Highly efficient detection of ciprofloxacin with a self-powered sensing device based on a Au NPs/g-C3N4 micron tube and a 3D Ni-doped ZnIn2S4 thin film†
Received
20th September 2022
, Accepted 23rd November 2022
First published on 24th November 2022
Abstract
As a second-generation fluoroquinolone antibiotic, ciprofloxacin (CIP) has been widely used in recent years, which allows it enter the water environment and food chain through various ways, causing serious harm to human health and the ecological environment. It is urgent to explore ultrasensitive and maneuverable monitoring methods to solve the environmental pollution caused by excessive use of CIP. In this study, a self-powered sensing device was fabricated based on a photoelectrochemical (PEC) system and 3D printing technology, which could generate electrical output to provide a sensing signal under photoirradiation, without an external power source, displaying highly efficient detection of CIP. In this system, n-type Au nanoparticles/graphite carbon nitride (g-C3N4) micron tube-modified fluorine tin oxide (FTO) conductive glass slides served as the photoanode for the oxidation of CIP under photoirradiation while p-type Ni-doped ZnIn2S4 film-modified FTO was employed as the cathode for the reduction of dissolved oxygen. A thiolated CIP binding aptamer was loaded on the surface of the photoanode to ensure selectivity. Combining photoactive materials and the aptamer, the as-obtained sensing platform can achieve the sensitive and specific recognition of CIP under complex environmental conditions. The open-circuit voltage (OCP) was sensitive to CIP in a wide concentration range (0.2–3840 ng mL−1) and had a low detection limit at 0.03 ng mL−1. This strategy paves the way to a simple approach for the determination of CIP in sewage and several commercial pure milk samples.
Environmental significance
Ciprofloxacin (CIP) is widely employed in agricultural industries. Its uncontrollable and extensive use allow CIP to enter the environment through various approaches, causing many tricky problems to the ecological environment. Many analysis technologies have been developed for monitoring CIP, but they suffer from high costs, complex detection procedures and technical requirements. Therefore, exploring ultrasensitive and maneuverable methods to detect CIP is urgently required. This work constructed a novel self-powered photoelectrochemical aptasensor with a double photoelectrode system. The Au NPs/g-C3N4 nanotube photoanode showed excellent photoelectric conversion efficiency and selectivity and could produce a large bias voltage with a Ni-doped ZnIn2S4 thin-film photocathode, realizing the self-powered ultrasensitive detection of CIP in sewage. The proposed sensor displayed an excellent performance with a wide concentration range (0.2–3840 ng mL−1) and a low detection limit of 0.03 ng mL−1, which shows great potential for application in environmental monitoring.
|
1. Introduction
Ciprofloxacin (CIP) is a fluoroquinolone antibiotic widely used in aquaculture and animal husbandry. According to a survey, approximately 70% of CIP is transferred to the environment in a variety of ways,1 and its improper usage may promote quinolone resistance of zoonotic pathogens and cause some adverse effects in humans through the food chain.2 Considering the high exposure to and potential risks of CIP, it is valuable to monitor CIP concentrations in the environment. Up to now, the majority of analytical methods such as fluorescence spectroscopy,3,4 high-performance liquid chromatography (HPLC)5 and enzyme-linked immunoassay6 have been able to measure CIP with high sensitivity and specificity, but they either require skilled personnel and costly instruments or rely on the quality of antibodies, limiting their practical application and development. Thus, a maneuverable, economical and convenient method for monitoring CIP concentrations in environmental samples remains essential.
In this study, a dual-electrode self-powered sensing technology arises at a historic moment. This is a novel technology based on a photoelectrochemical (PEC) system, which combines the dual advantages of photocatalysis and electrochemical analysis. By designing the photosensitive materials and sensing strategies, only two electrodes are required to construct the detection platform, which can simultaneously achieve ultrasensitive detection of the target and conversion of chemical energy to electrical energy. Gao et. al. developed a PEC-based self-powered sensor for aflatoxin B1 detection, which was composed of a NaYF4:Yb,Er/Bi2S3 photoanode and a Pt cathode.7 The proposed double-electrode self-powered sensing technology overcomes the shortcoming of traditional sensing technology requiring external energy input and a three-electrode system,8,9 dramatically simplifies the analysis equipment and promotes the development of sensing devices toward miniaturization and portability, which shows great potential for application in food safety analysis and environmental monitoring. In addition, micro-nano three-dimensional (3D) printing technology has revolutionized the development of sensing devices, allowing the creation of customized devices that can be adapted to a variety of application scenarios.10 Qiu et al. developed a dual-channel aptasensor using a spatially-resolved technique on a homemade 3D printed device, providing a promising tool for carcinoembryonic antigen detection.11 Coincidentally, a novel 3D printed device for biomarker monitoring was developed based on λ-exonuclease-assisted recycling amplification.12 Inspired by this, a portable sensing device was developed by combining micro-nano 3D printing technology with PEC-based sensing strategies for the first time.
Although this is a novel and ingenious combination, it remains a challenge to integrate a PEC-based sensor into a micromodel while maintaining its high sensitivity, and developing photoelectrodes with high activity plays a crucial role in the construction of sensing equipment.7,13 Most of the reported research studies have focused on a single-photoelectrode system wherein only the anode is the photosensitive material while the cathode is Pt or an electrocatalyst.14–16 However, this kind of cathode has suffered from the high cost of noble metals and external power, so it is reasonable to design a double photoelectrode system wherein both the anode and the cathode are modified by photosensitive materials. The self-bias caused by the Fermi level difference between photoelectrodes would cause the photoinduced electrons on the photoanode to transfer to the photocathode through an external circuit.16,17 Meanwhile, the target oxidation reaction and oxygen reduction reaction occur on the different photoelectrodes, respectively, realizing the detection and energy output synergistic effect. Therefore, exploring photosensitive materials with matching band structures and excellent photoelectric performance is crucial.
Graphitic carbon nitride (g-C3N4) micron tubes, an environmentally friendly n-type semiconductor material compared to other common g-C3N4 in the form of nanosheets, nanorods or nanoflowers,18,19 exhibit excellent separation/transfer kinetics of photoinduced carriers, as well as increased light utilization through repeated light scattering/reflection inside the tubular structure, making them suitable as the primary photosensitive material. However, single g-C3N4 tubes still suffer from a narrow visible light utilization range (< about 450 nm), shows a limited photoelectric conversion efficiency owing to the fast photoinduced carrier recombination and insufficient active sites. Incorporating Au NPs is a feasible approach due to the plasma resonance energy transfer (PRET) between Au NPs and g-C3N4 micron tubes; the visible light absorption capability of the g-C3N4 micron tubes can be significantly increased, and the photoelectric conversion efficiency can be further improved.20 In addition, Au NPs can also play the role of a binding site of the aptamer through the Au–S bond, which provides the recognition unit for the analyte.21 Coupling Au NPs with g-C3N4 micron tubes can function as a double-win strategy that simultaneously enhances the photoactivity and selectivity. Furthermore, hexagonal ZnIn2S4, a ternary p-type chalcogenide semiconductor, has been deemed as an ideal candidate for a PEC-based sensor because of its unique optical characteristics and adjustable morphology.22 In particular, the suitable band structure of ZnIn2S4 is beneficial to generate a large bias to construct a double photoelectrode system with a Au/g-C3N4 photoanode, making it the preferred material for the photocathode design. With the aim of designing a satisfactory photocathode, metal cations (Fe3+, Co2+ or Ni2+) can be doped to single ZnIn2S4 because these ions serve as active sites for the interfacial transfer of photoinduced carriers.23–25
Based on this, we proposed a 3D printed self-powered double photoelectrode device consisting of an n-type Au nanoparticle (NP) modified g-C3N4 micron tube photoanode and a p-type Ni-doped ZnIn2S4 thin-film photocathode. A thiolated CIP binding aptamer able to precisely capture the analyte was immobilized on the photoanode through Au–S. Under photoirradiation, CIP was oxidized by active photogenerated holes that accumulated at the photoanode while oxygen was facilely reduced at the photocathode, leading to the generation of power output that provides a responsive signal without external electrical power. This interesting sensing system relies on integrating the highly active photocatalyst and the specific aptamer, which can detect CIP with ultra-sensitivity and display high tolerance for various interferences. Furthermore, to demonstrate the practicability of the proposed sensor, the levels of CIP in sewage and commercial pure milk samples were measured and the results were quite accurate.
2. Experimental
2.1 Reagents and apparatus
The reagents and apparatus are detailed in the ESI† files.
2.2 Synthesis of photosensitive materials
The synthesis method of the g-C3N4 micron tube was performed according to an article we have reported before26 with some modification. 0.8 g melamine was added in 80 mL ultrapure water and magnetically stirred for 1 h. Then, the mixed solution was transferred to a 100 mL Teflon-lined autoclave and heated to 180 °C for 10 h. After the bottom setting was removed and washed with ultrapure water several times and dried at 60 °C for 8 h, white needle-like crystals can be observed. To obtain hollow tubular g-C3N4, 0.8 g of the above-mentioned needle-like crystals was put into a quartz boat with a cover and then heated to 500 °C at a rate of 2.5 °C min−1 in a tube furnace under a N2 atmosphere and maintained at this temperature for 2 h. After cooling down to room temperature, hollow tubular g-C3N4 was obtained. The synthesis method of the Au nanoparticle modified g-C3N4 micron tube is detailed in the ESI† files (section 3.1).
The Ni-doped ZnIn2S4 thin-film modified photocathode was synthesized in situ on a FTO conductive glass (1 cm × 2 cm) by a one-step hydrothermal method.27 Before the reaction, the FTO glass was cleaned with ethanol and water by ultrasonication for 30 min and soaked in a mixed acid solution (H2O2:H2SO4, v:v = 1
:
3) for 100 s and then finally rinsed with ultrapure water. 0.12 mmol ZnSO4·7H2O, 0.24 mmol InCl3·4H2O, 0.48 mmol C2H5NS and different masses of NiCl2·6H2O were dissolved in 30 mL ultrapure water. After magnetic stirring for 30 min, the mixed solution was transferred to the Teflon-lined autoclave encapsulated with a slice of cleaned FTO conductive glass at the bottom; the autoclave was heated to 160 °C for 12 h, cooled to room temperature naturally, and then the obtained FTO glass was taken out and rinsed with ultrapure water several times. Finally, the Ni-doped ZnIn2S4 thin-film photocathode was obtained by vacuum drying at 50 °C for 8 h. For comparison, the same method was used to prepare the ZnIn2S4 thin film, with the only difference being NiCl2·6H2O was not added in the solution. A possible formation mechanism of Ni-doped ZnIn2S4 thin-film modified FTO with three-dimensional (3D) reticulate structures is proposed in the ESI† files (section 3.3). The preparation process is demonstrated in Scheme 1A and B.
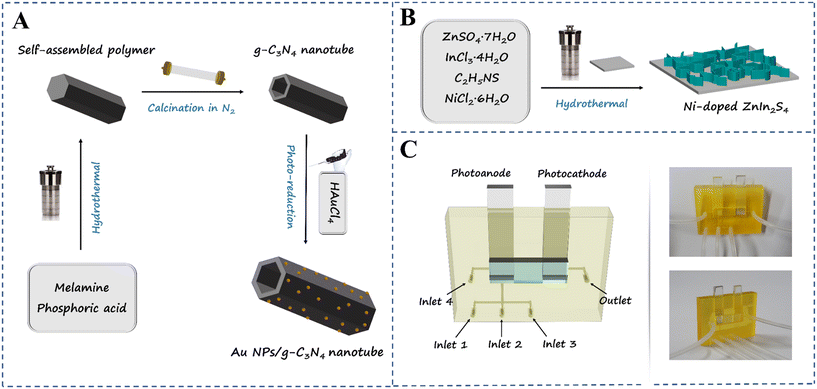 |
| Scheme 1 The preparation process of the (A) Au NPs/g-C3N4 micron tube and (B) Ni-doped ZnIn2S4 thin-film photocathode, and (C) the model diagram (left) and actual object figure (right) of the 3D printed sensing device. | |
2.3 Construction of a 3D printed double photoelectrode sensing platform
The Au NPs/g-C3N4 tube composite modified FTO electrode and the in situ grown Ni-doped ZnIn2S4 thin-film FTO electrode were prepared as the photoanode and photocathode, respectively, and the preparation details are given in the ESI† files (section 3 to 5). The 3D micromodel was designed by SolidWorks software and printed using a microArch S240 micro-stereolithography 3D printing system. The microchannels were treated with oxygen plasma treatment for 30 s to obtain better hydrophilicity, and then the synthesized photoelectrodes were fixed in the working tank of the micromodel.
To improve the specificity of the sensing platform, 20 μL thiolated CIP binding aptamer solution (3.0 μM) was injected into the Au NPs/g-C3N4 micron tube photoanode from inlet 1, holding for 30 min at 4 °C to prepare highly selective probes through the Au–S bond; the nonspecific sites were blocked by injecting 20 μL MCH solution (1 mM) from inlet 2, holding for 30 min, and then these were marked as the Apt/Au NPs/g-C3N4 micron tube and the MCH/Apt/Au NPs/g-C3N4 micron tube, respectively. During the detection progress, 20 μL TE buffer solution with a different concentration of CIP was injected into the surface of the MCH/Apt/Au NPs/g-C3N4 micron tube photoanode from inlet 3, holding for 40 min at 37 °C, and then marked as the CIP/Apt/Au NPs/g-C3N4 micron tube. At every step of the preparation process, the photoanode was washed with phosphate-buffered saline (PBS) from inlet 4. The actual object figure is shown in Scheme 1C. The PEC measurements were performed using an electrochemical workstation (CHI 660E), and a one-compartment quartz cell with 30 mL PBS (0.1 M, pH 7.4) electrolyte solution served as the reaction vessel. A 300 W Xenon arc lamp (PLS/SXE 300C, Beijing Perfectlight Co., Ltd.) was used as the irradiation source.
3. Results and discussion
3.1 Design principle of the self-powered double photoelectrode sensing device
There is an internal bias between the two photoelectrodes with different Fermi levels, which can drive the photoinduced electrons of the photoanode to transfer to the photocathode through an external circuit, forming a loop and generating electricity.28 It is found that the reaction between the target and the electrode would affect the efficiency of photoinduced electron transmission, resulting in a corresponding change of the output performance.29 On this basis, a linear relationship between the concentration of the target and the output performance can be obtained.
The Au NPs/g-C3N4 micron tube modified FTO and Ni-doped ZnIn2S4 thin-film modified FTO were utilized as the photoanode and the photocathode, respectively, for stable generation of sensing signals. The g-C3N4 micron tube is an environmentally friendly 3D semiconductor material with a unique hollow structure, making the incident light reflect and refract many times, resulting in a visible light absorption capability.30 Because of the localized surface plasmon resonance (LSPR) effect, the strong local electromagnetic field on the surface of Au NPs induces plasmonic oscillation, which leads to the photoinduced electrons remaining on Au NPs, improving the charge separation rate.31 In addition, the introduction of Au NPs also provides a binding site for aptamers, ensuring high selectivity. The Ni-doped ZnIn2S4 thin film, a typical p-type semiconductor with a reticular lamellar structure, can generate a large bias with Au/g-C3N4 and enforces fast transmission of photoinduced electrons. The detailed principle is described as follows: the Au NPs/g-C3N4 micron tube photoanode can generate strong oxidizing photoinduced holes under visible light and further produce highly active hydroxyl radicals (˙OH), which can directly oxidize CIP molecules (Fig. 1).32,33 The processes are shown below:
| Au NPs/g-C3N4 + hv → h+ + e− | (1) |
|  | (3) |
| 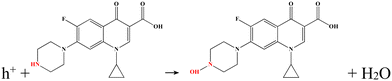 | (4) |
Meanwhile, the photoinduced electrons that accumulated in the conduction band (CB) of the g-C
3N
4 micron tube and on the surface of Au NPs transferred to the Ni-doped ZnIn
2S
4 thin-film photocathode through the external circuit and then reacted with oxygen as a reducing agent.
34 Such a synergy effect can facilitate the separation of photoinduced electron–hole pairs and improve the output performance of the system. In order to obtain the output signals simply, the OCP of this system was used to study the analysis results quantitatively.
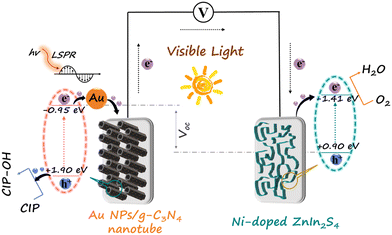 |
| Fig. 1 The sensing mechanism of the proposed self-powered double photoelectrode sensing device. | |
3.2 Material characterization
Fig. 2 shows typical scanning electron microscopy (SEM) images of the synthesized g-C3N4 micron tube and the Au NPs/g-C3N4 micron tube. The pure g-C3N4 micron tube exhibits a vimineous hollow tubular structure with a smooth surface (Fig. 2A and B). As shown in Fig. 2C, the surface of the g-C3N4 micron tube became rougher, which was caused by the newly formed Au NPs. Besides, the high-magnification images in Fig. 2C, E and F confirmed that there were many nanoparticles with a diameter of 10 nm anchored in the tube wall. The EDS spectra and the elemental mapping images (Fig. 2D) revealed that Au, C and N elements were evenly distributed on the tubular structure. Under irradiation, both the hollow tubular structure of g-C3N4 and the LSPR effect generated by Au NPs can bring enhanced light absorption, thus significantly improving the photoelectric response of the photoanode, which could be further proved by the UV-vis diffuse reflectance spectra (DRS) in Fig. 5A.
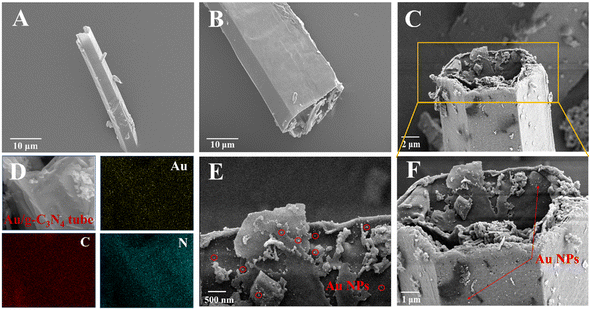 |
| Fig. 2 SEM images of the (A and B) pure g-C3N4 micron tube and (C, E and F) Au NPs/g-C3N4 micron tube; (D) the high-angle annular dark field-scanning transmission electron microscopy (HAADF-STEM) image and energy dispersive spectroscopy (EDS) elemental mapping images of the Au NPs/g-C3N4 micron tube. | |
Moreover, Fig. 3A and B show the microstructure and morphology of the Ni-doped ZnIn2S4 thin-film photocathode; the EDS spectrum and the elemental mapping images (Fig. 3C) confirmed the successful doping of Ni. The unique mesh structure shown in Fig. 3B greatly enhances the mass transfer area and efficiency between the electrode and the electrolyte, bringing a reduced carrier recombination rate and improved photoactivity.35
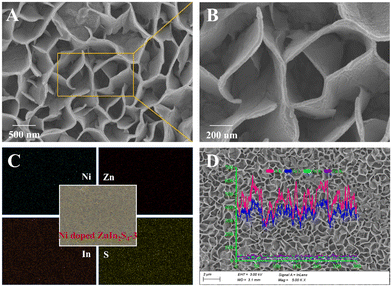 |
| Fig. 3 (A and B) SEM images, (C) HAADF-STEM image and EDS elemental mapping images, and (D) EDS line scanning images of the Ni-doped ZnIn2S4-3 photocathode. | |
The XRD spectra in Fig. 4A also revealed the internal composition of the two electrode materials. The peak at 27.5° can be indexed as the (002) plane of g-C3N4.36 Compared with the pure g-C3N4 micron tube, the Au NPs/g-C3N4 micron tube displays four diffraction peaks at 38.4°, 44.3°, 64.7° and 77.6°, which corresponded to the (111), (200), (220) and (311) crystal planes of the cubic Au nanocrystal (JCPDS card No. 04-0784).37 It can be noted that the peak pattern of the (111) crystal plane is the sharpest, indicating that the Au NPs tend to grow along the lowest energy crystal plane during the reduction process. As can be seen from Fig. 4B, the Ni-doped ZnIn2S4 thin-film photocathode shows three main diffraction peaks indexed to the (102), (104) and (112) crystal planes, which were consistent with hexagonal ZnIn2S4 (JCPDS card No. 72-0773).23 The characteristic peaks of the Ni-doped ZnIn2S4 thin-film photocathode shift towards higher diffraction angles than those of the ZnIn2S4 electrode, showing that Ni was successfully incorporated into the lattices of ZnIn2S4.24 To confirm the Ni doping in ZnIn2S4, the Ni doping concentration of Ni-doped ZnIn2S4-1–5 was determined by inductively coupled plasma mass spectrometry (ICP-MS), and the results are shown in Table S1.† X-ray photoelectron spectroscopy (XPS) was applied to investigate the chemical status of these materials; the full scan spectra of the g-C3N4 micron tube, Au NPs/g-C3N4 micron tube, ZnIn2S4 and Ni-doped ZnIn2S4-3, and the high-resolution spectra of each element are shown in Fig. S2 and S3, respectively.† The details are discussed in the ESI† files (section 4.2).
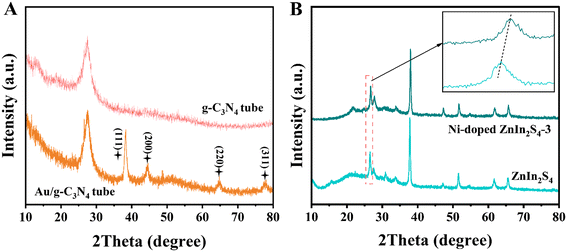 |
| Fig. 4 XRD spectra of the (A) pure g-C3N4 micron tube and Au NPs/g-C3N4 micron tube; (B) XRD spectra of ZnIn2S4 and the Ni-doped ZnIn2S4-3 thin-film photocathode. | |
3.3 Investigation of the energy band structure
UV-vis DRS and Mott–Schottky (MS) analysis were carried out to investigate the light absorption capability and band structure of the electrode materials. From Fig. 5A, the absorption edge of the g-C3N4 micron tube is determined to be about 455 nm, corresponding to a bandgap of about 2.85 eV (Fig. 5C). Compared to ordinary g-C3N4 nanosheets,21 although their absorption edges are almost the same, the absorption capability of the g-C3N4 tube increased due to the multiple light reflections and refractions by the tubular structure. After the Au NPs were incorporated, the absorption edge redshifted to ∼480 nm. Meanwhile, the absorption capability was significantly enhanced in the near-infrared region, and a significant shoulder peak appeared at ∼550 nm, which was attributed to the external oscillating electric field caused by the LSPR effect. As can be seen from Fig. 5B, the light absorption capability of the Ni-doped ZnIn2S4 thin-film electrode is more potent than that of the ZnIn2S4 thin film electrode, indicating that Ni doping has a specific effect on improving the optical performance.27 Among the ZnIn2S4 thin film electrodes with different Ni contents, Ni-doped ZnIn2S4-3 has the strongest light absorption ability and can be deemed as a prospective candidate for constructing the photocathode. In addition, Fig. 5C shows that the bandgap of Ni-doped ZnIn2S4-3 (2.31 eV) was lower than that of the ZnIn2S4 thin film (2.37 eV). This may be because the doping of Ni leads to the formation of the Ni 3d energy level in the valence band (VB) edge of the ZnIn2S4 thin film, which caused a narrowed band gap.38,39 In addition, the MS plots of the g-C3N4 micron tube and Ni-doped ZnIn2S4-3 were measured under a frequency of 2000 Hz to estimate the p–n type and the flat band potential (Ufb). As can be seen from Fig. 5D, the g-C3N4 micron tube showed the n-type nature and Ni-doped ZnIn2S4 showed the p-type nature according to their opposite linear slopes. To obtain the band structures of the g-C3N4 micron tube and Ni-doped ZnIn2S4-3, VB-XPS spectrum was investigated in Fig. 5E. The contact potential difference between the samples and the analyzer (VB potential (vs. NHE)) was estimated by the following equation:40
VB (vs. NHE) = Φ + VB(XPS) − 4.44 |
where Φ is the electron work function of the analyzer, which is 4.20 eV according to the instrument instructions. From Fig. 5E, the VB potentials of the g-C3N4 micron tube, ZnIn2S4 and Ni-doped ZnIn2S4-3 were estimated to be ≈1.90 eV, ≈1.08 eV and ≈0.90 eV, respectively. Hence, their VB potentials (vs. NHE) could be estimated to be ≈1.66, ≈0.84 and ≈0.66 eV, respectively, through the above equation. Combined with the obtained values of Eg, the conduction band (CB) potentials of the g-C3N4 micron tube, ZnIn2S4 and Ni-doped ZnIn2S4-3 thin film were determined to be ≈−1.19 eV, ≈−1.53 eV and ≈−1.65 eV, respectively, according the following equation:
Accordingly, the CB of Ni-doped ZnIn2S4-3 is estimated to be greater than that of ZnIn2S4, indicating an enhanced reduction potential of photoinduced electrons on the CB. The band structures of the g-C3N4 micron tube and Ni-doped ZnIn2S4-3 are shown in Fig. 5F. Obviously, the doping of Ni causes the VB and CB positions to move up, leading to an increased potential difference between the two photoelectrodes, which is conducive to enhancing the photoelectric activity of the 3D printed self-powered double photoelectrode device.
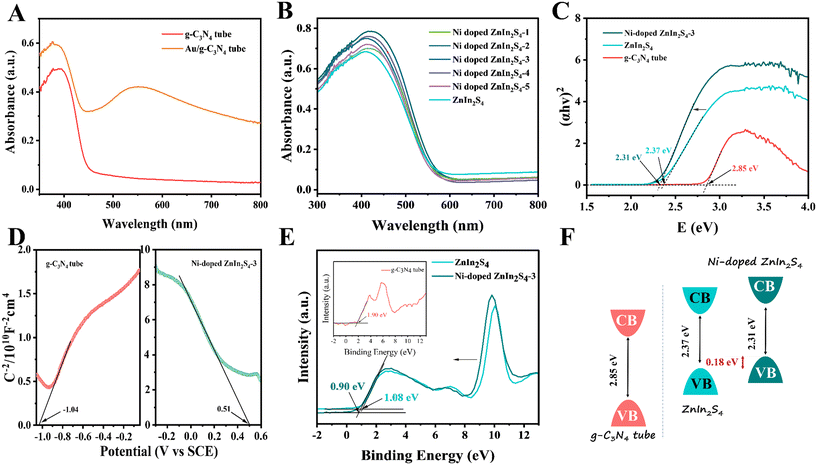 |
| Fig. 5 UV-vis DRS of the (A) pure g-C3N4 micron tube and Au NPs/g-C3N4 micron tube and (B) ZnIn2S4 and Ni-doped ZnIn2S4-1–5 thin-film photocathodes; (C) plots of (αhv)2vs. photon energy of the pure g-C3N4 micron tube, ZnIn2S4 and Ni-doped ZnIn2S4-3 thin-film photocathode; (D) MS plots of the pure g-C3N4 micron tube and Ni-doped ZnIn2S4-3 thin-film photocathode; (E) XPS-VB spectra of the pure g-C3N4 micron tube, ZnIn2S4 and Ni-doped ZnIn2S4-3 thin-film photocathode; (F) the energy band structures of the g-C3N4 micron tube and Ni-doped ZnIn2S4-3. | |
3.4 Photoelectrochemical activity analysis
To investigate the thermodynamic feasibility of the 3D printed self-powered double photoelectrode device, the polarization curves of the photoelectrodes in the same electrolyte (0.1 M PBS, pH 7.4) were measured. As shown in Fig. 6A, under irradiation, the initial potential of the Au NPs/g-C3N4 photoanode was about −0.15 V (curve a). A negatively shifted initial potential was observed (∼0.36 V) after 3840 ng mL−1 CIP was captured on the photoanode (curve b), suggesting that CIP was oxidized by photoinduced holes in the photoanode. As can be seen from Fig. 6B, the reduction reaction of O2 on the photocathode occurred at 0.39 V. It shows that there is a potential difference that exists when the photoanode and photocathode are assembled into a self-powered sensing platform. The photoanode provides a negative potential for the photocathode under irradiation, while the latter provides a positive potential for the former, ensuring the smooth redox reaction on the photoelectrode surfaces. Thus, the sensing platform based on these two photoelectrodes is feasible in terms of thermodynamics. We further recorded the OCP of this 3D printed self-powered double photoelectrode device under intermittent illumination. As depicted in Fig. S4,† the OCP reached ∼0.54 V under illumination, which was significantly higher than the ∼0.15 V under dark conditions. The results are consistent with those obtained from the polarization curves above. Also, it was proved that the proposed 3D printed self-powered double photoelectrode device has good signal stability within 450 s.
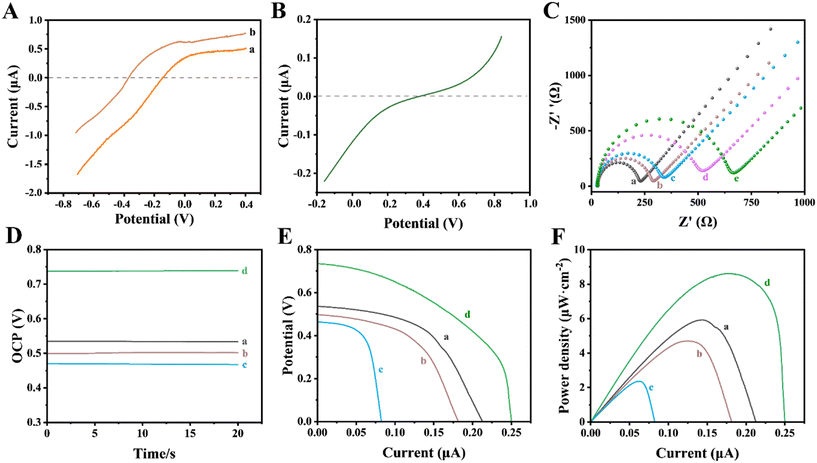 |
| Fig. 6 Polarization curves of the (A) MCH/Apt/Au NPs/g-C3N4 photoanode modified without (a) and with (b) 3840 ng mL−1 CIP under photoirradiation; (B) polarization curve of the Ni-doped ZnIn2S4-3 thin-film photocathode under photoirradiation; (C) EIS curves of the modified photoanodes: (a) Au NPs/g-C3N4, (b) Apt/Au NPs/g-C3N4, (c) Apt/Au NPs/g-C3N4 after MCH molecule blocking, (d) MCH/Apt/Au NPs/g-C3N4 after 160 ng mL−1 CIP was added, and (e) MCH/Apt/Au NPs/g-C3N4 after 1280 ng mL−1 CIP was added; (D) OCP responses, (E) V–I curves and (F) P–I curves of the 3D printed self-powered double photoelectrode device consisting of the Ni-doped ZnIn2S4-3 thin-film photocathode and different photoanodes under photoirradiation: (a) Au NPs/g-C3N4, (b) Apt/Au NPs/g-C3N4, (c) Apt/Au NPs/g-C3N4 after MCH molecule blocking, and (d) MCH/Apt/Au NPs/g-C3N4 after 3840 ng mL−1 CIP was added. | |
Electrochemical impedance spectroscopy (EIS) was performed to demonstrate the changes in surface properties during the photoanode modification. As shown in Fig. 6C, the charge transfer resistance (Rct) value of the Apt/Au NPs/g-C3N4 photoanode (curve b) was higher than that of the Au NPs/g-C3N4 photoanode (curve a), because the negative charge on the surface of the aptamer (a single-strand DNA) would hinder the absorption of [Fe(CN)6]3−/4− with the same electronegative properties.41 Owing to the weak electron-transfer ability caused by the steric hindrance effect, an increased Rct value was observed after MCH molecule blocking (curve c).42 When CIP of a different concentration interacts with the aptamer, the Rct value increases further. More importantly, CIP with a higher concentration (curve e) would cause a greater change than that with a lower concentration (curve d), indicating that the Rct values increase with the increase of CIP loading on the photoanode surface. Besides, the photoelectric properties of the 3D printed self-powered double photoelectrode device was analyzed by means of OCP, V–I and P–I curves. As shown in Fig. 6D–F, the output property of the system decreased during the modification of the photoanode. Compared with the primary Au NPs/g-C3N4 photoanode (0.54 V, curve a, Fig. 6D), the OCP values of the Apt/Au NPs/g-C3N4 photoanode (curve b, Fig. 6D) and the MCH/Apt/Au NPs/g-C3N4 photoanode (curve c, Fig. 6D) decreased to 0.50 V and 0.47 V, while the maximum power density (Pmax) values decreased to 4.82 μW cm−2 (curve b, Fig. 6F) and 2.23 μW cm−2 (curve c, Fig. 6F), respectively. These were in accord with the results of EIS analysis showing that an increased Ret value leads to a decreased output performance. However, an obvious increase in OCP (curve d, Fig. 6D) and Pmax (curve d, Fig. 6F) was observed after 3840 ng mL−1 CIP was captured on the photoanode surface, because the photoinduced holes with a strong oxidation capability would oxidize CIP directly on the surface of the photoanode,43 promoting the transfer of photoinduced electrons to the photocathode. The increased electron density in the loop resulted in enhanced output performance. On this basis, a double photoelectrode sensing strategy was proposed for CIP detection.
3.5 Optimization
In order to achieve optimal sensing performance, several experimental parameters were explored, including the mass concentration of Au NPs (ω (Au NPs)) in the photoanode, the concentration of the thiolated CIP binding aptamer, the pH value of the electrolyte and the incubation time of CIP. ω (Au NPs) has a more significant impact on the sensing performance because it can regulate the photoelectric efficiency of the photoanode. As can be seen from Fig. S5A,† when ω (Au NPs) was 10 wt%, the OCP value reached the maximum because introducing an appropriate amount of Au NPs can improve the light absorption capability of the photoanode and transport more photoinduced electrons into the loop. However, excessive Au NPs may reduce its light absorption capacity due to the agglomeration phenomenon, resulting in the reduction of photoinduced electrons in the loop. Thus, the optimal (ω (Au NPs)) in the photoanode is 10 wt%. In addition, the optimal pH value of the PBS electrolyte solution was investigated. Fig. S5B† shows that the maximum OCP response was obtained at pH 7.4, so the PBS electrolyte solution of pH 7.4 was used in this work. The concentration of the thiolated CIP binding aptamer was investigated. Fig. S5C† shows that the OCP value was induced with the increased thiolated CIP binding aptamer concentration from 0 to 5.0 μM and then reached a plateau at 3.0 μM. This may be due to the aptamer attachment sites reaching saturation. So the optimal CIP aptamer concentration was 3.0 μM. As can be seen from Fig. S5D,† the OCP value increased with the increase of incubation time of CIP (160 ng mL−1) and tended to be stable after 40 min, which can be ascribed to a shorter time not being conducive to the fixation and oxidation of CIP.
3.6 Measurement of CIP with different concentrations
A 3D printed self-powered double photoelectrode device was constructed under the optimal conditions above. To obtain the analysis results more easily, the OCP signal was used to study its sensing performance for CIP detection. As shown in Fig. 7A, the values of the OCP signal gradually increased with the increase in concentration of CIP. There were two linear segments with different slopes within the range of 0.2–3840 ng mL−1 (Fig. 7B); one of the corresponding linear equations was OCP(V) = 8 × 10−4Cciprofloxacin + 0.478 (R2 = 0.9891, Cciprofloxacin: 0.2–160 ng mL−1) and the other was OCP(V) = 3 × 10−5Cciprofloxacin + 0.6094 (R2 = 0.9916, Cciprofloxacin: 160–3840 ng mL−1). The piecewise linear function with two different slopes can be ascribed to the steric effect brought about by the aptamer–CIP complexes. On this basis, the limit of detection (LOD) was calculated to be 0.03 ng mL−1 (S/N = 3), which is lower than those of other previously reported techniques (Fig. 7C).44–50,32
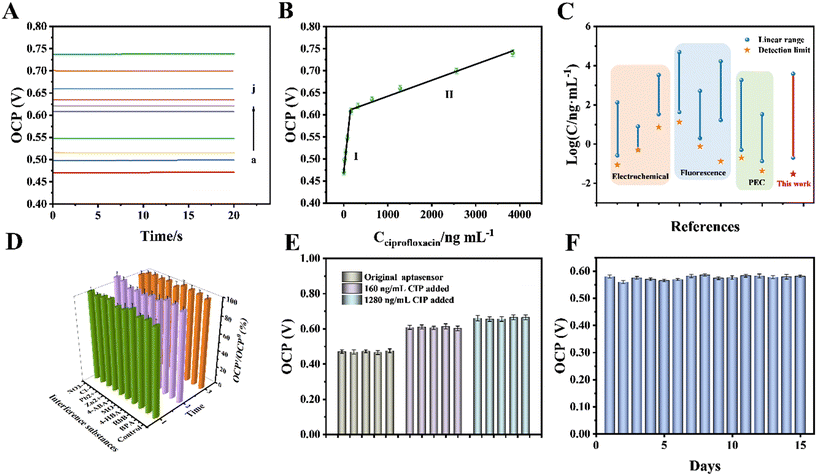 |
| Fig. 7 (A) OCP responses of the proposed sensor recorded at different CIP concentrations (from a to j): 0.2, 20, 40, 80, 160, 320, 640, 1280, 2560, and 3840 ng mL−1; (B) calibration curve for CIP detection; (C) comparison of the analytical performances of several reported methods for CIP detection; (D) OCP responses of the proposed sensor towards 160 ng mL−1 CIP obtained before (OCP0) and after (OCP′) adding a 100-fold concentration of interference substances; (E) the reproducibility of the photoelectrodes; (F) long stability of the proposed sensor. | |
3.7 Selectivity, stability and feasibility
To further evaluate the sensing property of the double photoelectrode sensing platform, the response signals of some interference substances such as antibiotics (bisphenol A (BPA), rhodamine B (RhB), 4-hydroxybenzoic acid (4-HBA), methyl orange (MO) and 4-aminobenzoic acid (4-ABA)) and inorganic metal ions (Zn2+, Pb2+, Cl−, NO3−) that may coexist in the actual water sample were investigated. As shown in Fig. 7D, the OCP responses towards 160 ng mL−1 CIP and a 100-fold concentration of interference substances were studied. There were no obvious changes observed in the sensing of CIP, suggesting that these interference substances do not influence CIP determination, which was attributed to the excellent specificity of the CIP binding aptamer. Similarly, the reproducibility of the electrodes was studied by recording the OCP signals of five parallel electrodes for different concentrations of CIP under similar experimental conditions. The results displayed in Fig. 7E reveal that the relative standard deviation (RSD) was 3.6%, indicating the stable sensing property of the proposed sensing platform. Meanwhile, the long stability of the proposed sensor was explored by recording the OCP signals every day. As can be seen from Fig. 7F, the sensor still retained 93.5% of its original OCP signals after 15 days, suggesting its excellent long stability.
To evaluate its feasibility, the proposed double photoelectrode sensing platform was applied to detect CIP levels in sewage and several commercial pure milk samples. The sewage samples were from the Xiangjiang sewage outlet, and four different milk samples from different companies were purchased from local supermarkets. One of the biggest advantages of this sensing strategy is the ease of preliminary sample treatment: 5 mL glacial acetic acid and 20 mL ethanol were added to 100 mL milk samples to dissolve CIP and precipitate protein. Afterwards, the mixture solution and sewage samples were centrifuged to obtain the supernatant. Finally, the obtained supernatant was filtered through a membrane filter (0.45 μm) to remove the impurities before detection. The accuracy of this sensor was investigated through spiking certain amounts of CIP in the sewage and milk samples, which were measured under the optimal experimental conditions. To evaluate the accuracy of the proposed self-powered aptasensing device for the analysis of real samples, we compared the measurement results with those from the High Performance Liquid Chromatography (HPLC) standard method. As shown in Table S2,† the proposed sensor showed good recoveries ranging from 95.05–108.06%, and the RSD value was lower than 4.65%, indicating that the accuracy of this new double photoelectrode sensing platform is qualified. The experimental results presented in Table S2† suggest that the proposed sensor is satisfactory in the detection of CIP, and it can be applied to CIP detection in the natural environment.
4. Conclusion
In this work, by coupling the Au NPs/g-C3N4 micron tube modified FTO that functioned as the photoanode and the Ni-doped ZnIn2S4 films modified FTO as the photocathode, we developed a 3D printed self-powered double photoelectrode device for the detection of CIP. We found that the signal value of OCP is highly dependent on the concentration of CIP in the photoanode. The highly photoelectroactive materials ensure sensitivity, and the CIP binding aptamer immobilized on the photoanode guarantees selectivity. Under optimal conditions, the 3D printed self-powered double photoelectrode device exhibits high anti-interference capability and shows a wide linear range (0.2–3840 ng mL−1) with a low LOD of 0.03 ng mL−1. In conclusion, the developed sensing device achieved sensitive detection of CIP without any expensive instrument, which greatly promotes the development of sensing devices towards miniaturization and portability.
Conflicts of interest
There are no conflicts to declare.
Acknowledgements
This study was financially supported by Projects U22A20617 and 51521006 by the National Natural Science Foundation of China. the National Innovative Talent Promotion Program of China (2017RA2088), the National Key Research and Development Program of China (No. 2021YFC1910400), and the Funds for Innovative Province Construction of the Hunan Province of China (2019RS3012).
References
- X. Liu, T. Wang, Y. Lu, W. Wang, Z. Zhou and Y. Yan, Constructing carbon dots and CdTe quantum dots multi-functional composites for ultrasensitive sensing and rapid degrading ciprofloxacin, Sens. Actuators, B, 2019, 289, 242–251 CrossRef CAS.
- C. Liu, S. Mao, M. Shi, F. Wang, M. Xia, Q. Chen and X. Ju, Peroxymonosulfate activation through 2D/2D Z-scheme CoAl-LDH/BiOBr photocatalyst under visible light for ciprofloxacin degradation, J. Hazard. Mater., 2021, 420, 126613 CrossRef CAS PubMed.
- R. Bosma, J. Devasagayam, A. Singh and C. M. Collier, Microchip capillary electrophoresis dairy device using fluorescence spectroscopy for detection of ciprofloxacin in milk samples, Sci. Rep., 2020, 10, 13548 CrossRef CAS PubMed.
- J. Tan, L. Liu, F. Li, Z. Chen, G. Y. Chen, F. Fang, J. Guo, M. He and X. Zhou, Screening of Endocrine Disrupting Potential of Surface Waters via an Affinity-Based Biosensor in a Rural Community in the Yellow River Basin, China, Environ. Sci. Technol., 2022, 56, 14350–14360 CrossRef CAS PubMed.
- N. Rezaee Moghadam, S. R. Arefhosseini, A. Javadi, F. Lotfipur, M. Ansarin, E. Tamizi and M. Nemati, Determination of Enrofloxacin and Ciprofloxacin Residues in Five Different Kinds of Chicken Tissues by Dispersive Liquid-Liquid Microextraction Coupled with HPLC, Iran. J. Pharm. Res., 2018, 17, 1182–1190 Search PubMed.
- F. Wang, N. Li, Y. Zhang, X. Sun, M. Hu, Y. Zhao and J. Fan, Preparation and Directed Evolution of Anti-Ciprofloxacin ScFv for Immunoassay in Animal-Derived Food, Foods, 2021, 10, 1933 CrossRef CAS PubMed.
- J. Gao, X. Yao, Y. Chen, Z. Gao and J. Zhang, Near-Infrared Light-Induced Self-Powered Aptasensing Platform for Aflatoxin B1 Based on Upconversion Nanoparticles-Doped Bi2S3 Nanorods, Anal. Chem., 2021, 93, 677–682 CrossRef CAS PubMed.
- Z. Yu, H. Gong, Y. Li, J. Xu, J. Zhang, Y. Zeng, X. Liu and D. Tang, Chemiluminescence-Derived Self-Powered Photoelectrochemical Immunoassay for Detecting a Low-Abundance Disease-Related Protein, Anal. Chem., 2021, 93, 13389–13397 CrossRef CAS PubMed.
- Z. Yu, G. Cai, R. Ren and D. Tang, A new enzyme immunoassay for alpha-fetoprotein in a separate setup coupling an aluminium/Prussian blue-based self-powered electrochromic display with a digital multimeter readout, Analyst, 2018, 143, 2992–2996 RSC.
- X. Ouyang, C. Feng, X. Zhu, Y. Liao, Z. Zhou, X. Fan, Z. Zhang, L. Chen and L. Tang, 3D printed bionic self-powered sensing device based on fern-shaped nitrogen doped BiVO4 photoanode with enriched oxygen vacancies, Biosens. Bioelectron., 2022, 220, 114817 CrossRef PubMed.
- Z. Qiu, J. Shu, J. Liu and D. Tang, Dual-Channel Photoelectrochemical Ratiometric Aptasensor with up-Converting Nanocrystals Using Spatial-Resolved Technique on Homemade 3D Printed Device, Anal. Chem., 2019, 91, 1260–1268 CrossRef CAS PubMed.
- K. Zhang, S. Lv and D. Tang, Novel 3D Printed Device for Dual-Signaling Ratiometric Photoelectrochemical Readout of Biomarker Using lambda-Exonuclease-Assisted Recycling Amplification, Anal. Chem., 2019, 91, 10049–10055 CrossRef CAS PubMed.
- M. Zhang, Z. Zhang, J. Wei, Z. Dai, N. Hao and K. Wang, A dual-photoelectrode photofuel cell based self-powered aptasensor using a multimeter as a direct visual readout strategy, Chem. Commun., 2021, 57, 5973–5976 RSC.
- M. Sun, Y. Zhu, K. Yan and J. Zhang, Dual-mode visible light-induced aptasensing platforms for bleomycin detection based on CdS-In2S3 heterojunction, Biosens. Bioelectron., 2019, 145, 111712 CrossRef CAS PubMed.
- L. He, Q. Liu, S. Zhang, X. Zhang, C. Gong, H. Shu, G. Wang, H. Liu, S. Wen and B. Zhang, High sensitivity of TiO2 nanorod array electrode for photoelectrochemical glucose sensor and its photo fuel cell application, Electrochem. Commun., 2018, 94, 18–22 CrossRef CAS.
- Q. Zeng, J. Bai, J. Li, Y. Li, X. Li and B. Zhou, Combined nanostructured Bi 2 S 3/TNA photoanode and Pt/SiPVC photocathode for efficient self-biasing photoelectrochemical hydrogen and electricity generation, Nano Energy, 2014, 9, 152–160 CrossRef CAS.
- K. E. Guima, L. E. Gomes, J. Alves Fernandes, H. Wender and C. A. Martins, Harvesting Energy from an Organic Pollutant Model Using a New 3D-Printed Microfluidic Photo Fuel Cell, ACS Appl. Mater. Interfaces, 2020, 12, 54563–54572 CrossRef CAS PubMed.
- K. Zhang, S. Lv, Q. Zhou and D. Tang, CoOOH nanosheets-coated g-C3N4/CuInS2 nanohybrids for photoelectrochemical biosensor of carcinoembryonic antigen coupling hybridization chain reaction with etching reaction, Sens. Actuators, B, 2020, 307, 127631 CrossRef CAS.
- S. Lv, Y. Li, K. Zhang, Z. Lin and D. Tang, Carbon Dots/g-C3N4 Nanoheterostructures-Based Signal-Generation Tags for Photoelectrochemical Immunoassay of Cancer Biomarkers Coupling with Copper Nanoclusters, ACS Appl. Mater. Interfaces, 2017, 9, 38336–38343 CrossRef CAS PubMed.
- W. Hong, Q. Cai, R. Ban, X. He, C. Jian, J. Li, J. Li and W. Liu, High-Performance Silicon Photoanode Enhanced by Gold Nanoparticles for Efficient Water Oxidation, ACS Appl. Mater. Interfaces, 2018, 10, 6262–6268 CrossRef CAS PubMed.
- X. Ouyang, L. Tang, C. Feng, B. Peng, Y. Liu, X. Ren, X. Zhu, J. Tan and X. Hu, Au/CeO2/g-C3N4 heterostructures: Designing a self-powered aptasensor for ultrasensitive detection of Microcystin-LR by density functional theory, Biosens. Bioelectron., 2020, 164, 112328 CrossRef CAS PubMed.
- Q. Liang, W. Gao, C. Liu, S. Xu and Z. Li, A novel 2D/1D core-shell heterostructures coupling MOF-derived iron oxides with ZnIn2S4 for enhanced photocatalytic activity, J. Hazard. Mater., 2020, 392, 122500 CrossRef CAS PubMed.
- G. Ma, C. Shang, M. Jin, L. Shui, Q. Meng, Y. Zhang, Z. Zhang, H. Liao, M. Li, Z. Chen, M. Yuan, X. Wang, C. Wang and G. Zhou, Amorphous Ti(iv)-modified flower-like ZnIn2S4 microspheres with enhanced hydrogen evolution photocatalytic activity and simultaneous wastewater purification, J. Mater. Chem. C, 2020, 8, 2693–2699 RSC.
- B. Qiu, P. Huang, C. Lian, Y. Ma, M. Xing, H. Liu and J. Zhang, Realization of all-in-one hydrogen-evolving photocatalysts via selective atomic substitution, Appl. Catal., B, 2021, 298, 120518 CrossRef CAS.
- H. Qian, Z. Liu, J. Ya, Y. Xin, J. Ma and X. Wu, Construction homojunction and co-catalyst in ZnIn2S4 photoelectrode by Co ion doping for efficient photoelectrochemical water splitting, J. Alloys Compd., 2021, 867, 159028 CrossRef CAS.
- Y. Zhang, M. Zhang, L. Tang, J. Wang, Y. Zhu, C. Feng, Y. Deng, W. He and Y. Hu, Platinum like cocatalysts tungsten carbide loaded hollow tubular g-C3N4 achieving effective space separation of carriers to degrade antibiotics, Chem. Eng. J., 2020, 391, 123487 CrossRef CAS.
- B. Fan, Z. Chen, Q. Liu, Z. Zhang and X. Fang, One-pot hydrothermal synthesis of Ni-doped ZnIn2S4 nanostructured film photoelectrodes with enhanced photoelectrochemical performance, Appl. Surf. Sci., 2016, 370, 252–259 CrossRef CAS.
- A. Rubino, R. Zanoni, P. G. Schiavi, A. Latini and F. Pagnanelli, Two-Dimensional Restructuring of Cu2O Can Improve the Performance of Nanosized
n-TiO2/p-Cu2O Photoelectrodes under UV-Visible Light, ACS Appl. Mater. Interfaces, 2021, 13, 47932–47944 CrossRef CAS PubMed.
- X. Ouyang, C. Feng, L. Tang, X. Zhu, B. Peng, X. Fan, Y. Liao, Z. Zhou and Z. Zhang, A flexible photoelectrochemical aptasensor using heterojunction architecture of α-Fe2O3/d-C3N4 for ultrasensitive detection of penbritin, Biosens. Bioelectron., 2022, 197, 113734 CrossRef CAS PubMed.
- Z. Sun, W. Wang, Q. Chen, Y. Pu, H. He, W. Zhuang, J. He and L. Huang, A hierarchical carbon nitride tube with oxygen doping and carbon defects promotes solar-to-hydrogen conversion, J. Mater. Chem. A, 2020, 8, 3160–3167 RSC.
- M. Shang, Y. Gao, J. Zhang, J. Yan and W. Song, Signal-on cathodic photoelectrochemical aptasensing of insulin: Plasmonic Au activated amorphous MoSx photocathode coupled with target-induced sensitization effect, Biosens. Bioelectron., 2020, 165, 112359 CrossRef CAS PubMed.
- C. Yuan, Z. He, Q. Chen, X. Wang, C. Zhai and M. Zhu, Selective and efficacious photoelectrochemical detection of ciprofloxacin based on the self-assembly of 2D/2D g-C3N4/Ti3C2 composites, Appl. Surf. Sci., 2021, 539, 148241 CrossRef CAS.
- W. Lai, Z. Chen, S. Ye, Y. Xu, G. Xie, C. Kuang, Y. Li, L. Zheng and L. Wei, BiVO4 prepared by the sol–gel doped on graphite felt cathode for ciprofloxacin degradation and mechanism in solar-photo-electro-Fenton, J. Hazard. Mater., 2021, 408, 124621 CrossRef CAS PubMed.
- Y. Fujishima, S. Okamoto, M. Yoshiba, T. Itoi, S. Kawamura, Y. Yoshida, Y. Ogura and Y. Izumi, Photofuel cell comprising titanium oxide and bismuth oxychloride (BiO1−xCl1−y) photocatalysts that uses acidic water as a fuel, J. Mater. Chem. A, 2015, 3, 8389–8404 RSC.
- C. Feng, L. Tang, Y. Deng, J. Wang, Y. Liu, X. Ouyang, H. Yang, J. Yu and J. Wang, A novel sulfur-assisted annealing method of g-C3N4 nanosheet compensates for the loss of light absorption with further promoted charge transfer for photocatalytic production of H2 and H2O2, Appl. Catal., B, 2021, 281, 119539 CrossRef CAS.
- C. Feng, L. Tang, Y. Deng, J. Wang, J. Luo, Y. Liu, X. Ouyang, H. Yang, J. Yu and J. Wang, Synthesis of leaf-vein-like g-C3N4 with tunable band structures and charge transfer properties for selective photocatalytic H2O2 evolution, Adv. Funct. Mater., 2020, 30, 2001922 CrossRef CAS.
- L. Zhang, Z. Luo, R. Zeng, Q. Zhou and D. Tang, All-solid-state metal-mediated Z-scheme photoelectrochemical immunoassay with enhanced photoexcited charge-separation for monitoring of prostate-specific antigen, Biosens. Bioelectron., 2019, 134, 1–7 CrossRef CAS PubMed.
- S. C. Das, R. J. Green, J. Podder, T. Z. Regier, G. S. Chang and A. Moewes, Band Gap Tuning in ZnO Through Ni Doping via Spray Pyrolysis, J. Phys. Chem. C, 2013, 117, 12745–12753 CrossRef CAS.
- C. Feng, Z. P. Wu, K. W. Huang, J. Ye and H. Zhang, Surface modification of 2D photocatalysts for solar energy conversion, Adv. Mater., 2022, 34, 2200180 CrossRef CAS PubMed.
- H. Yu, R. Shi, Y. Zhao, T. Bian, Y. Zhao, C. Zhou, G. I. N. Waterhouse, L. Z. Wu, C. H. Tung and T. Zhang, Alkali-Assisted Synthesis of Nitrogen Deficient Graphitic Carbon Nitride with Tunable Band Structures for Efficient Visible-Light-Driven Hydrogen Evolution, Adv. Mater., 2017, 29, 1605148 CrossRef PubMed.
- K. Mao, H. Zhang, Z. Wang, H. Cao, K. Zhang, X. Li and Z. Yang, Nanomaterial-based aptamer sensors for arsenic detection, Biosens. Bioelectron., 2020, 148, 111785 CrossRef CAS PubMed.
- B. Peng, Y. Lu, J. Luo, Z. Zhang, X. Zhu, L. Tang, L. Wang, Y. Deng, X. Ouyang, J. Tan and J. Wang, Visible light-activated self-powered photoelectrochemical aptasensor for ultrasensitive chloramphenicol detection based on DFT-proved Z-scheme Ag2CrO4/g-C3N4/graphene oxide, J. Hazard. Mater., 2021, 401, 123395 CrossRef CAS PubMed.
- P. Yan, J. Dong, Z. Mo, L. Xu, J. Qian, J. Xia, J. Zhang and H. Li, Enhanced photoelectrochemical sensing performance of graphitic carbon nitride by nitrogen vacancies engineering, Biosens. Bioelectron., 2020, 148, 111802 CrossRef CAS PubMed.
- L. Xu, H. Li, P. Yan, J. Xia, J. Qiu, Q. Xu, S. Zhang, H. Li and S. Yuan, Graphitic carbon nitride/BiOCl composites for sensitive photoelectrochemical detection of ciprofloxacin, J. Colloid Interface Sci., 2016, 483, 241–248 CrossRef CAS PubMed.
- K. Abnous, N. M. Danesh, M. Alibolandi, M. Ramezani, S. M. Taghdisi and A. S. Emrani, A novel electrochemical aptasensor for ultrasensitive detection of fluoroquinolones based on single-stranded DNA-binding protein, Sens. Actuators, B, 2017, 240, 100–106 CrossRef CAS.
- T. Madrakian, S. Maleki and A. Afkhami, Surface decoration of cadmium-sulfide quantum dots with 3-mercaptopropionic acid as a fluorescence probe for determination of ciprofloxacin in real samples, Sens. Actuators, B, 2017, 243, 14–21 CrossRef CAS.
- X. Hu, K. Y. Goud, V. S. Kumar, G. Catanante, Z. Li, Z. Zhu and J. L. Marty, Disposable electrochemical aptasensor based on carbon nanotubes-V2O5-chitosan nanocomposite for detection of ciprofloxacin, Sens. Actuators, B, 2018, 268, 278–286 CrossRef CAS.
- V. D. Dang, A. B. Ganganboina and R. A. Doong, Bipyridine- and Copper-Functionalized N-doped Carbon Dots for Fluorescence Turn Off-On Detection of Ciprofloxacin, ACS Appl. Mater. Interfaces, 2020, 12, 32247–32258 CrossRef CAS PubMed.
- P. Gayen and B. P. Chaplin, Selective Electrochemical Detection of Ciprofloxacin with a Porous Nafion/Multiwalled Carbon Nanotube Composite Film Electrode, ACS Appl. Mater. Interfaces, 2016, 8, 1615–1626 CrossRef CAS PubMed.
- Y. Song, J. Bai, R. Zhang, H. He, C. Li, J. Wang, S. Li, Y. Peng, B. Ning, M. Wang and Z. Gao, Michael-Addition-Mediated Photonic Crystals Allow Pretreatment-Free and Label-Free Sensoring of Ciprofloxacin in Fish Farming Water, Anal. Chem., 2017, 90, 1388–1394 CrossRef PubMed.
|
This journal is © The Royal Society of Chemistry 2023 |