End-of-life MoS2-enabled device and material transformation in landfill leachate and its effect on the landfill microbiome†
Received
27th July 2022
, Accepted 13th December 2022
First published on 15th December 2022
Abstract
The safety of nano-enabled devices must be assessed throughout the device lifecycle. Landfills are the end-of-life repository for functional devices; however, the dynamism of leachate age and complexity of leachate chemistry make material safety assessments challenging. This study evaluates the transformation and effects of water treatment membranes and transistors enabled with MoS2—a transition metal dichalcogenide (TMD) used in electronic transistors and futuristic wearable electronics—in complex landfill conditions. Device decay and MoS2 transformation are studied with a suite of characterization tools. The effects of MoS2 colloids on microbial diversity in young and mature landfill leachates are investigated. Results indicate that MoS2-enabled membranes and transistors become attached to residues from the leachate but do not undergo major chemical decay. Furthermore, complex environments like leachates are robust, resisting microbiome changes upon exposure to MoS2. The results obtained under the specific study conditions indicate that disposal of MoS2-enabled devices in landfills does not induce significant deleterious effects on the landfill in the absence of photo-transformation (as is the case in covered landfills). However, further studies are needed to assess whether a high concentration of MoS2, a likely result of its accumulation in the landfill over time, has the potential to substantially change the leachate microbiome.
Environmental significance
Since nearly 60% of nanomaterial (NM) release is expected to occur after disposal into a landfill, evaluating nano-enabled device transformation under realistic landfill conditions is particularly important for environmental health and safety (EHS). Though nano-EHS research space is rich in fate, transport, and transformation studies, there is a paucity of literature that evaluates device-embedded NM fate in realistic environmental conditions. This work is highly environmentally relevant, as it considers two devices that are usually landfilled, and it assesses MoS2 release from these devices and evaluates impacts on these when exposed to field-collected landfill leachate samples (on both young and aged leachate). Moreover, the study assesses the role of MoS2 on the landfill-relevant microbiome—which is key to proper functioning of bioreactor municipal landfills. The findings indicate that under the tested conditions, MoS2–embedded membranes and devices do not leach NM from the matrices and thus will not impart significant deleterious impacts to the leachate chemistry or microbiome.
|
Introduction
The footprint that a process or device embodies in its life-cycle includes its energy signature and toxicity manifestation to humans and the environment.1 Stakeholder-accepted sustainable engineering thus necessitates minimum impact to the ecosphere, when a process or device is transferred from the technosphere.2 At its core, sustainable engineering includes material risk mitigation by either utilizing inherently safer nanomaterials (NMs) or properly embedding NMs to prevent their release.1 Because nearly 60% of NM release is expected to occur after disposal into a landfill,3 the “do nano right”1 movement requires an understanding of nano-safety in a complex landfill environment. This is particularly important for materials incorporated into electronics and polymeric membranes used in the water treatment industry.
The nano-environmental health and safety (EHS) literature has extensively studied NM mass-flow throughout its lifecycle,4–6 including device recycling processes.6 These studies have proposed the utilization of multiple attractive frameworks for material selection (e.g., Ashby selection strategy7) and big-data analysis tools.8 At the center of these approaches is the notion of reliable NM fate, transport, transformation, and toxicity data. However, the nano-EHS literature is sparse in such NM safety and release data for complex environmental matrices.1 Although mesocosm studies9 have been conducted on numerous NMs10–12 and in a myriad of environmental systems (e.g., simulated freshwater wetland13,14 or indoor aquatic mesocosm15), end-of-life NM release and fate remain severely understudied.1
Global life cycle analysis of NMs has estimated that 63–91% of over 260
000–309
000 metric tons of NMs can end up in municipal solid waste (MSW) landfills4 over a year (i.e., 2010 for this study). Thus, this environmental system is likely the largest repository of NMs. Because landfills are designed with the objective of receiving waste over time, a constant increase in nano-enabled devices, as well as an increase in the concentration of NMs released into the landfill environment, is likely. Thus, the retention of NMs in a device or the risk from NMs when released from a device must be evaluated under landfill-relevant conditions.
Landfills are acutely complex systems. A series of biological and chemical reactions takes place after refuse is buried in a landfill.16 Therefore, the chemistry of leachate generated from a landfill depends on age (due to these reactions), refuse type, and landfilling technology.16 Natural organic matter (NOM), inorganic macrocomponents (e.g., common metals and ligands), heavy metals, and xenobiotic organic compounds (e.g., aromatic hydrocarbons, pesticides, plasticizers) vary widely (e.g., pH: 4.5–9; NOM: 30–29
000 mg L−1 total organic carbon, total solids: 2000–60
000 mg L−1);16 concentrations of these in a landfill often are 1000 to 5000 times those found in groundwater. Leachate composition changes over time—e.g., from >95% volatile fatty acids in young leachate17 to higher molecular weight compounds and humic-like substances17,18 but almost no volatile fatty acids in mature leachate. Such dynamism in leachate composition and chemistry makes it critical for understanding NM release in these complex systems to generate reliable nano-EHS data.
Only a handful of studies have assessed NM fate and their effects in a landfill environment. Mobility and colloidal stability of surface-modified semiconductor quantum dots (QDs) were studied in landfill leachate over a 6 month time period, where leachate composition and QD surface modification were identified as critical in controlling their fate.19 Moreover, degradation of devices in a landfill environment is even less studied. One study evaluated solar photovoltaics in leachates, comparing the dissolution of metals in synthetic leachates (with varying pH) and in a single real leachate sample.20 Systematic assessment of the release of NMs—particularly of those in the two-dimensional (2D) NM family—from nano-enabled devices and their effects in a landfill are needed.
MoS2, one of the most studied 2D NMs, is a transition metal dichalcogenide (TMD) and presents unique advantages for electronics (e.g., tunable bandgap, high carrier mobility, and improved mechanical properties)21,22 and water treatment membranes (e.g., providing molecular selectivity).23 These TMDs present high surface area and distinctive chemical features, which might affect their fate and transport in a landfill environment. MoS2 nanosheets that are well-exfoliated or have increased layer spacing exhibit high adsorption capacities by exposing a large percentage of the sulfur atoms.24 Sulfur from MoS2 in complex systems can attract soft-acid and heavy metal species, due to its soft Lewis base character, while resisting other cations.25 Other mechanisms for adsorption include ion exchange24 or multilayer interactions. The dissolution kinetics of MoS2 also can be unique. For example, its route of exfoliation and crystalline phases (i.e., 1T vs. 2H crystalline phases) have been identified as important predictors of its oxidative dissolution tendencies.26
TMDs can undergo environmental transformations, such as oxidation, under certain conditions.26 Such transformations might affect the leachate microbiome. Abundant and diverse populations of microorganisms exist in landfills, including Pseudomonas, Balneola, Fluviicola, Cetobacterium, Acholeplasma, and Halocella, with some playing key roles in organic contaminant degradation, carbon cycling, and denitrification.27 Changes in the microbial diversity due to environmental or engineered stressors likely can impact important landfill processes, such as methanogenesis.28 The biological toxicity of NMs is directly related to the chemical and physical properties of the particle, which influence cellular uptake or interactions. Upon interaction with 2D NMs having thin, active edge sites, microbial cells might get deformed and damaged.29 For instance, the edge roughness of 2-D graphene was proposed to facilitate penetration of these NMs across the bacterial cell membrane.30 Studies on MoS2 toxicity reveal mixed messages; while generally non-toxic at low concentrations,31,32 one study has shown that low-dose exposure to MoS2 can inhibit efflux pump activities of human hepatoma HepG2 cells by damaging the plasma membrane and releasing soluble species.33 A long-term exposure to a sub-lethal dose of high-activity NMs might lead to changes in the microbial activities of landfill leachate.28,34
The objective of this study is to investigate NM transformation (i.e., state of stability and rate of dissolution) and release from MoS2-embedded electronic transistors and desalination membranes. Field leachate samples and real nano-enabled devices are utilized to conduct a detailed and systematic nano-EHS study. Material characterization is conducted with Raman spectroscopy, scanning electron microscopy (SEM), Attenuated Total Reflection-Fourier Transform Infrared (ATR-FTIR) spectroscopy, and Inductively Coupled Plasma-Optical Emission Spectroscopy (ICP-OES). Time-Of-Flight Secondary Ion Mass Spectrometry (TOF-SIMS) is used to evaluate chemical changes in the devices. This study not only presents detailed results of the fate of an important 2D NM under complex landfill conditions but also evaluates NM release from simple nano-enabled electronic devices and membranes and its effects on the leachate microbiome. Furthermore, the methodology developed for handling NMs in a complex environmental system can be broadly utilized for assessing nano-enabled device safety.
Materials and methods
Collection, storage, and characterization of MSW landfill leachate
Young-aged leachate (YL), approximately 2 years old, and mature-aged Leachate (ML), approximately 30 years old, were collected from the Waste Management Austin Community Landfill on 2020/12/01. The leachate was collected from a collection sump at the landfill in high density polyethylene (HDPE) containers, transported on ice to the laboratory, and stored at 4 °C (with nitrogen purging conducted to replace the ingressed oxygen during pumping). Prior to removing an aliquot of leachate for an experiment, the HDPE container was bubbled with nitrogen and shaken or inverted to resuspend the settled solids. Nitrogen was bubbled through the leachate again before sealing the container. Common water quality analyses (total dissolved and suspended solids (TDS, TSS), pH, conductivity, alkalinity, chemical oxygen demand (COD), and five-day biochemical oxygen demand (BOD5)) were conducted on the leachate samples as per APHA 2005 and Standard Methods for the Examination of Water and Wastewater. Total organic carbon (TOC) was measured by combustion catalytic oxidation (Shimadzu, TOC-L TOC Analyzer, Shimadzu Scientific Instruments, Columbia, MD). Leachates were digested with microwave-assisted digestion (Milestone Ethos UP, Sorisole (BG), Italy) in a mixture of 32% v/v HNO3 and 7% v/v HCl before ICP-OES analysis for cations. Once digested, the samples were stored at 4 °C before further dilution to 3.2% v/v HNO3 and 0.7% v/v HCl. This acid composition was used to prepare further dilutions of samples as well as ICP-OES standards. Quantification of a suite of 17 elements was conducted with ICP-OES for their respective ionic concentrations, which include Na, Mg, Al, Si, K, Ca, Ti, Cr, Mn, Fe, Co, Ni, Cu, Zn, Mo, Ag, and Pb. Anions in the leachates also were quantified by ion chromatography (Dionex ICS-2100; Dionex IonPac AS19 column; 20–45 mM KOH eluent; 1 mL min−1 eluent flow rate). Samples were pre-treated by filtering through a 0.45 μm PTFE syringe filter, and appropriate dilutions were made with deionized (DI) water.
Preparation of MoS2 powder, MoS2-enabled devices, and membranes
Preparation of MoS2 powder.
In brief, 1 g bulk MoS2 (Sigma-Aldrich, St. Louis, MO) powder was dispersed in 100 mL of a 45
:
55 v/v mixture of anhydrous ethanol and DI water and sonicated for 5 h with a probe ultrasonicator (0.25 in microtip probe: 4420, sonicator: Q700, Qsonica LLC, Newtown, CT) in an ice bath. An amplitude setting of 80% was set for probe sonication in a pulsed mode with 8 s on and 2 s off. The suspension was subsequently centrifuged at 380 × g to separate unexfoliated bulk materials. The collected supernatant was centrifuged at 11
648 × g at 18 °C and resuspended in DI water to isolate the few-layered particles in an aqueous medium. This aliquot was frozen at −80 °C before freeze drying (Labconco, Kansas City, MO) for at least 36 h. The dried, few-layered MoS2 was collected and stored in glass scintillation vials, which were wrapped in aluminum foil to avoid photodegradation.
Preparation of MoS2-enabled devices.
Simple MoS2-enabled devices were fabricated by exfoliating MoS2 crystals (HQ Graphene, Groningen, The Netherlands) onto polydimethylsiloxane (PDMS) following a previously developed protocol.35 After identifying monolayers with Raman spectroscopy and photoluminescence (PL) measurements, each selected flake was transferred onto the Si/SiO2 substrate. The Si substrate is of the p+ type, <0.005 Ω cm, <100> Si with 125 nm of SiO2 (University Wafers, South Boston, MA). The samples were annealed at 9.33 × 10−5 Pa and 200 °C for 6 h to reduce induced strain on the MoS2 flake.
Preparation of MoS2-enabled membranes.
For thin film composite (TFC) membrane fabrication, 1,3-phenylenediamine (MPD, >99%, Spectrum Chemicals, St. Louis, MO), and 1,3,5-benzenetricarbonyltrichloride (TMC, 98%, Spectrum Chemicals, St. Louis, MO) were used. Isopar-G, a proprietary non-polar organic solvent, was obtained from Univar (Redmond, WA). For preparation of the thin film nanocomposite membranes with MoS2, a procedure was developed based on previously published protocols.36 To generate the active layer (with or without MoS2) on hand-cast polysulfone support (see details in ESI†), the pre-cut support was taped to a glass plate and immersed into 3.5 wt% aqueous MPD solution at a 20° angle for 2 min to expose the top of the membrane to MPD. Next, the membrane was withdrawn, and excess MPD was removed from the surface. The plate was subsequently dried and immersed in a 0.15 wt% TMC solution for 1 min. For MoS2-TFC membranes, MoS2 was added to the TMC solution at 0.02 wt%. During this step, the MPD in the membrane diffused out of the membrane and cross-linked with TMC, or with TMC and MoS2, at the water/solvent interface to form the polyamide active layer on the membrane. The glass plate was withdrawn from the TMC solution, allowed to sit for 2 min, and then immersed into simmering (∼95 °C) DI water for 2 min. The membrane was immersed in a sodium hypochlorite solution (1.5 mL of reagent grade 10–15% v/v solution in 1 L of DI water) for 2 min and then was transferred to a 1000 ppm aqueous sodium bisulfate solution for 30 s and finally to simmering DI water (∼95 °C) for 2 min.
Exposure of MoS2-enabled materials to MSW leachate
Exposure of MoS2-enabled membranes and devices in young and mature MSW leachates.
MoS2-enabled and control membranes (with no MoS2) were cut into 0.5′′ × 2.5′′ pieces with a sterile blade and weighed prior to exposure to YL or ML. Two pieces of membrane—one with low and another with high MoS2 concentration, based on visual observation of color of the membranes—were individually weighed and placed in a single anaerobic culture tube. The leachates were pre-filtered with Whatman ashless filters to remove large solids. Aliquots of filtered YL (diluted 2×) and filtered ML (undiluted), 20 mL volumes, were added to the tubes and sealed with Suba-Seal® septa. The membrane samples and devices were exposed to the leachates for 8 weeks. The exposure duration is chosen to simulate MoS2 release during the long retention expected in landfills and during leachate treatment.37 The tubes were sparged with nitrogen and then placed in a shaker at 150 rpm at 25 °C. Duplicate samples were included for each time point.
Physical changes on the membranes and devices were imaged with a FEI Quanta 650 SEM (FEI, Hillsboro, OR). Before SEM observation, the membranes were dried under nitrogen in a desiccator, and then sputter-coated with gold (Electron Microscopy Sciences, Hatfield, PA). FT-IR spectra were collected using a Thermo Nicolet FTIR to identify changes to the surface functional groups during interaction with organic matter in the leachates. At least three measurements per sample were acquired to ensure reproducibility. Structural changes to the MoS2 were probed with Raman spectroscopy using a HORIBA LabRAM HR Evolution Raman spectrometer, equipped with a 532 nm excitation laser. All spectra were recorded at 9 different locations on the membrane to ensure reproducibility. FTIR and Raman peaks were identified and processed using Origin Pro 2021 software. The photo-luminescence (PL) spectra of TMD on the devices before and after exposure to the leachates were recorded on a Photon Technology International system with a Newport RSP-1T solid sample holder. Samples were excited at 400 nm, and emission spectra were collected from 700–425 nm at a 1 nm step size, with 1 nm excitation and emission slits. The Mo released into the leachates represented the sum of fragments released from the membranes as particulates as well as ions. To quantify the dissolved fraction, ultrafiltration (3 kDa Vivaspin® 6 Centrifugal Filters) was employed for 20 min at 4500 × g. Total Mo was measured by using ICP-OES on microwave digested samples in 4% v/v HNO3 and 1% v/v HCl (30 min, 1800 W).
Landfill leachate microbial community analysis.
The effect of colloidal TMDs on microbial diversity was determined. Stock suspensions with high MoS2 concentration (up to 5000 mg L−1) were prepared by dispersing exfoliated MoS2 powder in filtered leachates with probe sonication. The concentrated stock was added to appropriate volumes of YL and ML to achieve final concentrations of 1 mg L−1 and 100 mg L−1 with a total volume of 50 mL. The control samples contained only MSW leachate. After flushing with N2 to create an anaerobic environment, the tubes were sealed and incubated in a shaker (150 rpm) at 25 °C. All treatments were conducted for up to 12 weeks.37,38 Over time, sample aliquots were removed and filtered with a 0.2 μm polycarbonate membrane (Pall Life Sciences; Ann Arbor, MI). DNA was extracted using the DNeasy PowerSoil Kit (Qiagen; Germantown, MD) per the manufacturer's protocol. The membrane containing the filtered solids was placed into the PowerSoil PowerBead tube using tweezers. DNA was quantified with a NanoDrop spectrophotometer and stored at −20 °C until analysis. DNA was sequenced and processed as described in Rowles et al.39 In brief, DNA sequencing was conducted by Miseq at MR DNA (Molecular Research LP; Shallowater, TX), where the 16S rRNA gene V4–V5 variable region was amplified with primers 515F (5′-GTGYCAGCMGCCGCGGTAA-3′) and 926R (5′-CCGYCAATTYMTTTRAGTTT-3′). The MR DNA analysis pipeline (MR DNA; Shallowater, TX) produced the sequence data, which were joined and cleaned up (where fragments <150 bp and those with ambiguous base calls were removed). After de-noising of the sequences, zero-radius operational taxonomic units (zOTUs) were generated, and chimeras were removed. zOTUs were taxonomically classified using Basic Local Alignment Search Tool Nucleotide (BLASTn) against a curated database derived from the National Center for Biotechnology Information (NCBI) and Ribosomal Database Project.40 A phylogenetic tree was created by combining zOTU and taxonomy tables in the R environment (code in ESI† document 1 (ref. 41)) using the Phyloseq and Ape packages.42,43 The GUniFrac package was employed to calculate the UniFrac distance at α = 0.5and principal coordinate analysis (PCoA) was completed using the Vegan package.44–46 The parameter α controls the weighting of abundant lineages, and the resulting UniFrac distance is reported to be robust. The Adonis method from the R Vegan package and R pairwiseAdonis package47 were used for permutational multivariate analysis of variance (PERMANOVA) and pairwise statistical comparisons.
Results and discussion
Leachate characteristics
Results from the water quality analyses conducted on YL and ML are presented in Table 1. In general, higher values are observed for YL than for ML. Differences between YL and ML are most profound for alkalinity, oxygen demand, and TOC. Some of the cations and anions (such as Na+, K+, Ca2+, NO3−, PO43−) are present at much higher concentrations in YL, resulting in a higher conductivity value. Other cations, i.e., Si4+, Mg2+, and Fe3+, and anionic ligands, i.e., Cl−, and SO42−, are detected at comparable concentrations in YL and ML.
Table 1 Leachate characteristics (average and standard deviations of at least 2 measurements) measured for YL and ML collected from a local MSW landfill. Concentrations are stated in mg L−1 unless otherwise specified
Parameter |
Young leachate (YL) |
Mature leachate (ML) |
pH |
7.9 ± 0.1 pH units |
7.5 ± 0.1 pH units |
TSS |
2620 |
2517 |
TDS |
2124 ± 512 |
1660 ± 144 |
Conductivity |
4541 ± 35 μS cm−1 |
3531 ± 35 μS cm−1 |
Alkalinity |
2983 ± 222 mg L−1 CaCO3 |
975 ± 29 mg L−1 CaCO3 |
BOD5 |
670 ± 15 |
77 ± 21 |
COD |
1055 ± 21 |
815 ± 35 |
TOC |
866 ± 1 |
105 ± 15 |
Na+ |
299 ± 3 |
137 ± 1 |
Ca2+ |
457 ± 10 |
334 ± 3 |
Si4+ |
33 ± 1 |
26 ± 1 |
K+ |
224 ± 1 |
59 ± 1 |
Mg2+ |
198 ± 1 |
170 ± 1 |
Fe3+ |
4 ± 1 |
7 ± 1 |
Cl− |
184 ± 6 |
160 ± 3 |
NO3−–N |
3.0 ± 0.05 |
0.25 ± 0.50 |
SO42− |
445 ± 7 |
435 ± 40 |
PO43− |
1.4 ± 0.1 |
0.2 ± 0.1 |
MoS2 membranes: physical and chemical changes upon weathering in leachate
Characterization of the MoS2 membranes exposed to ML and YL over 8 weeks is shown in Fig. 1. Raman spectra of the samples (Fig. 1a) show distinct MoS2 peaks, corresponding to the in-plane vibration (E12g mode) of the two atoms (i.e., with respect to the Mo atom at ∼379 cm−1) and the out-of-plane vibration (A1g mode) of the S atoms (at ∼405 cm−1). The FTIR spectra of the samples over time (Fig. 1b) show no appreciable changes to the functional groups on the membranes. The FTIR spectra of control membranes (containing no MoS2) are shown in Fig. S1.† Compiled SEM images in Fig. 1c show the membrane surface over the exposure period. While the micron-scale morphology remains relatively unchanged, some new features can be observed on the membrane surfaces during aging. The observed features are likely attached microbial fragments and/or organic impurities or colloids, based on the oblong feature on the MoS2-membranes that are aged over 1 week in ML. Similarly, regular crystalline morphology of salts appears in the 4- and 8 week samples, when observed at lower magnification (Fig. S2†). The concentrations of organic impurities associated with the membrane surface are likely to be low because they were not detected by multiple FTIR scans. Overall, at these tested conditions, the membrane surfaces only accumulate biomaterials and/or colloids at the surface, which do not appear to chemically or structurally affect the MoS2 embedded within the membranes.
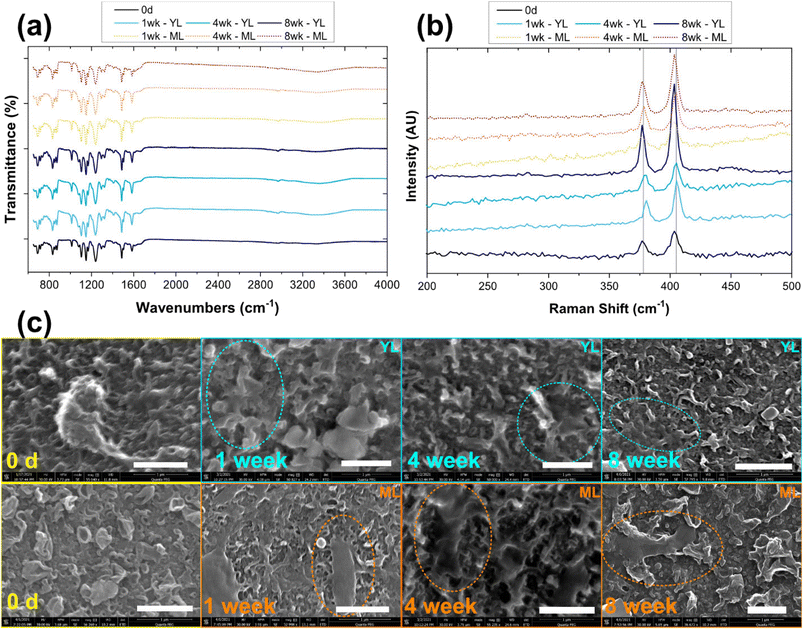 |
| Fig. 1 Physicochemical characterization of MoS2-enabled membranes upon exposure to YL and ML over a period of 8 weeks. Panels show (a) FTIR spectra of the membranes, (b) Raman spectra of the membranes, and (c) SEM images of the membrane surface upon exposure to YL and ML. Circles indicate attachment of organic solids that were not observed in the pristine membrane images. Scale bars shown in the image are 1 μm. | |
Several published papers have documented the release of embedded NMs from nanocomposite polymeric matrices under a range of degradation conditions.19,48–50 Gallagher et al. studied the environmental exposure of CdSe- and CdSe/ZnS-embedded polymer under ultraviolet (UV-C) irradiation. The resulting loss in NM signals and detection of polymeric fragments in the aqueous media indicated the release of NMs in ionic form and those associated with the released polymer fragments.48 Similarly, Pillai et al. observed the release of quantum dots in ionic and polymer-embedded forms when the nanocomposite materials were exposed to water or 3% acetic acid at 75 °C for 15 days.49 Using laser scanning confocal microscopy (LSCM) to track the luminescence of the quantum dots, that study also reported NM release from the interior of the nanocomposite into the surrounding matrix. If stronger degradation conditions such as exposure to ultraviolet light, longer exposure, or higher temperature, are applied, MoS2 membranes might undergo more severe degradation and NM release as compared what has been observed in our study.
Assessment of MoS2 or metal ion release from the membranes
The concentrations of dissolved molybdenum (Mo) and total Mo (particulate plus dissolved Mo) released from MoS2-enabled membranes are shown in Fig. 2a and b. Additionally, ICP-OES data for dissolved and total Mo released from control membranes (containing no Mo) are compiled in ESI† Fig. S3. In YL-only or ML-only (control) samples, the estimated concentration of total Mo is less than the detection limit of ICP-OES for Mo (4 μg L−1). Over time, the degradation of the membranes in ML and YL result in a release of Mo species into the leachate. The dissolved Mo in the samples containing MoS2 membranes in both ML and YL (Fig. 2a) is approximately 40% of the total Mo released, indicating that the primary form of release is likely to be particulate. A sustained release of Mo (dissolved and total) over time is evident in YL and ML (Fig. 2b), as the concentration of Mo increases from approximately 0.4–0.5 μg Mo per L per mg of membrane to approximately 0.6 μg Mo per L per mg of membrane.
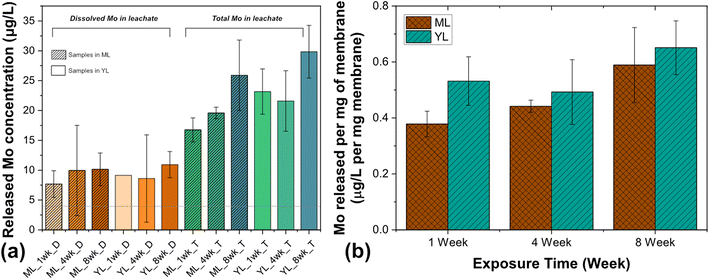 |
| Fig. 2 (a) Concentration of dissolved and total molybdenum (Mo) released from MoS2-enabled membranes, and (b) concentration of total Mo released (in μg L−1) per mg of MoS2-enabled membrane exposed to ML and YL over an exposure period of 8 weeks, measured by ICP-OES. Error bars represent the standard deviation of up to 3 replicate measurements, and the limit of detection is shown with a dashed line in (a). | |
Metal ion release from other metallic NMs exposed to landfill leachate has been reported in the literature.51 The composition of organic matter (i.e., acetic and humic acids) in the leachate was linked to the stability of the nano-ZnO in the presence of heavy metals. Li et al. investigated the fate of nano-ZnO in simulated landfill leachate as a function of its complicated interactions with coexisting heavy metal ions, such as Cu(II), Cr(III), and Cr(VI). In fresh leachate, Cu(II) ions aided the dissolution of ZnO in young leachate; however, such dissolution was suppressed in mature leachate due to the association of ZnO with humic acids.51 In another study, when silver nanoparticles (AgNPs) were exposed to middle-aged and mature landfill leachates for 56 days, the increase in aqueous silver concentration over time was attributed to the increased dispersibility and dissolution of the AgNPs due to their association with the organic fractions of the leachates. The dissolved Ag+ concentration was predicted to be much lower (<1%) than the dissolved Ag associated with sulfide and ammonia components in the leachates.52 This study also observed increased concentrations of NMs in larger size fractions isolated from the leachate, indicating that the NMs agglomerated, likely due to high ionic strength in the leachate. In the case of MoS2 release into leachates, a detailed study is necessary to identify whether high ionic strength of the medium also can cause precipitation and agglomeration of dissolved Mo.
MoS2 devices: physical and chemical changes upon weathering in leachates
Characterization of the MoS2-enabled devices exposed to ML and YL over 0–6 weeks is shown in Fig. 3. For the samples exposed to YL (Fig. 3b), some minor physical fragmentation of the flakes is observed. Fig. 3c shows almost full coverage of the substrate surface with colloids and solid impurities from the leachate, nearly obscuring any MoS2 flake or alignment markers from observation. The PL and Raman spectra displayed in Fig. 3d and e are captured from the green laser spot in Fig. 3b; the presence of MoS2 is confirmed because typical Raman (379 cm−1 & 405 cm−1) and PL (1.85 eV) spectral peaks for MoS2 are found.
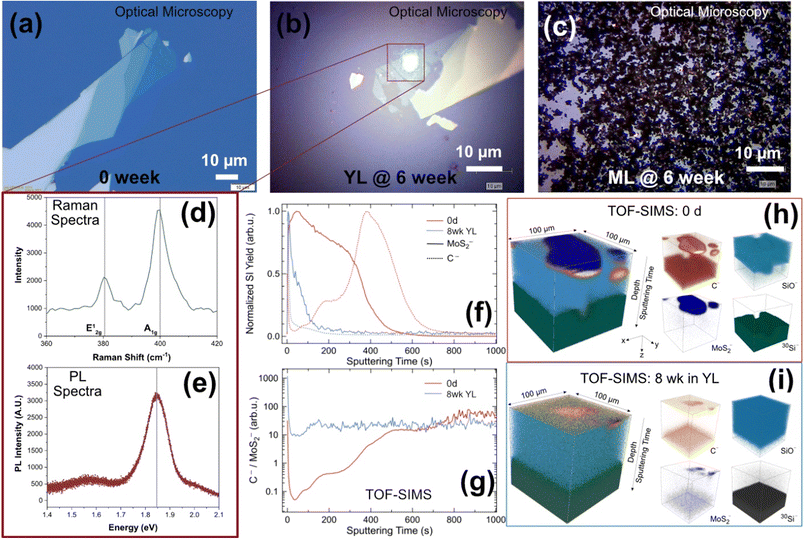 |
| Fig. 3 Physicochemical characterization of MoS2-enabled devices upon exposure to YL and ML over time. Panels show: optical microscopy image of MoS2 devices aged for (a) 0 weeks (pristine device), (b) 6 weeks in YL, and (c) 6 weeks in ML; (d) Raman spectra, and (e) PL spectra of MoS2 corresponding to the green laser spot in (b); (f) TOF-SIMS depth profiles normalized to their maxima of MoS2− (solid lines) and C− (dashed lines) from MoS2 devices aged 0 weeks and 8 weeks in YL. Sputtering time represents depth; (g) C− : MoS2− TOF-SIMS depth profiles ratio on the MoS2-device at 0 d vs. 8 weeks in YL; 3D rendering of MoS2− and C− TOF-SIMS depth profiles for MoS2-devices aged for (h) 0 weeks and (i) 8 weeks in YL. | |
The onset of fragmentation of MoS2 flakes on the devices is indicated in Fig. 3c (where the sample was aged to 6 weeks), and for the samples aged for 1–2 weeks (not pictured), where monolayers are not located during post-exposure characterization. These flakes likely have disintegrated directly into the leachate matrix. Alternatively, the flakes could have been dislodged during a processing step (drops of DI water added and dried with nitrogen gas) that was conducted to remove deposited solids from the device surface after exposure. Fig. 3f compares the TOF-SIMS depth profiles of MoS2− and carbon, normalized to their maxima on a pristine MoS2 device and on a device exposed for 8 weeks to YL (analysis conditions: Cs+ sputtering ion beam, Bi+ analysis ion beam, sputtering area: 300 μm × 300 μm, analysis area: 100 μm × 100 μm). These profiles indicate the depth localization (stratification) of various component layers at the surface. To establish a relative comparison of C to MoS2 amounts between the pristine and aged devices, C−
:
MoS2− secondary ion yield ratio has been analyzed as a function of depth (Fig. 3g). This ratio accounts for analysis area and sputtering condition variations between the samples. Near the device surface (corresponding to low sputtering times), the ratio is approximately 200 times larger for the aged sample as compared to the pristine MoS2-device, indicating that a large amount of carbon has associated with the device surface during exposure to YL. This is apparent from Fig. 3i, where carbon is observed in close proximity to Mo on the device surface, as compared to the carbon signature that mainly associates with the Si substrate under the MoS2 flakes (Fig. 3h). The 3D rendering of these elemental distributions (Fig. 3h and i) indicates that the presence of MoS2 decreased during the exposure of the devices to the leachates over the 8 weeks period. Since these flakes are micron-scale and only one device is exposed per sample tube for this study, detection of Mo in the leachate sample is not expected; therefore, leachate was not characterized with ICP-OES. Future studies could consider the exposure of a larger device, or multiple devices in leachates for more accurate assessment of particulate and dissolved Mo release from such device surfaces.
Impact of colloidal MoS2 on leachate microbial community
The bacterial communities in the leachates, with and without MoS2 exposure over a period of 8 weeks, are shown in Fig. 4. The Archaeal community profiles for the same conditions are presented in ESI† Fig. S4. Distinct communities are observed in YL and ML at the initial time point, as indicated by the PCoA (Fig. 5) plot, showing clustering of the ML and YL samples in different quadrants. Pair-wise similarity between samples using UniFrac distances (α = 0.5) also is presented in ESI† Table S1, where ‘0’ indicates identical samples and ‘1’ indicates that no branches in the phylogenetic tree are shared by the samples. At the phylum level (Fig. 4), the bacterial communities in ML and YL are dominated by Proteobacteria (relative abundance of 46–82%), which includes a wide range of Gram-negative bacteria. Overall, the high abundance of Firmicutes, Proteobacteria, and Bacteroidetes phyla agree with several published studies, which report microorganisms found in typical anaerobic environments pertinent to degradation processes of macromolecules in landfills.27,53,54 As an initial check on MoS2 toxicity, the Gram-negative soil bacterium Pseudomonas putida strain F1 was exposed to high concentrations of MoS2, the cell density was reduced by approximately 36% (Fig. S5†).
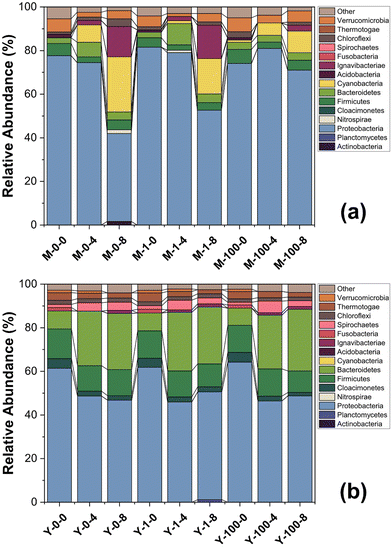 |
| Fig. 4 Relative abundance of Bacteria at the phylum level in (a) ML, and (b) YL in the presence of MoS2. Only phyla comprising 1% or greater of the community are shown. Sample names are read as: Y: young, M: mature, first number: MoS2 concentration in mg L−1, second number: time in weeks. | |
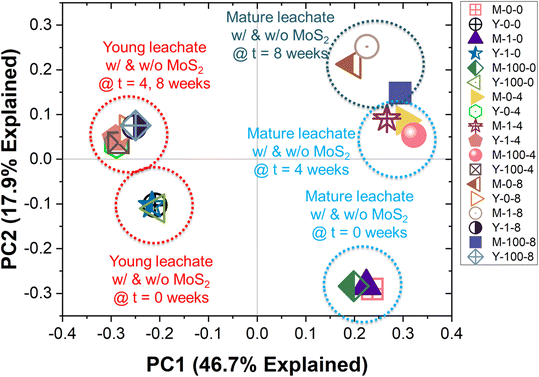 |
| Fig. 5 PCoA plot based on UniFrac distances (α = 0.5) of ML and YL samples over time, containing 0, 1, and 100 mg L−1 MoS2. The first two principal coordinates account for approximately 64.6% of the variation in the microbial community data. Sample names are read as: Y: young, M: mature, first number: MoS2 concentration in mg L−1, second number: time in weeks. | |
When qualitatively examining the microbial community of ML at a single MoS2 concentration (Fig. 4a), shifts in the predominant phyla are observed over time. The decrease in Proteobacteria and increase in Cyanobacteria relative abundance over time is suppressed in the presence of increasing MoS2 concentration. The temporal increase in Cyanobacteria, a photosynthetic phylum, is confined to the ML samples, where its relatively higher optical clarity as compared to that of YL might have allowed light intrusion during sample removal/adjustment from the shaker. The smaller temporal increase in Cyanobacteria in ML samples containing 100 mg L−1 of MoS2, compared to the other MoS2 levels in ML, supports this conclusion—because high concentrations of MoS2 produce a dark ML suspension, likely suppressing phototrophic growth. Meanwhile, in the YL microbial community (Fig. 4b), the relative abundance of populations appears to be relatively unperturbed over time when exposed to MoS2.
The PCoA plot (Fig. 5) indicates that the samples with different MoS2 concentrations at the same time point are clustered together. For instance, the data points corresponding to 0, 1, and 100 mg L−1 MoS2 in YL at 4 weeks cluster in the fourth quadrant. This is supported by the UniFrac distance matrix (Table S1†), where small UniFrac distances are calculated between samples at different MoS2 concentrations (0, 1, 100 mg L−1 MoS2) at the same time point (Adonis, p > 0.5 (YL) and p > 0.2 (ML)), while substantial UniFrac distances are calculated for a sample with the same MoS2 concentration over time (Adonis, p < 0.01 (YL) and p < 0.05 (ML)). That is, the microbial communities change significantly from time 0 to 4 weeks (pairwiseAdonis, p <0.1 (YL) and p = 0.1 (ML)); however, the shift in the microbial communities from the 4th week to 8th week is not significant (pairwiseAdonis, p > 0.1 (YL) and p > 0.8 (ML)).
Conclusions and environmental implications
Research on NM toxicity has evolved from studying the interaction of ENM in simple aquatic systems and short timescales to more realistic and complex matrices, as characterization techniques and resolutions improve. The study of medium- and long-term effects of ENMs (or close approximations of typical product matrices containing ENMs) in environmental sinks at environmentally relevant concentrations can provide insight into the fate and transport of these materials. Under the tested conditions, this study indicates that membranes and devices with embedded MoS2 do not leach ENM from the matrices and thus may not impart significant deleterious impacts to the leachate chemistry or microbiome. However, it is to be noted that the electronegativity of the chalcogenide ligands (i.e., S, Se, or Te) varies widely (S: 2.58, Se: 2.55, Te: 2.1),55 which likely will influence metal release from the TMDs. That is, the more electronegative the ligand (e.g., S as compared to Se and Te), the stronger it will bind the metal. Furthermore, the layer thickness of 2D ENMs affects the electronic properties and influences the number of metal atoms available for release. It also can affect the attractive forces between the sheets, which in turn can influence NM stability in water. Thus, the apparent benign nature of MoS2-modified membranes and transistors should not be taken as reflective of all TMD-enabled devices. Other TMD-enabled devices, particularly those with ligands having lower electronegativity as compared to S or those embedded with mono-layer TMDs, should be assessed carefully to evaluate their safety.
Because landfills are designed to receive wastes for an extended period of time, high concentrations of MoS2 and other TMDs are likely to accrue in landfill leachate. Unlike other environmental systems, high NM concentrations can be relevant for landfills. Although substantial changes in microbial community structure did not occur due to MoS2 exposures up to 100 mg L−1, the effect of longer exposure times at higher concentrations of the 2D NMs should be examined in future studies. Leachate pH may vary widely and thus can affect NM dissolution, surface charge, and thus NM fate. Leachates with a wide range of pH may be used in the future to assess NM fate in the leachate environment.
A key novelty of this work lies in the careful experiments conducted in a complex landfill environment. This is also one of the first studies that utilized both colloidal NMs and water treatment membranes and devices modified with MoS2 to evaluate the safety of NM-enabled devices. The methodology developed here can guide the nano-EHS community toward assessing NM-enabled device safety in complex environmental systems. It can be concluded that under the conditions examined in this study, the MoS2-enabled electronic devices and membranes do not present significant deleterious impacts to the landfill environment during their end-of-life disposal; notably, the landfill microbiome did not change appreciably in the face of high MoS2 concentrations (100 mg L−1) as compared to the absence of MoS2.
Abbreviations
MoS2 | Molybdenum disulfide |
YL | Young leachate |
ML | Mature leachate |
Author contributions
The manuscript was written through contributions of all authors. All authors have given approval to the final version of the manuscript.
Conflicts of interest
There are no conflicts to declare.
Acknowledgements
IVS was partially supported by the Environmental Research and Education Foundation (EREF) Scholarship and by funding from the U.S. Environmental Protection Agency (00D68518 to MJK and NBS). The views expressed herein are those of the authors and do not necessarily reflect the views or policies of U.S. Environmental Protection Agency nor EREF; therefore, no official endorsement should be inferred. Any mention of trade names or commercial products does not constitute endorsement or recommendation for use. The authors thank the instrument managers at the Texas Materials Institute for their assistance with material characterization.
References
- M. M. Falinski, R. S. Turley, J. Kidd, A. W. Lounsbury, M. Lanzarini-Lopes, A. Backhaus, H. E. Rudel, M. K. M. Lane, C. L. Fausey, A. C. Barrios, J. E. Loyo-Rosales, F. Perreault, W. S. Walker, L. B. Stadler, M. Elimelech, J. L. Gardea-Torresdey, P. Westerhoff and J. B. Zimmerman, Doing Nano-Enabled Water Treatment Right: Sustainability Considerations from Design and Research through Development and Implementation, Environ. Sci.: Nano, 2020, 7(11), 3255–3278, 10.1039/d0en00584c.
- N. C. Mueller, J. Buha, J. Wang, A. Ulrich and B. Nowack, Modeling the Flows of Engineered Nanomaterials during Waste Handling, Environ. Sci.: Process. Impacts, 2013, 15(1), 251–259, 10.1039/c2em30761h.
- A. A. Keller and A. Lazareva, Predicted Releases of Engineered Nanomaterials: From Global to Regional to Local, Environ. Sci. Technol. Lett., 2013, 1(1), 65–70, DOI:10.1021/ez400106t.
- A. A. Keller, S. McFerran, A. Lazareva and S. Suh, Global Life Cycle Releases of Engineered Nanomaterials, J. Nanopart. Res., 2013, 15 DOI:10.1007/s11051-013-1692-4.
- N. C. Mueller, J. Buha, J. Wang, A. Ulrich and B. Nowack, Modeling the Flows of Engineered Nanomaterials during Waste Handling, Environ. Sci.: Process. Impacts, 2013, 15(1), 251–259, 10.1039/c2em30761h.
- A. Caballero-Guzman, T. Sun and B. Nowack, Flows of Engineered Nanomaterials through the Recycling Process in Switzerland, Waste Manage., 2015, 36, 33–43, DOI:10.1016/j.wasman.2014.11.006.
- M. M. Falinski, D. L. Plata, S. S. Chopra, T. L. Theis, L. M. Gilbertson and J. B. Zimmerman, A Framework for Sustainable Nanomaterial Selection and Design Based on Performance, Hazard, and Economic Considerations, Nat. Nanotechnol., 2018, 13(8), 708–714, DOI:10.1038/s41565-018-0120-4.
- D. L. Plata and N. Z. Janković, Achieving Sustainable Nanomaterial Design Though Strategic Cultivation of Big Data, Nat. Nanotechnol., 2021, 16(6), 612–614, DOI:10.1038/s41565-021-00902-7.
- M. Auffan, M. Tella, C. Santaella, L. Brousset, C. Paillès, M. Barakat, B. Espinasse, E. Artells, J. Issartel, A. Masion, J. Rose, M. R. Wiesner, W. Achouak, A. Thiéry and J. Y. Bottero, An Adaptable Mesocosm Platform for Performing Integrated Assessments of Nanomaterial Risk in Complex Environmental Systems, Sci. Rep., 2014, 4, 11–14, DOI:10.1038/srep05608.
- N. K. Geitner, J. L. Cooper, A. Avellan, B. T. Castellon, B. G. Perrotta, N. Bossa, M. Simonin, S. M. Anderson, S. Inoue, M. F. Hochella, C. J. Richardson, E. S. Bernhardt, G. V. Lowry, P. L. Ferguson, C. W. Matson, R. S. King, J. M. Unrine, M. R. Wiesner and H. Hsu-Kim, Size-Based Differential Transport, Uptake, and Mass Distribution of Ceria (CeO2) Nanoparticles in Wetland Mesocosms, Environ. Sci. Technol., 2018, 52(17), 9768–9776, DOI:10.1021/acs.est.8b02040.
- E. S. Money, L. E. Barton, J. Dawson, K. H. Reckhow and M. R. Wiesner, Validation and Sensitivity of the FINE Bayesian Network for Forecasting Aquatic Exposure to Nano-Silver, Sci. Total Environ., 2014, 473–474, 685–691, DOI:10.1016/j.scitotenv.2013.12.100.
- A. Avellan, M. Simonin, E. McGivney, N. Bossa, E. Spielman-Sun, J. D. Rocca, E. S. Bernhardt, N. K. Geitner, J. M. Unrine, M. R. Wiesner and G. V. Lowry, Gold Nanoparticle Biodissolution by a Freshwater Macrophyte and Its Associated Microbiome, Nat. Nanotechnol., 2018, 13(11), 1072–1077, DOI:10.1038/s41565-018-0231-y.
- A. Avellan, M. Simonin, S. M. Anderson, N. K. Geitner, N. Bossa, E. Spielman-Sun, E. S. Bernhardt, B. T. Castellon, B. P. Colman, J. L. Cooper, M. Ho, M. F. Hochella, H. Hsu-Kim, S. Inoue, R. S. King, S. Laughton, C. W. Matson, B. G. Perrotta, C. J. Richardson, J. M. Unrine, M. R. Wiesner and G. V. Lowry, Differential Reactivity of Copper- And Gold-Based Nanomaterials Controls Their Seasonal Biogeochemical Cycling and Fate in a Freshwater Wetland Mesocosm, Environ. Sci. Technol., 2020, 54(3), 1533–1544, DOI:10.1021/acs.est.9b05097.
- B. P. Espinasse, N. K. Geitner, A. Schierz, M. Therezien, C. J. Richardson, G. V. Lowry, L. Ferguson and M. R. Wiesner, Comparative Persistence of Engineered Nanoparticles in a Complex Aquatic Ecosystem, Environ. Sci. Technol., 2018, 52(7), 4072–4078, DOI:10.1021/acs.est.7b06142.
- M. Nassar, M. Auffan, M. Auffan, C. Santaella, A. Masion, J. Rose and J. Rose, Multivariate Analysis of the Exposure and Hazard of Ceria Nanomaterials in Indoor Aquatic Mesocosms, Environ. Sci.: Nano, 2020, 7(6), 1661–1669, 10.1039/c9en01439j.
- P. Kjeldsen, M. A. Barlaz, A. P. Rooker, A. Baun, A. Ledin and T. H. Christensen, Present and Long-Term Composition of MSW Landfill Leachate: A Review, Crit. Rev. Environ. Sci. Technol., 2002, 32(4), 297–336, DOI:10.1080/10643380290813462.
- J. Harmsen, Identification of Organic Compounds in Leachate from a Waste Tip, Water Res., 1983, 17(6), 699–705, DOI:10.1016/0043-1354(83)90239-7.
- J. Artiola-Fortuny and W. H. Fuller, Humic Substances in Landfill Leachates: I. Humic Acid Extraction and Identification, J. Environ. Qual., 1982, 11(4), 663–669, DOI:10.2134/jeq1982.00472425001100040021x.
- F. Part, C. Zaba, O. Bixner, C. Zafiu, S. Lenz, L. Martetschläger, S. Hann, M. Huber-Humer and E. K. Ehmoser, Mobility and Fate of Ligand Stabilized Semiconductor Nanoparticles in Landfill Leachates, J. Hazard. Mater., 2020, 394, 122477, DOI:10.1016/j.jhazmat.2020.122477.
- P. Nain and A. Kumar, Metal Dissolution from End-of-Life Solar Photovoltaics in Real Landfill Leachate versus Synthetic Solutions: One-Year Study, Waste Manage., 2020, 114, 351–361, DOI:10.1016/j.wasman.2020.07.004.
- K. F. Mak, C. Lee, J. Hone, J. Shan and T. F. Heinz, Atomically Thin MoS2: A New Direct-Gap Semiconductor, Phys. Rev. Lett., 2010, 105(13), 2–5, DOI:10.1103/PhysRevLett.105.136805.
- A. Castellanos-Gomez, R. van Leeuwen, M. Buscema, H. S. J. van der Zant, G. A. Steele and W. J. Venstra, Single-Layer MoS2 Mechanical Resonators, Adv. Mater., 2013, 25(46), 6719–6723, DOI:10.1002/adma.201303569.
- Z. Wang, Q. Tu, S. Zheng, J. J. Urban, S. Li and B. Mi, Understanding the Aqueous Stability and Filtration Capability of MoS2 Membranes, Nano Lett., 2017, 17(12), 7289–7298, DOI:10.1021/acs.nanolett.7b02804.
- K. Ai, C. Ruan, M. Shen and L. Lu, MoS2 Nanosheets with Widened Interlayer Spacing for High-Efficiency Removal of Mercury in Aquatic Systems, Adv. Funct. Mater., 2016, 26(30), 5542–5549 CrossRef CAS.
- A. E. Gash, A. L. Spain, L. M. Dysleski, C. J. Flaschenriem, A. Kalaveshi, P. K. Dorhout and S. H. Strauss, Efficient Recovery of Elemental Mercury
from Hg(II)-Contaminated Aqueous Media Using a Redox-Recyclable Ion-Exchange Material, Environ. Sci. Technol., 1998, 32(7), 1007–1012, DOI:10.1021/es970804n.
- Z. Wang, A. Von Dem Bussche, Y. Qiu, T. M. Valentin, K. Gion, A. B. Kane and R. H. Hurt, Chemical Dissolution Pathways of MoS2 Nanosheets in Biological and Environmental Media, Environ. Sci. Technol., 2016, 50(13), 7208–7217, DOI:10.1021/acs.est.6b01881.
- L. Song, Y. Wang, W. Tang and Y. Lei, Bacterial Community Diversity in Municipal Waste Landfill Sites, Appl. Microbiol. Biotechnol., 2015, 99(18), 7745–7756, DOI:10.1007/s00253-015-6633-y.
- Y. Yang, S. Gajaraj, J. D. Wall and Z. Hu, A Comparison of Nanosilver and Silver Ion Effects on Bioreactor Landfill Operations and Methanogenic Population Dynamics, Water Res., 2013, 47, 3422–3430, DOI:10.1016/j.watres.2013.03.040.
- E. L. K. Chng, Z. Sofer and M. Pumera, MoS2 Exhibits Stronger Toxicity with Increased Exfoliation, Nanoscale, 2014, 6(23), 14412–14418, 10.1039/c4nr04907a.
- O. Akhavan and E. Ghaderi, Toxicity of Graphene and Graphene Oxide Nanowalls against Bacteria, ACS Nano, 2010, 4(10), 5731–5736, DOI:10.1021/nn101390x.
- W. Z. Teo, E. L. K. Chng, Z. Sofer and M. Pumera, Cytotoxicity of Exfoliated Transition-Metal Dichalcogenides (MoS 2, WS2, and WSe2) Is Lower than That of Graphene and Its Analogues, Chem. – Eur. J., 2014, 20(31), 9627–9632, DOI:10.1002/chem.201402680.
- J. H. Appel, D. O. Li, J. D. Podlevsky, A. Debnath, A. A. Green, Q. H. Wang and J. Chae, Low Cytotoxicity and Genotoxicity of Two-Dimensional MoS2 and WS2, ACS Biomater. Sci. Eng., 2016, 2(3), 361–367, DOI:10.1021/acsbiomaterials.5b00467.
- S. Liu, Z. Shen, B. Wu, Y. Yu, H. Hou, X. X. Zhang and H. Q. Ren, Cytotoxicity and Efflux Pump Inhibition Induced by Molybdenum Disulfide and Boron Nitride Nanomaterials with Sheetlike Structure, Environ. Sci. Technol., 2017, 51(18), 10834–10842, DOI:10.1021/acs.est.7b02463.
-
B. A. Chambers, A Molecular Biological Model Describing Silver Nanoparticle Mechanism of Toxicity and Associated Antibiotic Resistance, Dissertation, The University of Texas at Austin, 2018 Search PubMed.
- E. Barré, J. A. C. Incorvia, S. H. Kim, C. J. McClellan, E. Pop, H. S. P. Wong and T. F. Heinz, Spatial Separation of Carrier Spin by the Valley Hall Effect in Monolayer WSe2Transistors, Nano Lett., 2019, 19(2), 770–774, DOI:10.1021/acs.nanolett.8b03838.
- A. Inurria, P. Cay-Durgun, D. Rice, H. Zhang, D. K. Seo, M. L. Lind and F. Perreault, Polyamide Thin-Film Nanocomposite Membranes with Graphene Oxide Nanosheets: Balancing Membrane Performance and Fouling Propensity, Desalination, 2019, 451, 139–147, DOI:10.1016/j.desal.2018.07.004.
- S. C. Bolyard, D. R. Reinhart and S. Santra, Behavior of Engineered Nanoparticles in Landfill Leachate, Environ. Sci. Technol., 2013, 47(15), 8114–8122, DOI:10.1021/es305175e.
- J. Shi, Y. Su, Z. Zhang and B. Xie, How Do Zinc Oxide and Zero Valent Iron Nanoparticles Impact the Occurrence of Antibiotic Resistance Genes in Landfill Leachate?, Environ. Sci.: Nano, 2019, 6, 2141–2151, 10.1039/c9en00068b.
- L. S. Rowles, A. I. Hossain, S. Aggarwal, M. J. Kirisits and N. B. Saleh, Water Quality and Associated Microbial Ecology in Selected Alaska Native Communities: Challenges in off-the-Grid Water Supplies, Sci. Total Environ., 2020, 711 DOI:10.1016/j.scitotenv.2019.134450.
- J. R. Cole, Q. Wang, J. A. Fish, B. Chai, D. M. McGarrell, Y. Sun, C. T. Brown, A. Porras-Alfaro, C. R. Kuske and J. M. Tiedje, Ribosomal Database Project: Data and Tools for High Throughput RRNA
Analysis, Nucleic Acids Res., 2014, 42(D1), 633–642, DOI:10.1093/nar/gkt1244.
- L. S. Rowles, A. I. Hossain, I. Ramirez, N. J. Durst, P. M. Ward, M. J. Kirisits, I. Araiza, D. F. Lawler and N. B. Saleh, Seasonal Contamination of Well-Water in Flood-Prone Colonias and Other Unincorporated U.S. Communities, Sci. Total Environ., 2020, 740, 140111, DOI:10.1016/j.scitotenv.2020.140111.
- P. J. McMurdie and S. Holmes, Phyloseq: An R Package for Reproducible Interactive Analysis and Graphics of Microbiome Census Data, PLoS One, 2013, 8(4), e61217, DOI:10.1371/journal.pone.0061217.
- E. Paradis and K. Schliep, Ape 5.0: An Environment for Modern Phylogenetics and Evolutionary Analyses in R, Bioinformatics, 2019, 35, 526–528, DOI:10.1093/bioinformatics/bty633.
- J. Chen, K. Bittinger, E. S. Charlson, C. Hoffmann, J. Lewis, G. D. Wu, R. G. Collman, F. D. Bushman and H. Li, Associating Microbiome Composition with Environmental Covariates Using Generalized UniFrac Distances, Bioinformatics, 2012, 28(16), 2106–2113, DOI:10.1093/bioinformatics/bts342.
- C. Lozupone and R. Knight, UniFrac: A New Phylogenetic Method for Comparing Microbial Communities, Appl. Environ. Microbiol., 2005, 71(12), 8228–8235, DOI:10.1128/AEM.71.12.8228-8235.2005.
-
J. Oksanen, F. G. Blanchet, R. Kindt, P. Legendre, P. R. Minchin, R. B. O'Hara, G. L. Simpson, P. Solymos, M. H. H. Stevens and H. Wagner, Vegan: Community Ecology Package, R Package Version 2.5-7, 2014 Search PubMed.
-
P. Martinez Arbizu, PairwiseAdonis: Pairwise Multilevel Comparison Using Adonis, 2020 Search PubMed.
- M. J. Gallagher, J. T. Buchman, T. A. Qiu, B. Zhi, T. Y. Lyons, K. M. Landy, Z. Rosenzweig, C. L. Haynes and D. H. Fairbrother, Release, Detection and Toxicity of Fragments Generated during Artificial Accelerated Weathering of CdSe/ZnS and CdSe Quantum Dot Polymer Composites, Environ. Sci.: Nano, 2018, 5(7), 1694–1710, 10.1039/c8en00249e.
- K. V. Pillai, P. J. Gray, C. C. Tien, R. Bleher, L. P. Sung and T. V. Duncan, Environmental Release of Core-Shell Semiconductor Nanocrystals from Free-Standing Polymer Nanocomposite Films, Environ. Sci.: Nano, 2016, 3(3), 657–669, 10.1039/c6en00064a.
- R. S. Lankone, J. Wang, J. F. Ranville and D. H. Fairbrother, Photodegradation of Polymer-CNT Nanocomposites: Effect of CNT Loading and CNT Release Characteristics, Environ. Sci.: Nano, 2017, 4(4), 967–982, 10.1039/c6en00669h.
- X. Li, W. Ding, S. Tan and X. Zeng, Stability of Nano-ZnO in Simulated Landfill Leachate Containing Heavy Metal Ions, Ecotoxicol. Environ. Saf., 2020, 198, 110641, DOI:10.1016/j.ecoenv.2020.110641.
- S. C. Bolyard, D. R. Reinhart and S. Santra, Behavior of Engineered Nanoparticles in Landfill Leachate, Environ. Sci. Technol., 2013, 47(15), 8114–8122, DOI:10.1021/es305175e.
- S. J. Liu, B. D. Xi, Z. P. Qiu, X. S. He, H. Zhang, Q. L. Dang, X. Y. Zhao and D. Li, Succession and diversity of microbial communities in landfills with depths and ages and its association with dissolved organic matter and heavy metals, Sci. Total Environ., 2019, 651, 909–916, DOI:10.1016/j.scitotenv.2018.09.267.
- L. Song, Y. Wang, H. Zhao and D. T. Long, Composition of Bacterial and Archaeal Communities during Landfill Refuse Decomposition Processes, Microbiol. Res., 2015, 181, 105–111, DOI:10.1016/j.micres.2015.04.009.
- L. Pauling, The Nature of the Chemical Bond. IV. The Energy of Single Bonds and the Relative Electronegativity of Atoms, J. Am. Chem. Soc., 1932, 54(9), 3570–3582 CrossRef CAS.
Footnotes |
† Electronic supplementary information (ESI) available: R source code to process microbial data, aging of membranes: additional data, MoS2 tolerance by pure culture strain Pseudomonas putida, microbial DNA analyses, archaeal community profiles. See DOI: https://doi.org/10.1039/d2en00707j |
‡ Current Affiliation: Semiconductor Process Engineer, Micron Technology Inc., Boise, ID, USA |
|
This journal is © The Royal Society of Chemistry 2023 |
Click here to see how this site uses Cookies. View our privacy policy here.