are expected to further improve the photocatalytic performance of the resulting composites by integrating fast electron transport along 1D nanorods and superior electron mobility in 2D nanosheets, both of which are beneficial for accelerating the transfer and separation of charge carries.29,30 Moreover, the combined 1D and 2D hierarchical structure would likely enlarge the interface contact area and then provide abundant active sites for superior photocatalytic performance.31,32 In our study, the visible-light-induced photocatalytic performances of 1D/2D La(OH)3/(BiO)2OHCl catalysts were optimized by selecting tetracycline hydrochloride (TC) and o-nitrophenol (o-NP) as target pollutants. The mechanisms underlying enhanced photocatalytic performance were explored in detail, which were supported by inherent characteristics including crystal, textual, optical, and photoelectrochemical properties of samples. It is believed that the improved photocatalytic performances were ascribed to the combined effect of flower-like heterojunctions and OVs. The possible photocatalytic degradation pathways of TC were also explored on the basis of identified intermediates.
Experimental
Preparation of photocatalysts
All the materials used are described in ESI† (ESI, Text S1). The synthesis of La(OH)3/(BiO)2OHCl heterojunction photocatalysts is schematically shown in Scheme 1. Typically, 0.97 g Bi(NO3)3·5H2O was dissolved in 20 mL deionized (DI) water, followed by the addition of 0.149 g KCl. An appropriate quantity of concentrated ammonia solution was used to adjust the pH of the solution to 10.0. Our previous results have indicated that BiOCl was formed when the pH of the solution was adjusted to 7.0.9 Herein, under alkaline conditions with a solution pH of 10.0, Cl− between the [Bi2O2]2+ layers of BiOCl could be partially replaced by OH− in the solution via an ion-exchange process, leading to the formation of (BiO)2OHCl.26 After continuous stirring for 5 min, a certain amount of La(NO3)3·6H2O (0.05 g, 0.1 g, 0.2 g or 0.3 g) was added, and the obtained mixture was stirred for another 1 h at room temperature before transferring to a polytetrafluoroethylene reactor for reaction at 180 °C for 20 h. Once the sealed reactor was cooled to room temperature, solid powder materials were obtained by centrifugal separation, followed by repeated washing with DI water and ethanol. The final product was dried at 60 °C for 12 h and named BOL-x (x = 1–4), corresponding to Bi/La molar ratios of 8
:
1, 4
:
1, 2
:
1 or 4
:
3, respectively.
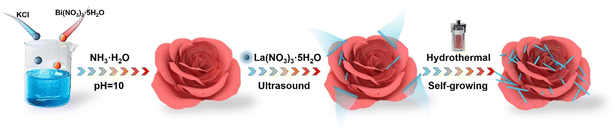 |
| Scheme 1 Synthesis of (BiO)2OHCl/La(OH)3 heterojunction photocatalysts. | |
For comparison, the samples of pure (BiO)2OHCl and La(OH)3 were also prepared. Pure (BiO)2OHCl was synthesized following the above-mentioned procedures but without the addition of La(NO3)3·6H2O. La(OH)3 nanorods were synthesized based on a previous study.23 Besides, mechanically mixed (BiO)2OHCl/La(OH)3 with a Bi/La molar ratio of 4
:
1 was synthesized for comparison. Briefly, 2 mmol (BiO)2OHCl and 1 mmol La(OH)3 were dispersed in 30 mL absolute ethyl alcohol and stirred for 12 h. The resulting sample was denoted as m-BOL-2.
Photocatalytic activity of catalysts
Tetracycline hydrochloride (TC) and o-nitrophenol (o-NP) were selected as typical pollutant models to study the photocatalytic activity of our catalyst materials. In brief, 50 mg photocatalyst was suspended in 80 mL aqueous solution containing TC (5–100 mg L−1) or o-NP (30 mg L−1) in a double-walled beaker reactor at room temperature. A 420 nm LED lamp was used to provide simulated visible light on top of the reactor. Before applying light irradiation, the suspension was stirred in the darkness for 30 min in order to reach the adsorption–desorption equilibrium. During light irradiation, 3 mL of the suspension was taken out and centrifuged at 10
000 rpm to analyze the residual concentration of TC or o-NP in the supernatant.
The trapping experiments were carried out to investigate the roles of reactive species, i.e. superoxide radical (˙O2−), holes (h+), and hydroxyl radicals (˙OH), in the photocatalytic degradation of TC over BOL-2. 1,4-Benzoquinone (PBQ), ethylenediamine tetraacetic acid disodium salt dihydrate (EDTA-2Na) or tert-butanol (TBA) was added as the scavenger for ˙O2−, h+, or ˙OH, respectively. The experimental procedures were similar to the above-mentioned tests on photocatalytic activity. The reusability of BOL-2 was carried out by collecting and using it for four cycles. After each cycle, the spent catalyst sample was collected by centrifugation at 10
000 rpm for 10 min and then washed with distilled water. After drying at 80 °C overnight, it was reused in the next cycle of photocatalytic degradation. Approximately 85.4% of used catalyst was collected after each such centrifugation-washing-drying.
Results and discussion
Phase structures
Fig. 1a shows the XRD spectra of the as-prepared samples. For pure (BiO)2OHCl, the XRD peaks located at 2θ of 11.61°, 26.01°, 33.24° and 47.72° are ascribed to the (001), (101), (110) and (200) crystal planes of (BiO)2OHCl (JCPDS # 41-0708).10 Furthermore, the peaks at 2θ of 15.66°, 27.97°, 39.47° and 48.64° for pure La(OH)3 correspond to the (100), (101), (201) and (211) crystal planes of La(OH)3 (JCPDS # 83-2034).33 Note that the intensities of La(OH)3 characteristic peaks in BOL-x composites increase along with increased addition of La3+ during synthesis, from sample BOL-1 to BOL-4. Obvious diffraction peaks referred to La(OH)3 can be observed in the XRD pattern of BOL-4, whereas the La(OH)3 peaks in the XRD patterns of BOL-1 and BOL-2 are relatively weak, probably due to the smaller amount of La(OH)3 formed. However, La(OH)3-related peaks can still be clearly observed when the XRD pattern of BOL-2 is separately compared with La(OH)3 and (BiO)2OHCl standard cards (Fig. S1a†). This indicates that La(OH)3/(BiO)2OHCl heterojunctions have been successfully synthesized. Besides, Fig. S1b† shows the magnified XRD pattern of the 2θ region from 25° to 28°. It was found that the peaks of the (101) crystal plane for (BiO)2OHCl in BOL-x shift to higher values, which is believed due to lattice distortion caused by the incorporation of La3+ into (BiO)2OHCl. A similar phenomenon was also found by La(OH)3/BiOCl heterojunctions with high OVs.11
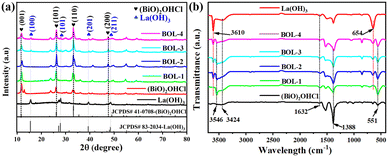 |
| Fig. 1 (a) XRD patterns and (b) FT-IR spectra of pure La(OH)3, (BiO)2OHCl, and BOL-x (x = 1, 2, 3, and 4). | |
The FT-IR spectra of samples are displayed in Fig. 1b. The absorption peaks at 3424 cm−1 and 1632 cm−1 are ascribed to the vibrations of the O–H bond from surface adsorbed water. For (BiO)2OHCl and BOL-x composites, the absorption peak at 3546 cm−1 is assigned to the stretching vibration of the O–H bond arising from (BiO)2OHCl, indicating the presence of hydroxyl groups in (BiO)2OHCl.34 The two absorption peaks at 551 cm−1 and 1388 cm−1 are identified as the vibrations of the Bi–O bond and Bi–Cl bond.10 For La(OH)3 and BOL-x composites, the absorption peak at 3610 cm−1 matches the vibration of the O–H bond in La(OH)3, and the band at 654 cm−1 corresponds to La–OH bending vibration in La(OH)3.35 Obviously, the FT-IR spectra of BOL-x composites include characteristic absorption peaks of Bi–O and La–OH originating from (BiO)2OHCl and La(OH)3, strongly confirming the successful synthesis of La(OH)3/(BiO)2OHCl composites.
Morphological characteristics
The morphology of (BiO)2OHCl, La(OH)3 and BOL-2 was investigated by SEM (Fig. 2). The sample (BiO)2OHCl exhibits rose-like microflowers assembled by interlaced 2D nanosheets (Fig. 2a and b), while La(OH)3 displays a stacked 1D rod-like structure (Fig. 2c). A similar rose-like morphology is also observed for BOL-2 (Fig. 2d). When these rose-like architectures are further magnified for investigation under SEM, several 1D La(OH)3 nanorods can be found to rationally grow on 2D (BiO)2OHCl nanosheets. Such close 1D/2D interface contact of La(OH)3/(BiO)2OHCl can also be obviously found in various parts of other BOL-2 microflowers (Fig. S2a and b†), indicating the formation of heterojunctions between La(OH)3 and (BiO)2OHCl with effective contact. Fig. S2g† shows the energy-dispersive X-ray spectra (EDS) of BOL-2, which reveals that all the O (20.37 wt%), Cl (5.80 wt%), La (19.84 wt%) and Bi (53.98 wt%) elements are present in BOL-2. This is further confirmed by the SEM mapping shown in Fig. S2c–f.†
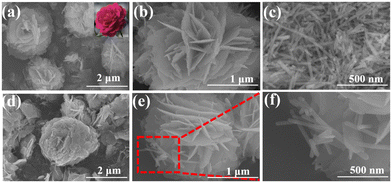 |
| Fig. 2 SEM images of (a and b) (BiO)2OHCl, (c) La(OH)3, and (d–f) BOL-2. | |
In Fig. 3a and b, the 1D nanorods are deposited on the surface of 2D nanosheets. The close contact between the 1D nanorods and 2D nanosheets leads to the formation of 1D/2D heterojunctions. Fig. 3c and d shows the HRTEM images of selected nanorod and nanosheet areas in Fig. 3b. As can be seen, the d space of 0.32 nm is attributed to the (101) plane of La(OH)3, while the d space of 0.34 nm is ascribed to the (101) plane of (BiO)2OHCl. Besides, the selected area electron diffraction (SAED) image of BOL-2 shows the (101), (110) and (200) crystal planes of (BiO)2OHCl and the (202) crystal plane of La(OH)3 (Fig. 3e). The TEM image in Fig. 3f and the corresponding elemental mapping images exhibit that Bi, Cl, O and La elements are well distributed on BOL-2. This observation further supports the successful construction of heterojunctions between 2D (BiO)2OHCl nanosheets and 1D La(OH)3 nanorods.
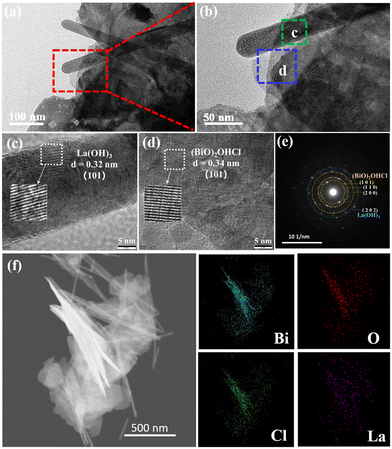 |
| Fig. 3 TEM images (a and b), HRTEM images (c and d), SAED image (e), and elemental mapping image (f) of BOL-2. | |
Textual properties
The N2 adsorption–desorption isotherms and corresponding pore size distribution plots of (BiO)2OHCl, La(OH)3 and BOL-x (x = 1–4) are shown in Fig. S3.†Table 1 lists the summary of specific surface area (SBET), average pore size and pore volume for each sample, which have been suggested as important factors affecting the photocatalytic property of the material.36 The SBET of the BOL-x composite gradually increases from BOL-1 (8.37 m2 g−1) to BOL-4 (14.79 m2 g−1), by increasing the molar ratio of La/Bi during synthesis. The slight increase in SBET values of composites should be the result of the formation of La(OH)3 nanorods on rose-like (BiO)2OHCl nanosheets; this is supported by the SEM observation in Fig. 2. It is also noted that the N2 adsorption–desorption isotherm curves for (BiO)2OHCl and BOL-x can be classified as type IV with H3-type hysteresis loops (Fig. S2a†), indicating the presence of slit-shaped pores aggregated from flake-like materials.27 The average pore sizes of the samples are in the range of 15–25 nm, as shown in the pore size distribution diagrams in Fig. S2c.† The presence of large mesopores may be due to the agglomeration of La(OH)3 nanorod/(BiO)2OHCl nanosheet heterojunctions in the rose-like hierarchical architecture. Such beneficial features are expected to provide channels for pollutants to reach more accessible active sites, thereby improving the photocatalytic activity of BOL-2.
Table 1 Specific surface area (SBET), average pore size and pore volumes of samples
Samples |
S
BET (m2 g−1) |
Average pore size (nm) |
Pore volume (cm3 g−1) |
(BiO)2OHCl |
9.42 |
28.33 |
0.067 |
La(OH)3 |
38.05 |
20.39 |
0.190 |
BOL-1 |
8.37 |
18.26 |
0.038 |
BOL-2 |
9.51 |
28.86 |
0.069 |
BOL-3 |
11.59 |
28.67 |
0.083 |
BOL-4 |
14.79 |
22.38 |
0.083 |
Surface composition
The XPS survey spectrum of BOL-2 confirms the presence of Bi, O, Cl, and La elements (Fig. S4†). In the high-resolution XPS spectrum of Bi 4f (Fig. 4a), the two peaks located at binding energies of 159.28 eV and 164.58 eV are ascribed to Bi 4f7/2 and Bi 4f5/2, suggesting that bismuth exists in the form of Bi3+ in BOL-2.10,37 Compared with pure (BiO)2OHCl shown in Fig. 4b, these two Bi 4f peaks are found to slightly shift to higher bonding energy regions, possibly resulting from the interaction between La(OH)3 and (BiO)2OHCl.27 Besides, two adjacent peaks with a lower intensity located at binding energies of 158.18 and 163.68 eV are referred to low-valence state Bi3–x due to the presence of OVs.26 In Fig. 4c, two sets of bimodal peaks ascribed to La 3d3/2 and La 3d5/2 of BOL-2 can be fitted into four peaks at binding energies of 851.83/855.44 eV and 834.80/838.79 eV, respectively, suggesting the presence of La3+ in the form of La(OH)3.11,38 Compared with La 3d of pure La(OH)3 shown in Fig. 4d, all these four peaks in BOL-2 are observed to shift to higher binding energies, indicating the formation of La(OH)3/(BiO)2OHCl heterojunctions in BOL-2.11 The O 1s spectra for BOL-2 can be fitted into three peaks at 530.06 eV, 531.16 eV and 532.76 eV (Fig. 4e), which are ascribed to lattice oxygen of Bi–O/La–O bonds, the presence of oxygen vacancies (OVs), and oxygen of OH groups,10,11 respectively. Compared with (BiO)2OHCl (Fig. 4f) and La(OH)3 (Fig. 4g), these three peaks also undergo a shift. It is notable that the intensity of peaks ascribed to OVs in BOL-2 is much higher than that in (BiO)2OHCl and La(OH)3, with a calculated content of 26.37%, as compared to 13.61% and 23.98% for (BiO)2OHCl and La(OH)3 respectively. This indicates the higher OV concentration in BOL-2 than in (BiO)2OHCl and La(OH)3. As for the Cl 2p spectrum of BOL-2 (Fig. S4†), the two fitted peaks centered at 198.09 eV and 199.64 eV could be attributed to the bonding energies of Cl 2p3/2 and Cl 2p1/2.11 Similarly, in contrast to (BiO)2OHCl, these two peaks have a slight shift. Therefore, compared with pure (BiO)2OHCl and La(OH)3, the shifts of Bi 4f, La 3d, Cl 2P, and O 1s in BOL-2 confirm the successful formation of La(OH)3/(BiO)2OHCl heterojunctions with good interaction in BOL-2, which agrees well with the results of SEM, TEM, and EDX.
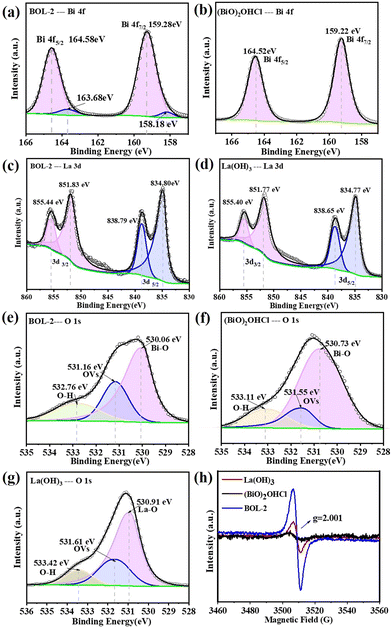 |
| Fig. 4 High-resolution XPS spectra of (a and b) Bi 4f for BOL-2 and (BiO)2OHCl, (c and d) La 3d for BOL-2 and La(OH)3, (e–g) O 1s for BOL-2, (BiO)2OHCl and La(OH)3; (h) EPR spectra of La(OH)3, (BiO)2OHCl and BOL-2. | |
To further confirm the existence of OVs, low-temperature electron paramagnetic resonance (EPR) was applied to provide persuasive evidence. The EPR spectra of BOL-2, (BiO)2OHCl and La(OH)3 are displayed in Fig. 4h. All the samples clearly show a symmetric resonance at about g = 2.001, which has been identified as paramagnetic species resulting from oxygen vacancy trapping and unpaired electrons, confirming the existence of OVs.8 Moreover, the peak intensity of BOL-2 is much stronger than that of La(OH)3, indicating that the amount of OVs in BOL-2 is much greater than that in La(OH)3. The EPR spectra of BOL-2 and m-BOL-2 are compared, as shown in Fig. S5a.† Notably, m-BOL-2, which was synthesized by mechanical mixing, exhibits significantly weaker peaks at around g = 2.001 than BOL-2, indicating a much lower concentration of OVs in m-BOL-2.
Optical properties
Fig. 5a shows the UV-vis diffuse reflectance spectra (UV-vis DRS) of the as-prepared samples, the technique of which has been commonly used to study the optical properties of catalyst materials.32,39 Clearly, the light absorption capacity of La(OH)3 within the UV region is much lower than that of (BiO)2OHCl and BOL-x, although its light absorption edge is recorded at ∼430 nm. As compared to (BiO)2OHCl, the BOL-x composites show enhanced UV adsorption and visible light response, with the red-shift of light absorption edge. Notably, the sample BOL-2 exhibits an obvious exponentially decaying absorption tail with improved absorption across the entire visible light region, which may be due to the presence of OVs in heterojunctions,11,39 later convinced by the XPS and EPR spectra shown in Fig. 4. This indicates the promising photocatalytic activity of BOL-2 induced by visible light. Besides, the UV-vis DRS spectra of m-BOL-2 and BOL-2 are compared in Fig. S5b.† Obviously, the sample of BOL-2 exhibits a wide light absorption range and enhanced light absorption capacity, indicating the importance of OVs in the optical property of La(OH)3/(BiO)2OHCl heterojunctions.
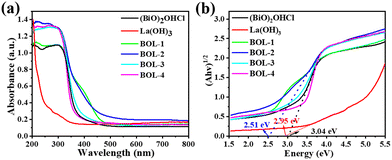 |
| Fig. 5 (a) UV-vis DRS spectra and (b) band gaps of (BiO)2OHCl, La(OH)3 and BOL-x (x = 1–4). | |
The band gap energy (Eg) can be estimated from the tangent intercept of (αhν)2vs. hν plots, according to the formula:10,34αhν = A(hν − Eg)n/2, in which α, h, ν, Eg and A are the absorption coefficient, Plank constant, optical frequency, band gap energy and constant value, respectively. The results are shown in Fig. 5b and Table S1.† The Eg value of pure (BiO)2OHCl was estimated to be 3.04 eV. It is noteworthy that the band gap of BOL-2 is approximately 2.51 eV, which is the narrowest among BOL-x (x = 1–4) samples; this material is expected to possess enhanced photocatalytic activity.
Photocatalytic activities
In order to examine the removal efficiency of catalysts, tetracycline hydrochloride (TC) and o-nitrophenol (o-NP) were selected as representative pollutants (Fig. 6). The adsorption of TC and o-NP was first studied in the darkness for 130 min (Fig. S6†). It can be seen that the removal rates of TC and o-NP over the as-prepared catalysts are almost unchanged from 30 min to 130 min, suggesting that adsorption equilibrium has been reached within 30 min. Fig. 6a presents the TC residual concentration (C) vs. its initial concentration (C0 = 30 mg L−1) along adsorption in the darkness and subsequently photocatalytic degradation under visible light irradiation. As can be seen, the change in TC concentration in the solution without any catalyst is almost negligible, indicating the good stability of TC in the darkness and under visible light. The samples of BOL-x (x = 1–4) show higher adsorption capacities in the darkness than both (BiO)2OHCl and La(OH)3, which are beneficial for highly efficient contaminant elimination.40,41 In Fig. 6a, the total removal efficiency of BOL-x (72.7–91.3%) is greater than that of pure (BiO)2OHCl (50.5%) and La(OH)3 (53.1%). In particular, BOL-2 shows the highest TC removal, reaching 91.3%. Moreover, higher degradation efficiencies, 96.7% and 98.3%, are achieved using BOL-2 in the TC solution with a lower initial concentration (10 mg L−1 and 5 mg L−1) (Fig. 7b and S7b†). Even at a relatively high initial concentration of 100 mg L−1, BOL-2 can still remove ∼58% of TC (Fig. S7a†). Such promising performances suggest the great potential application of BOL-2 in removing TC from raw domestic sewage (typically TC ranging from 100 ng L−1 to 5 mg L−1) and pharmaceutical/hospital wastewater (typically TC in the range of 100–500 mg L−1). It should be noted that the removal efficiency of m-BOL-2 (80.6%) is lower than that of BOL-2 (91.3%) under the same experimental conditions (Fig. S5d†), verifying the important role of OVs during the visible-light-induced photocatalytic process. Table S2† compares the removal efficiency of BOL-2 towards antibiotics with other bismuth-based catalysts11,24,26,34 reported in the literature. As can be seen, BOL-2 synthesized in this work exhibits a higher TC removal efficiency than most of our selected Bi-based photocatalysts under simulated visible light.
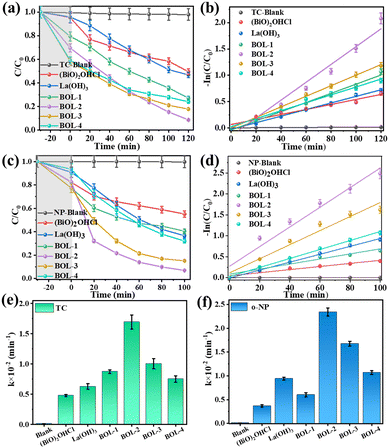 |
| Fig. 6 TC (a) and o-NP (c) removal by adsorption in the dark and photocatalytic degradation under visible light (C0 = 30 mg L−1); Pseudo-first-order kinetic plots of photocatalytic degradation of TC (b) and o-NP (d); comparison of kinetic rate constants k for TC (e) and o-NP (f) photocatalytic degradation. | |
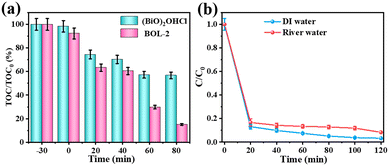 |
| Fig. 7 (a) Comparison of the percentage of TOC removal in the photocatalytic degradation of TC using BOL-2 and (BiO)2OHCl (C0 = 30 mg L−1). (b) Photocatalytic degradation of TC in DI water and river water using BOL-2 (C0 = 10 mg L−1). | |
Furthermore, almost no o-NP can be removed in the solution (C0 = 30 mg L−1) without catalyst. In the dark environment, the adsorptive removal of o-NP using BOL-x, (BiO)2OHCl, or La(OH)3 is less than 25%, due to the presence of limited functional groups on o-NP molecules and then their weak interaction with our prepared catalysts. However, it is clear that the photocatalytic degradation mainly accounts for the removal of o-NP. In particular, the photocatalytic degradation efficiency of BOL-2 is 93.1%, which is the highest among all the samples, further confirming the optimal photocatalytic activity of BOL-2.
The photocatalytic degradation kinetics of TC and o-NP was further analyzed using the pseudo-first-order reaction kinetic equation (eqn S3†).42 Their fitting curves are shown in Fig. 6b and d. Fig. 6e and f compare the kinetic rate constants (k) of samples, with the corresponding k values and correlation coefficients (R2) listed in Table S3.† Obviously, BOL-2 shows the highest k for TC (0.0169 min−1) and o-NP (0.0235 min−1) among all the samples. Moreover, the value of k for TC is 3.5 times and 2.7 times that of pure (BiO)2OHCl and La(OH)3, respectively, indicating the optimized photocatalytic activity of BOL-2 by rationally constructing (BiO)2OHCl/La(OH)3 heterojunctions.
The change of total organic carbon contents (TOC) in the solution during photocatalytic degradation of 30 mg L−1 TC is shown in Fig. 7(a). The value of TOC/TOC0 gradually decreases with the prolonged reaction time. After 80 min photocatalytic reaction, the percentage of TOC removal by utilizing BOL-2 reaches 84.9%, whereas that of pure (BiO)2OHCl is only 43.3%. Undoubtedly, BOL-2 has better capability to mineralize TC into CO2 and H2O during the photocatalytic degradation process.
The TC photocatalytic degradation efficiencies of BOL-2 in deionized (DI) water and river water were compared, as shown in Fig. 7(b). It is well known that natural water contains various inorganic ions and dissolved organics, which may suppress the photocatalytic activity of catalysts due to their competition for active sites and consuming part of reactive species. However, no obvious inhabitation was observed in the river water (Zhang jiang River, Jiangxi Province in China) sample containing 10 mg L−1 TC, indicating that a degradation efficiency of 91.6% was achieved after 120 min visible light irradiation, as compared to that of 96.7% in DI water. This proves that BOL-2 has a good photocatalytic activity in real water environment, which can be applied for practical wastewater remediation.
Fig. 8a shows the percentage of removal in the repetitive runs of 30 min dark adsorption and subsequently 120 min visible-light-initiated photocatalytic degradation of TC using BOL-2, respectively. As observed, a slight drop of 9.3% TC adsorptive removal is observed in the second run in the darkness, which is probably due to the occupation of active sites by intermediate products during TC photocatalytic degradation.43 However, the percentage of TC removal by photocatalytic degradation under visible light irradiation is almost unchanged in four cycles, indicating the good photocatalytic reusability of BOL-2. Fig. 8b compares the XRD patterns of fresh and spent BOL-2 before and after four cycles. There is no significant change observed in the XRD patterns, and the peaks assigned to (BiO)2OHCl and La(OH)3 can be clearly seen after four cycles, which indicates that BOL-2 has good stability.
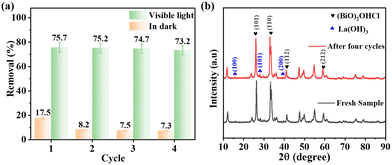 |
| Fig. 8 (a) Percentage of TC removal by BOL-2 in dark and under visible light irradiation in four consecutive cycles (C0 = 30 mg L−1). (b) XRD patterns of BOL-2 before and after four cycles. | |
In order to further study degradation and mineralization pathways of TC using BOL-2, the HPLC-MS analysis was used to study the intermediate products during photocatalytic degradation (Fig. 9 and S8†). There are 15 intermediates observed at main mass peaks of m/z = 403–74 (Fig. S5†), which are produced after the decomposition of specific functional groups, ring opening reaction, and hydroxylation of TC.44–46 According to their molecular weights and structures, it is inferred that two possible degradation pathways are involved (Fig. 9). In pathway I, the first intermediate product TC1 (m/z = 403) is produced by the deamidation of (–C
ONH2) of TC,46 while further demethylation at the –N(CH3)2 bond of the TC molecule and dehydration reaction lead to the formation of TC2 (m/z = 362).47 Next, the carbon ring open reaction along with deamination and dehydration reactions yields TC3 (m/z = 318), and then TC4 (m/z = 274) is formed by the decarboxylation reaction.47 Meanwhile, the intermediate TC5 (m/z = 362) is formed by losing a dimethylamino group from TC1 (m/z = 403). Further, TC6 (m/z = 318) and TC7 (m/z = 274) are formed due to the ring opening reaction at the TC5 molecule and followed by the elimination of the formyl group (–C
OCH3).46 In degradation pathway II, the intermediate TC8 (m/z = 395) is formed via demethylation at the –N(CH3)2 bond of the TC molecule, further dehydration process and loss of amino group; the following deamination and dehydroxylation processes lead to the formation of TC9 (m/z = 339), which is converted into TC7 (m/z = 282) by a ring-opening reaction.48,49 With the prolonged reaction time, the intermediates of TC4, TC7, and TC10 are further oxidized into TC11 (m/z = 230) by demethylation and dehydration processes, and the loss of functional groups (such as hydroxyl and methyl alcohol).50 When the reaction progresses further, some intermediates with relatively low molecular weights, i.e. TC12 (m/z = 182), TC13 (m/z = 149) and TC14 (m/z = 142), are generated by undergoing a ring-opening reaction,50,51 which are further oxidized into small molecules of TC15 (m/z = 74).44 Finally, some of the intermediate products are mineralized into CO2, H2O, NO3− and NH4+.
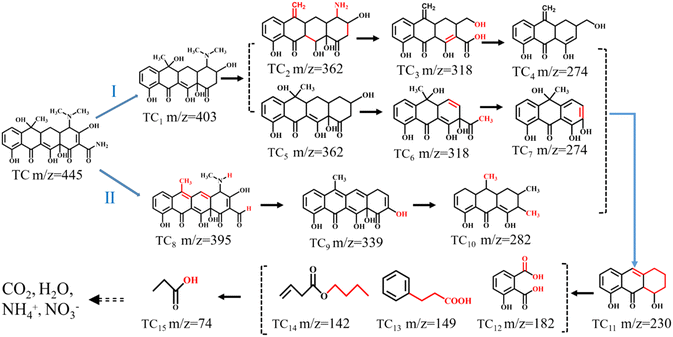 |
| Fig. 9 Intermediates and possible photocatalytic degradation pathways for TC removal using BOL-2. | |
As observed, our newly developed catalyst, BOL-2, has been proven to achieve high TC removal at different initial concentrations (e.g., 5, 10, 30 and 100 mg L−1) and, meanwhile, mineralize TC into CO2 and H2O during photocatalytic degradation. In particular, no apparently reduced performance was observed in the sample of river water, indicating BOL-2 to possess good photocatalytic activity in a real water environment. Moreover, BOL-2 exhibited excellent stability and good reusability in photocatalytic degradation. Due to the high TC removal rate, excellent stability, and good reusability of developed BOL-2, it shows promising potential for use in real wastewater treatment.
Electrochemical measurements
To reveal the internal origin of photocatalytic performance enhancement in the BOL-x samples, the separation, recombination, and transfer of charge carries were measured by several photoelectrochemical tests. Photocurrent transient response plots of BOL-x (x = 1–4) are shown in Fig. 10a, along with (BiO)2OHCl and La(OH)3 included for comparison. Generally, higher photocurrent indicates a higher separation efficiency of photogenerated electron/hole pairs.52 The photocurrent intensity decreases in the order of BOL-2 > BOL-3 > BOL-4 > La(OH)3 > BOL-1 > (BiO)2OHCl. Obviously, BOL-2 has the highest photocurrent intensity value, indicating the higher electron–hole (e−/h+) pair separation efficiency in BOL-2 than in other BOL-x samples, as well as (BiO)2OHCl and La(OH)3.53 This reveals that the rational construction of La(OH)3/(BiO)2OHCl heterojunctions by introducing proper amounts of La(OH)3 nanorods to (BiO)2OHCl nanosheets can greatly improve the separation efficiency of photo-generated e−/h+ pairs in the resulting catalyst.
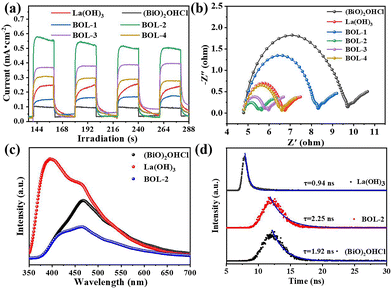 |
| Fig. 10 (a) Photocurrent transient response plots and (b) EIS Nyquist plots of (BiO)2OHCl, La(OH)3 and BOL-x (x = 1–4). (c) Photoluminescence (PL) spectra of (BiO)2OHCl, La(OH)3 and BOL-2. (d) Time-resolved fluorescence decay spectra of (BiO)2OHCl, La(OH)3 and BOL-2. | |
The electrochemical impedance spectroscopy (EIS) Nyquist plots of (BiO)2OHCl, La(OH)3 and BOL-x (x = 1–4) are displayed in Fig. 10b. In general, a smaller arc radius of Nyquist semicircle in the high-frequency region suggests lower interfacial charge transfer resistance and faster e−/h+ separation efficiency.40 As can be seen, the radius of La(OH)3/(BiO)2OHCl heterojunctions increases in the order of BOL-2 < BOL-3 < BOL-4 < BOL-1. It should be noted that the arc radius of BOL-2 is the smallest, indicating the lowest interfacial charge transfer resistance in BOL-2. This is consistent with the results from photocurrent transient response plots (Fig. 10a). Besides, the EIS plots of m-BOL-2 and BOL-2 are compared in Fig. S5c,† and the arc radius of m-BOL-2 is found much larger than that of BOL-2. The above-mentioned results further confirmed the most efficient charge separation and transfer ability in BOL-2, which contribute to its greatly enhanced photocatalytic activity.
Photoluminescence (PL) spectroscopy is used to detect the recombination status of photogenerated electron–hole pairs in catalysts. Fig. 10c shows the PL spectra of (BiO)2OHCl, La(OH)3 and BOL-2. La(OH)3 possesses a much higher PL intensity than those of pure (BiO)2OHCl and BOL-2 composites, indicating the highest recombination rate of photogenerated electron–hole pairs among three samples.36 Meanwhile, it is noted that the intensity of the PL emission peak for BOL-2 is much lower than that of La(OH)3 or (BiO)2OHCl, indicating the lowest charge recombination rate among the three samples.28
The time-resolved fluorescence emission decay spectra of (BiO)2OHCl, La(OH)3 and BOL-2 were used to study the lifetime of photo-generated charge carriers, as shown in Fig. 10d. The fitted parameters are summarized in Table S4.† As can be observed, the average lifetime (τ) of La(OH)3, (BiO)2OHCl and BOL-2 is 0.94 ns, 1.92 ns and 2.25 ns, respectively, which indicates that the life expectancy of photoinduced charge carriers in BOL-2 is 0.33 ns and 1.31 ns longer than that of (BiO)2OHCl and La(OH)3. Such extension of lifetime further discloses the enhanced interfacial charge separation efficiency by forming 1D/2D La(OH)3/(BiO)2OHCl heterojunctions.54,55
The charge separation of BOL-2 and (BiO)2OHCl was further analyzed by a surface photovoltage (SPV) technique, as shown in Fig. S9.† The SPV response of BOL-2 is much higher in peak intensity than that of (BiO)2OHCl, which further proves more efficient separation of the photo-generated e−/h+ pair in BOL-2 than in (BiO)2OHCl.56 Besides, BOL-2 shows a positive photoelectric response in the band range of 330–440 nm, while the photovoltage response range of (BiO)2OHCl is between 320 nm and 360 nm. The band range of BOL-2 which is broadened to the visible light region implies the greatly enhanced absorption and utilization of visible light by BOL-2, which is consistent with the results from DRS analysis.
Photocatalytic mechanisms
To elucidate the role of active species during the photocatalytic process, the trapping experiment of TC over BOL-2 and pristine (BiO)2OHCl was conducted (Fig. 11a and S10†), with the use of PBQ, EDTA-2Na, or TBA as the quencher for superoxide radicals (˙O2−), holes (h+), or hydroxide radicals (˙OH), respectively.57 As shown in Fig. S10,† when (BiO)2OHCl is used as a photocatalyst, the introduction of TBA or EDTA-2Na induces negligible impacts on its TC degradation efficiency. However, the addition of PBQ leads to a sharp reduction in the TC degradation with the use of (BiO)2OHCl, from 38.75% to only 3.09%. This indicates that ˙O2− plays a vital role in the (BiO)2OHCl photocatalytic system. In case of BOL-2 (Fig. 11a), the photocatalytic degradation of TC is most apparently weakened by 47.7% in the presence of PBQ, while a slight inhibition of ∼20% is observed in the TC degradation process when EDTA-2Na or TBA is used as the quencher. This suggests that ˙O2−, h+ and ˙OH all participate in the TC degradation in the BOL-2 photocatalytic system; in particular, ˙O2− is the main active species.
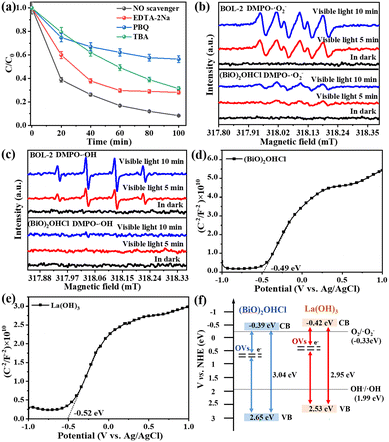 |
| Fig. 11 (a) Effect of different trapping agents on the photocatalytic degradation of TC on BOL-2; ESR spectra of BOL-2 (b) DMPO–˙O2− and (c) DMPO–˙OH. Mott-Schottky plots of (d) (BiO)2OHCl and (e) La(OH)3. (f) Energy band structures of (BiO)2OHCl and La(OH)3. | |
The ESR technology was then applied to further confirm the production of ˙O2− and ˙OH by BOL-2 and pure (BiO)2OHCl under visible light irradiation. As shown in Fig. 11b, no obvious DMPO–˙O2− characteristic signal is observed for either (BiO)2OHCl or BOL-2 in the darkness. However, typical DMPO–˙O2− characteristic signals are observed after light exposure (e.g. 5–10 min); the intensity of DMPO–˙O2− signals gradually increases with the prolonged exposure time. Moreover, the intensity of DMPO–˙O2− signals for BOL-2 is significantly higher than that of (BiO)2OHCl, suggesting that more ˙O2−- radicals can be generated by BOL-2 during the photocatalytic reaction.40 This could be ascribed to the more efficient interfacial charge separation efficiency and the presence of enriched surface OVs, which can act as traps to capture photo-generated electrons and promote the conversion of adsorbed oxygen into ˙O2− radicals.13 Similarly, no ESR signal of DMPO–˙OH adducts is found in the darkness (Fig. 11c), while an increase in the intensity of DMPO–˙OH characteristic peaks is detected over BOL-2 by extending its exposure time from 5 min to 10 min. It is also noted that BOL-2 possesses extremely stronger DMPO–˙OH signals than (BiO)2OHCl, suggesting the generation of more ˙OH by BOL-2 during the photocatalytic reaction.
The band positions of (BiO)2OHCl and La(OH)3 were studied using the Mott–Schottky plots (Fig. 11d and e), in which the linear part is a positive slope proving both of them as n-type semiconductors.58 As can be seen, the flat band potential (Efb) of (BiO)2OHCl and La(OH)3 is −0.49 eV and −0.52 eV vs. Ag/AgCl, respectively, which is equivalent to −0.29 eV and −0.32 eV vs. the normal hydrogen electrode (NHE).59 Generally, the conduction band potential (ECB) of an n-type semiconductor is about 0.1 eV more negative than its Efb.60 Therefore, the ECB value of (BiO)2OHCl and La(OH)3 was estimated to be −0.39 eV and −0.42 eV vs. NHE, respectively. Combined with the band gap value (Eg) derived from the DRS measurement in Fig. 5, the valence band potential (EVB) of (BiO)2OHCl and La(OH)3 was calculated as 2.65 eV and 2.53 eV vs. NHE, respectively, based on the empirical equation EVB = ECB + Eg.61Fig. 11f shows the detailed energy band structures of as-prepared (BiO)2OHCl and La(OH)3. Even though the wide band gap (3.04 eV) of (BiO)2OHCl seems inactive to visible light, the surface OVs enable the formation of an intermediate defect level between the CB and VB states, which can act as springboards to facilitate the excitation of photogenerated charges and enhance the light absorption property.8 The visible-light-induced photocatalytic activities of (BiO)2OHCl and La(OH)3 are due to the presence of OVs, which has been verified by their degradation capabilities towards TC and o-NP (Fig. 6). Moreover, due to the matched band energy structure (Fig. 11f), it is obvious that a type-II heterojunction can be formed by integrating (BiO)2OHCl with La(OH)3, which significantly enhances the charge transfer ability of BOL-2 and boosts the photocatalytic degradation performance, as evidenced in Fig. 6.
Therefore, based on the aforementioned observation, possible photocatalytic degradation mechanisms of TC and o-NP over BOL-2 are proposed, as shown in Scheme 2. Thanks to the rose-like architecture, 1D La(OH)3 nanorods are hierarchically distributed on 2D (BiO)2OHCl nanosheets to form a close contact interface, which significantly reduces the photo-induced carrier migration distance and, in turn, effectively inhibits the recombination of photo-generated e−–h+ pairs.62 Due to the presence of enriched surface OVs, the OV-induced intermediate level enables the production of photo-generated e−/h+ by both (BiO)2OHCl and La(OH)3, so that the excited e− transit to the OV level and then from the OV level to the CB level.13 The e− at the CB of La(OH)3 can migrate to the CB of (BiO)2OHCl, since ECB of La(OH)3 (−0.42 eV vs. NHE) is more negative than that of (BiO)2OHCl (−0.39 eV vs. NHE). Meanwhile, the h+ in the VB of (BiO)2OHCl can transfer to the VB of La(OH)3, because EVB of (BiO)2OHCl (2.65 eV vs. NHE) is greater than that of La(OH)3 (2.53 eV vs. NHE). As ECB of (BiO)2OHCl is more negative than E0 (O2/˙O2−) (−0.33
eV vs. NHE) and EVB of La(OH)3 is more positive than E0 (OH−/˙OH) (1.99 eV vs. NHE), the accumulated e− in the CB of (BiO)2OHCl possesses the ability of reducing O2 to ˙O2−, as well as h+ in the VB of La(OH)3 has the ability to oxidize OH− and then to yield ˙OH.63,64 Therefore, in this way, the e− and h+ can be efficiently separated, and the formed ˙O2−, ˙OH, along with h+ as active substances participate in the photocatalytic degradation process to achieve an excellent elimination effect of both TC and o-NP from wastewater. The organic pollutants are finally degraded into CO2, H2O, and other small compounds.
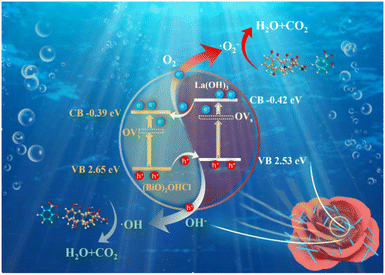 |
| Scheme 2 Photocatalytic degradation mechanisms of pollutants over BOL-2. | |
Conclusions
In this study, a series of rose-like photocatalysts with the assembly of 1D/2D La(OH)3 nanorod/(BiO)2OHCl nanosheet heterojunctions have been successfully synthesized. The novel La(OH)3/(BiO)2OHCl heterojunctions featured with rich OVs and close interface contact, which enhanced the visible light absorption capacity and improved the charge separation efficiency. The optimal sample BOL-2 showed superior removal efficiency for TC and o-NP by synergistic adsorption in the darkness and visible-light-induced photocatalytic degradation. The kinetic rate constant k of TC photocatalytic degradation over BOL-2 was 3.5 times and 2.7 times that of pure (BiO)2OHCl and La(OH)3, respectively. A TOC removal of 84.9% was achieved by BOL-2, as compared to only 43.3% over (BiO)2OHCl. It is believed that the unique rose-like heterostructure with large mesopores in BOL-2 was beneficial for improved adsorption of TC, while the formation of 1D/2D La(OH)3/(BiO)2OHCl heterojunctions in type-II scheme enhanced the interface charge separation efficiency, prolonged the lifetime of charge carries, and broadened the light absorption region. The free radical scavenging tests along with ESR analyses revealed the main role of ˙O2− along with the participation of h+ and ˙OH in the BOL-2 photocatalytic system. The catalyst BOL-2 showed excellent stability in four consecutive cycles. This work has provided a blueprint for the highly efficient removal of organic pollutants from wastewater by designing novel bismuth-based photocatalysts with the coupling effect of OV engineering and morphology control of heterojunctions.
Author contributions
Ying Tan & Qin Zhou: investigation, writing – original draft. Weiya Huang: conceptualization, supervision, writing – review and editing. Kangqiang Lu: writing – review and editing. Kai Yang: resources, writing – review and editing. Xunjun Chen: data curation, project administration. Hua He: formal analysis, resources. Dan Li: writing – review and editing. Dionysios D. Dionysiou: writing – review and editing.
Conflicts of interest
The authors declare that there are no competing financial interest.
Acknowledgements
The research was financially supported by National Natural Science Foundation of China (21962006), China Postdoctoral Science Foundation (2021M692456), Jiangxi Provincial Education Department Project (GJJ200819), Jiangxi Provincial Academic and Technical Leaders Training Program – Young Talents (20204BCJL23037), Jiangxi Province “Double Thousand Plan”, Jiangxi Provincial Natural Science Foundation (20212BAB213016) and Qingjiang Youth Talent Program (JXUSTQJYX20170005). D. D. Dionysiou also acknowledges support from the University of Cincinnati through the Herman Schneider Professorship in the College of Engineering and Applied Sciences.
References
- J. Wang and R. Zhuan, Degradation of antibiotics by advanced oxidation processes: An overview, Sci. Total Environ., 2020, 701, 135023 CrossRef CAS PubMed
.
- D. Cheng, H. Ngo, W. Guo, S. W. Chang, D. D. Nguyen, Y. Liu, Q. Wei and D. Wei, A critical review on antibiotics and hormones in swine wastewater: Water pollution problems and control approaches, J. Hazard. Mater., 2020, 387, 121682 CrossRef CAS
.
- J. Wang, L. Chu, L. Wojnarovits and E. Takacs, Occurrence and fate of antibiotics, antibiotic resistant genes (ARGs) and antibiotic resistant bacteria (ARB) in municipal wastewater treatment plant: An overview, Sci. Total Environ., 2020, 744, 140997 CrossRef CAS PubMed
.
- J. Chen, X. Chen, N. Li, Y. Liang, C. Yu and L. Yao, Enhanced photocatalytic activity of La1-xSrxCoO3/Ag3PO4 induced by the synergistic effect of doping and heterojunction, Ceram. Int., 2021, 47, 19923–19933 CrossRef CAS
.
- T. Zhu, Z. Su, W. Lai, Y. Zhang and Y. Liu, Insights into the fate and removal of antibiotics and antibiotic resistance genes using biological wastewater treatment technology, Sci. Total Environ., 2021, 776, 145906 CrossRef CAS
.
- A. S. Oberoi, Y. Jia, H. Zhang, S. K. Khanal and H. Lu, Insights into the fate and removal of antibiotics in engineered biological treatment systems: A critical review, Environ. Sci. Technol., 2019, 53, 7234–7264 CrossRef CAS PubMed
.
- M. Taheran, S. K. Brar, M. Verma, R. Y. Surampalli, T. C. Zhang and J. R. Valero, Membrane processes for removal of pharmaceutically active compounds (PhACs) from water and wastewaters, Sci. Total Environ., 2016, 547, 60–77 CrossRef CAS PubMed
.
- J. Guo, X. Li, J. Liang, X. Yuan, L. Jiang and H. Yu, Fabrication and regulation of vacancy-mediated bismuth oxyhalide towards photocatalytic application: Development status and tendency, Coord. Chem. Rev., 2021, 443, 214033 CrossRef CAS
.
- Q. Zhou, W. Huang, C. Xu, X. Liu, K. Yang, D. Li, Y. Hou and D. D. Dionysiou, Novel hierarchical carbon quantum dots-decorated BiOCl nanosheet/carbonized eggshell membrane composites for improved removal of organic contaminants from water via synergistic adsorption and photocatalysis, Chem. Eng. J., 2021, 420, 129582 CrossRef CAS
.
- Y. Zhai, A. Zhang, F. Teng, Y. Yang, W. Gu and W. Hao, Effect of mixed anion layer on energy band, charge separation and photochemical properties of (BiO)2OHCl, Appl. Catal., B, 2018, 224, 116–124 CrossRef CAS
.
- Z. Zhou, H. Xu, D. Li, Z. Zou and D. Xia, Microwave-assisted synthesis of La(OH)3/BiOCl n-n heterojunctions with high oxygen vacancies and its enhanced photocatalytic properties, Chem. Phys. Lett., 2019, 736, 136805 CrossRef CAS
.
- L. Hao, H. Huang, Y. Zhang and T. Ma, Oxygen vacant semiconductor photocatalysts, Adv. Funct. Mater., 2021, 31, 21001919 Search PubMed
.
- H. Li, J. Li, Z. Ai, F. Jia and L. Zhang, Oxygen vacancy-mediated photocatalysis of BiOCl: Reactivity, selectivity, and perspectives, Angew. Chem., Int. Ed., 2018, 57, 122–138 CrossRef CAS PubMed
.
- Z. Wan, M. Hu, B. Hu, T. Yan, K. Wang and X. Wang, Vacancy induced photocatalytic activity of La doped In(OH)3 for CO2 reduction with water vapor, Catal. Sci. Technol., 2020, 10, 2893–2904 RSC
.
- V. Subhiksha, S. Kokilavani and S. S. Khan, Recent advances in degradation of organic pollutant in aqueous solutions using bismuth based photocatalysts: A review, Chemosphere, 2022, 290, 133228 CrossRef CAS PubMed
.
- X. Liu, W. Y. Huang, Q. Zhou, X. R. Chen, K. Yang, D. Li and D. D. Dionysiou, Ag-decorated 3D flower-like Bi2MoO6/rGO with boosted photocatalytic
performance for removal of organic pollutants, Rare Met., 2021, 40, 1086–1098 CrossRef CAS
.
- P. Dong, G. Hou, X. Xi, R. Shao and F. Dong, WO3-based photocatalysts: morphology control, activity enhancement and multifunctional applications, Environ. Sci.: Nano, 2017, 4, 539–557 RSC
.
- Y. Peng, Q. Liu and S. Chen, Structural engineering of semiconductor nanoparticles by conjugated interfacial bonds, Chem. Rec., 2020, 20, 41–50 CrossRef CAS PubMed
.
- Y. Liu, L. Chen, Q. Yuan, J. He, C. Au and S. Yin, A green and efficient photocatalytic route for the highly-selective oxidation of saturated alpha-carbon C–H bonds in aromatic alkanes over flower-like Bi2WO6, Chem. Commun., 2016, 52, 1274–1277 RSC
.
- T. Ouyang, Y. Q. Ye, S. Guo, S. Huang, R. Zhao, S. Zhao and Z. Liu, 1D Bi/α-Fe2O3/ZnO nanojunction arrays as photocathode for efficient photoelectrochemical reduction CO2-to-HCOOH, J. Phys. Chem. Lett., 2022, 13, 6867–6874 CrossRef CAS PubMed
.
- S. Huang, Y. Wu, Q. Zhang, X. Jin, D. Li, H. Liu, P. Chen, W. Lv and G. Liu, Bi2O2CO3/Bi2O3 Z-scheme photocatalyst with oxygen vacancies and Bi for enhanced visible-light photocatalytic degradation of tetracycline, Environ. Sci.: Nano, 2022, 9, 2104–2120 RSC
.
- S. Guo, Z. Tang, Y. I. Du, T. Liu, T. Ouyang and Z. Liu, Chlorine anion stabilized Cu2O/ZnO photocathode for selective CO2 reduction to CH4, Appl. Catal., B, 2023, 321, 122035 CrossRef CAS
.
- Y. Sun, X. Xiao, X. Dong, F. Dong and W. Zhang, Heterostructured BiOI@La(OH)3 nanorods with enhanced visible light photocatalytic NO removal, Chin. J. Catal., 2017, 38, 217–226 CrossRef CAS
.
- B. Wang, K. Wei, F. Chen, Y. Wang, G. He and W. Li, Effects of active species on degrading A-ring of tetracycline in the Z-scheme heterostructured core-shell La(OH)3@BaTiO3 composition, J. Alloys Compd., 2019, 804, 100–110 CrossRef CAS
.
- A. Etogo, E. Hu, C. Zhou, Y. Zhong, Y. Hu and Z. Hong, Facile fabrication of mesoporous BiOCl/(BiO)2CO3/Bi2O3 ternary flower-like heterostructured microspheres with high visible-light-driven photoactivity, J. Mater. Chem. A, 2015, 3, 22413–22420 RSC
.
- C. Sun, R. Li, Y. Zhao, R. Yang, H. Xu, D. Ma and J. Zhao, Ammonia-dependent synthesis of (BiO)2OHCl@Bi24O31Cl10 heterostructures with enhanced visible-light induced photocatalytic activities on levofloxacin removal, J. Alloys Compd., 2022, 901, 163647 CrossRef CAS
.
- N. Han, Q. Xu, G. Beyene and Q. Zhang, Enhanced photocatalytic activity over g-C3N4/(BiO)2(OH)xCl2−x Z-scheme heterojunction, Appl. Surf. Sci., 2020, 521, 146464 CrossRef CAS
.
- R. N. Priyanka, S. Joseph, T. Abraham, N. J. Plathanam and B. Mathew, Novel La(OH)3-integrated sGO-Ag3VO4/Ag nanocomposite as a heterogeneous photocatalyst for fast degradation of agricultural and industrial pollutants, Catal. Sci. Technol., 2020, 10, 2916–2930 RSC
.
- H. Hou and X. Zhang, Rational design of 1D/2D heterostructured photocatalyst for energy and environmental applications, Chem. Eng. J., 2020, 395, 125030 CrossRef CAS
.
- R. Xiao, C. Zhao, Z. Zou, Z. Chen, L. Tian and H. Xu, In situ fabrication of 1D CdS nanorod/2D Ti3C2 MXene nanosheet Schottky heterojunction toward enhanced photocatalytic hydrogen evolution, Appl. Catal., B, 2020, 268, 118382 CrossRef CAS
.
- G. Yang, T. Chen, C. Xing, Z. Tian, Y. Hu and G. Yu, Construction of multi-scale 1D/2D CdS/ZnS(en)0.5 nanorod/nanosheet heterojunction to boost photocatalytic
hydrogen generation performance, Appl. Surf. Sci., 2022, 578, 152033 CrossRef CAS
.
- Y. Zhang, D. Chen, N. Li, Q. Xu, H. Li and J. Lu, Fabricating 1D/2D Co3O4/ZnIn2S4 core–shell heterostructures with boosted charge transfer for photocatalytic hydrogen production, Appl. Surf. Sci., 2023, 610, 155272 CrossRef CAS
.
- Z. Hu, H. Guo, L. Zhao, Y. Wu, S. Wang and L. Wan, Enhanced efficiency in dye-sensitized solar cells with La(OH)3: Yb3+/Er3+ and large particles scatting layer hybrid double photoanodes, J. Mater. Sci.: Mater. Electron., 2018, 29, 7692–7700 CrossRef CAS
.
- X. Wu, K. Li, Y. Li and G. Zhang, Motivating visible light photocatalytic activity of ultrathin Bi2O2(OH)xCl2−x solid solution with exposed {001} facets by the co-effect of oxygen vacancy and OH replacement, Nanoscale, 2018, 10, 15294–15302 RSC
.
- S. Li, X. Huang, Z. Wan, J. Liu, L. Lu and K. Peng, Green synthesis of ultrapure La(OH)3 nanoparticles by one-step method through spark ablation and electrospinning and its application to phosphate removal, Chem. Eng. J., 2020, 388, 124373 CrossRef CAS
.
- L. Yang, J. Guo, T. Yang, C. Guo, S. Zhang, S. Luo, W. Dai, B. Li, X. Luo and Y. Li, Self-assembly Cu2O nanowire arrays on Cu mesh: A solid-state, highly-efficient, and stable photocatalyst for toluene degradation under sunlight, J. Hazard. Mater., 2021, 402, 123741 CrossRef CAS PubMed
.
- C. Xu, Q. Zhou, W. Huang, K. Yang, Y. Zhang, T. Liang and Z. Liu, Constructing Z-scheme β-Bi2O3/ZrO2 heterojunctions with 3D mesoporous SiO2 nanospheres for efficient antibiotic remediation via synergistic adsorption and photocatalysis, Rare Met., 2022, 41, 2094–2107 CrossRef CAS
.
- W. Huang, Y. Tan, C. Zhang, Q. Zhou, K. Yang, Y. Zhang, D. Li and D. D. Dionysiou, In situ decoration of La(OH)3 on polyethyleneimine linked dendritic mesoporous silica nanospheres targeting at efficient and simultaneous removal of phosphate and Congo red, Environ. Sci.: Nano, 2021, 8, 3792–3805 RSC
.
- X. Zeng, X. Gong, Y. Wan, R. He and Z. Xu, Formation of Oxygen Vacancies on the {010} Facets of BiOCl and Visible Light Activity for Degradation of Ciprofloxacin, Chem. Res. Chin. Univ., 2018, 34, 711–718 CrossRef CAS
.
- X. Lu, W. Che, X. Hu, Y. Wang, A. Zhang and F. Deng, The facile fabrication of novel visible-light-driven Z-scheme CuInS2/Bi2WO6 heterojunction with intimate interface contact by in situ hydrothermal growth strategy for extraordinary photocatalytic performance, Chem. Eng. J., 2019, 356, 819–829 CrossRef CAS
.
- W. Hou, J. Yang, H. Xu, D. Li, Z. Zou and D. Xia, Syntheses of nymphaea-like BiOCl with oxygen vacancies for effective removal of tetracycline hydrochloride, CrystEngComm, 2020, 22, 3956–3964 RSC
.
- E. Jiang, N. Song, X. Zhang, L. Yang, C. Liu and H. Dong, In-situ fabrication of Z-scheme Bi3O4Cl/Bi12O17Cl2 heterostructure by facile pH control strategy to boost removal of various pollutants in water, Chem. Eng. J., 2020, 388, 123483 CrossRef CAS
.
- X. Xu, F. Deng, P. Shao, D. D. Dionysiou, X. Luo and X. Li, Internal electric field driving separation and migration of charge carriers via Z-scheme path in AgIn5S8/ZnO heterojunction for efficient decontamination of pharmaceutical pollutants, Chem. Eng. J., 2022, 428, 132096 CrossRef CAS
.
- B. Tan, Y. Fang, Q. Chen, X. Ao and Y. Cao, Construction of Bi2O2CO3/Ti3C2 heterojunctions for enhancing the visible-light photocatalytic activity of tetracycline degradation, J. Colloid Interface Sci., 2021, 601, 581–593 CrossRef CAS PubMed
.
- G. Yang, Y. A. Zhu, Y. Liang, J. Yang, K. Wang and Z. Zeng, Crystal defect-mediated {010} facets of Bi2MoO6 nanosheets for removal of TC: Enhanced mechanism and degradation pathway, Appl. Surf. Sci., 2021, 539, 148038 CrossRef CAS
.
- M. Li, C. Lai, H. Yi, D. Huang, L. Qin and X. Liu, Multiple charge-carrier transfer channels of Z-scheme bismuth tungstate-based photocatalyst for tetracycline degradation: Transformation pathways and mechanism, J. Colloid Interface Sci., 2019, 555, 770–782 CrossRef CAS
.
- M. Shen, Z. Huang, X. Luo, Y. Ma, C. Chen and X. Chen, Activation of persulfate for tetracycline degradation using the catalyst regenerated from Fenton sludge containing heavy metal: Synergistic effect of Cu for catalysis, Chem. Eng. J., 2020, 396, 125238 CrossRef CAS
.
- Z. J. Xie, Y. P. Feng, F. L. Wang, D. N. Chen, Q. X. Zhang, Y. Q. Zeng, W. Y. Lv and G. G. Liu, Construction of carbon dots modified MoO3/g-C3N4 Z-scheme photocatalyst with enhanced visible-light photocatalytic activity for the degradation of tetracycline, Appl. Catal., B, 2018, 229, 96–104 CrossRef CAS
.
- J. Y. Cao, Z. K. Xiong and B. Lai, Effect of initial pH on the tetracycline (TC) removal by zero-valent iron: Adsorption, oxidation and reduction, Chem. Eng. J., 2018, 343, 492–499 CrossRef CAS
.
- F. Guo, X. L. Huang, Z. H. Chen, H. R. Sun and W. L. Shi, Investigation of visible-light-driven photocatalytic tetracycline degradation via carbon dots modified porous ZnSnO3 cubes: Mechanism and degradation pathway, Sep. Purif. Technol., 2020, 253, 117518 CrossRef CAS
.
- J. W. Liu, L. Y. Huang, Y. P. Li, J. Yao, S. X. Shu, L. J. Huang, Y. H. Song and Q. W. Tian, Constructing an S-scheme CuBi2O4/Bi4O5I2 heterojunction for light emitting diode-driven pollutant degradation and bacterial inactivation, J. Colloid Interface Sci., 2022, 621, 295–310 CrossRef CAS
.
- X. Li, J. Xiong, X. Gao, J. Ma, Z. Chen and B. Kang, Novel BP/BiOBr S-scheme nano-heterojunction for enhanced visible-light photocatalytic tetracycline removal and oxygen evolution activity, J. Hazard. Mater., 2020, 387, 121690 CrossRef CAS PubMed
.
- F. Deng, F. Zhong, D. Lin, L. Zhao, Y. Liu and J. Huang, One-step hydrothermal fabrication of visible-light-responsive AgInS2/SnIn4S8 heterojunction for highly-efficient photocatalytic treatment of organic pollutants and real pharmaceutical industry wastewater, Appl. Catal., B, 2017, 219, 163–172 CrossRef CAS
.
- D. Xiang, X. Hao and Z. Jin, Cu/CdS/MnOx nanostructure-based photocatalyst for photocatalytic hydrogen evolution, ACS Appl. Nano Mater., 2021, 4, 13848–13860 CrossRef CAS
.
- W. Zhao, J. Zhang, F. Zhu, F. Mu, L. Zhang and B. Dai, Study the photocatalytic mechanism of the novel Ag/p-Ag2O/n-BiVO4 plasmonic photocatalyst for the simultaneous removal of BPA and chromium (VI), Chem. Eng. J., 2019, 361, 1352–1362 CrossRef CAS
.
- B. Men, J. Zhang, C. Diao, X. Li, X. Liu and H. Zheng, Photoluminescence, surface photovoltage and photocatalytic properties of BaBiO3 powders, J. Mater. Sci.: Mater. Electron., 2018, 29, 12729–12734 CrossRef CAS
.
- J. Sun, X. Li, Q. Zhao and B. Liu, Ultrathin nanoflake-assembled hierarchical BiOBr microflower with highly exposed {001} facets for efficient photocatalytic degradation of gaseous ortho-dichlorobenzene, Appl. Catal., B, 2021, 281, 119478 CrossRef CAS
.
- L. Huang, L. Yang, Y. Li, C. Wang, Y. Xu and L. Huang, p-n BiOI/Bi3O4Cl hybrid junction with enhanced photocatalytic performance in removing methyl orange, bisphenol A, tetracycline and Escherichia coli, Appl. Surf. Sci., 2020, 527, 146748 CrossRef CAS
.
- K. Jing, W. Ma, Y. Ren, J. Xiong, B. Guo and Y. Song, Hierarchical Bi2MoO6 spheres in situ assembled by monolayer nanosheets toward photocatalytic selective oxidation of benzyl alcohol, Appl. Catal., B, 2019, 243, 10–18 CrossRef CAS
.
- X. Yu, J. Huang, J. Zhao, S. Liu, D. Xiang and Y. Tang, Efficient visible light photocatalytic antibiotic elimination performance induced by nanostructured Ag/AgCl@Ti3+-TiO2 mesocrystals, Chem. Eng. J., 2021, 403, 126359 CrossRef CAS
.
- Q. Zhou, Y. Huang, Y. Zhang, C. Xu, W. Huang and Y. Kang, Insight into the growth mechanism of AgIn5S8 nanoparticles in a low temperature co-precipitation process and their visible-light-driven photocatalytic activities, Mater. Chem. Phys., 2022, 276, 125333 CrossRef CAS
.
- M. Lu, Q. Li, C. Zhang, X. Fan, L. Li and Y. Dong, Remarkable photocatalytic activity enhancement of CO2 conversion over 2D/2D g-C3N4/BiVO4 Z-scheme heterojunction promoted by efficient interfacial charge transfer, Carbon, 2020, 160, 342–352 CrossRef CAS
.
- W. Huang, S. Wang, Q. Zhou, X. Liu, X. Chen and K. Yang, Constructing novel ternary composites of carbon quantum dots/Bi2MoO6/graphitic nanofibers with tunable band structure and boosted photocatalytic activity, Sep. Purif. Technol., 2019, 217, 195–205 CrossRef CAS
.
- L. Yang, X. Bai, J. Shi, X. Du, L. Xu and P. Jin, Quasi-full-visible-light absorption by D35-TiO2/g-C3N4 for synergistic persulfate activation towards efficient photodegradation of micropollutants, Appl. Catal., B, 2019, 256, 117759 CrossRef CAS
.
|
This journal is © The Royal Society of Chemistry 2023 |