ZnIn2S4 with oxygen atom doping and surface sulfur vacancies for overall water splitting under visible light irradiation†
Received
17th October 2022
, Accepted 11th November 2022
First published on 12th November 2022
Abstract
Previous reports have found that surface sulfur vacancies can improve the hydrogen production performance of ZnIn2S4, and oxygen atom doping can improve its water oxidation ability. However, the simultaneous introduction of these two factors to achieve photocatalytic overall water splitting under visible-light irradiation has not been reported. In this work, we introduce surface sulfur vacancies and oxygen atom doping simultaneously via calcination of the ZnIn2S4 obtained by a hydrothermal method under an air atmosphere. Their synergistic effect on the photocatalytic overall water splitting activity is investigated in detail by controlling the calcination temperature and calcination time. Both surface sulfur vacancies and oxygen atom doping can inhibit carrier recombination, and oxygen atom doping can increase the photogenerated carrier concentration. Surface sulfur vacancies and oxygen atom doping can increase the photocatalytic rate 2–3 times, respectively, confirming that the two are equally important for the improvement of photocatalytic activity. More importantly, the synergistic effect of sulfur vacancies and oxygen atom doping results in a solar-to-hydrogen (STH) efficiency of ∼0.035% (the highest efficiency reported so far for single-phase ZnIn2S4) and a stable photocatalytic overall water splitting performance.
1. Introduction
Photocatalytic overall water splitting for hydrogen production is considered as one of the effective strategies to solve energy shortage and environmental pollution.1–4 In order to realize the commercial value of photocatalytic hydrogen production, the STH efficiency should reach 10%.5,6 According to the relationship between STH efficiency and the wavelength of sunlight, the absorption edge of the photocatalyst should be above 500 nm to achieve this goal.5,7 Therefore, photocatalysts with a wide visible light absorption range are still a research hotspot.
At present, the photocatalysts that have an absorption edge above 500 nm and an overall water splitting ability are mainly oxysulfides (such as Y2Ti2O5S2),8,9 oxynitrides (such as CaTaO2N, LaMg1/3Ta2/3O2N, and Ta3N5),10–12 and sulfides (such as ZnIn2S4, CdS).2,13–15 Among them, ZnIn2S4 has attracted much attention because of its simple preparation method and environmentally friendliness.16–18 Limited by its rapid carrier recombination and severe photocorrosion, the water splitting efficiency of ZnIn2S4 is low. A number of strategies have been explored to improve its efficiency, including doping,19 heterojunction construction,18 and morphology regulation.2 Compared with other strategies, the doping strategy with simple and effective advantages has attracted more attention.
ZnIn2S4 with metal doping (such as Ag and Zn)2,19 uses metal impurities as active sites for oxygen production, which effectively inhibit photocorrosion. Meanwhile, metal impurities themselves become electron–hole recombination centers, resulting in low overall water splitting activity. Oxygen atom doping as a kind of non-metallic doping can reduce the oxygen evolution reaction (OER) overpotential to enhance photocorrosion resistance,20,21 but a high carrier concentration leads to severe recombination, so overall water splitting has not yet been achieved with oxygen doped ZnIn2S4. The surface sulfur vacancies can be used as electron trapping sites to promote the separation and migration of photogenerated carriers of ZnIn2S4.13,22,23 Therefore, the simultaneous introduction of surface sulfur vacancies and oxygen doping in ZnIn2S4 may achieve efficient overall water splitting.
In this work, ZnIn2S4 with surface sulfur vacancies and oxygen atom doping is obtained via calcining a hydrothermal preparation of initial ZnIn2S4 under an air atmosphere. The amount of surface sulfur vacancies and oxygen atoms on ZnIn2S4 is controlled by adjusting the calcination temperature and calcination time. It is found that sulfur vacancies and oxygen atoms have a great effect on the activity of overall water splitting. The photocatalytic H2 evolution rate of ZnIn2S4 with an optimal amount of sulfur vacancies and oxygen atoms is ∼13 times as high as that of the initial ZnIn2S4. Based on the results of the surface chemical state, photoelectrochemical test, photocatalytic performance, etc., the synergistic effect of the two factors is explored in detail.
2. Experiment
2.1 Preparation of ZnIn2S4 with sulfur vacancies and oxygen atom doping
The hydrothermal preparation of initial ZnIn2S4 was obtained according to the reported literature.13 The prepared ZnIn2S4 (0.5 g) was then calcined at different temperatures and times under an air atmosphere. The products calcined at 350 °C for 1 h, 4 h and 7 h were denoted as ZnIn2S4-350-1h, ZnIn2S4-350-4h and ZnIn2S4-350-7h, respectively. The products calcined at 200 °C and 500 °C for 4 h were denoted as ZnIn2S4-200-4h and ZnIn2S4-500-4h, respectively.
2.2 Photocatalytic overall water splitting activity measurements
The photocatalytic overall water splitting performance was tested using a Labsolar-IIIAG system (Perfectlight). The cocatalyst was loaded onto the sample by the photodeposition method. Typically, H2PtCl6 (1.5 wt% Pt), Cr(NO3)3 (0.5 wt% Cr) and the photocatalyst (50 mg) were added to 100 mL of pure water. The suspension was stirred and irradiated with a 300 W Xenon lamp for 1 h under a vacuum environment. Prior to photocatalytic water splitting, the suspension was evacuated to remove dissolved gas. A 300 W Xenon lamp (Perfectlight, PLX-SXE300) equipped with a UV cutoff filter was used to provide visible light (λ ≥ 420 nm). |  | (1) |
To obtain the STH efficiency, 100 mg photocatalyst and a 300 W xenon lamp with an AM 1.5G filter (100 mW cm−2) were used.
|  | (2) |
2.3 Photoelectrochemical measurements
An electrochemical workstation (CHI-760E) was used to test the photoelectric chemical properties in a three-electrode system. The working electrode was prepared using a reported electrophoretic deposition method.13,24 Electrophoresis was performed for 5 min at a 10 V constant bias. The prepared electrode was dried at 100 °C in air for 1 h. Platinum foil (10 × 10 mm) and a Hg/HgO electrode were used as the counter and reference electrodes, respectively. A KOH solution (0.1 M, pH = 13) was used as an electrolyte.
The chemical section and characterization section are shown in the ESI.†
3. Results and discussion
3.1 Structural characteristics
As shown in Fig. 1a, the initial ZnIn2S4 obtained by the hydrothermal method is a hexagonal phase, and the crystalline phase remains unchanged after calcination at 350 °C for several hours. Fig. 1b shows that the calcination temperature has a great effect on the crystal phase of ZnIn2S4. A large amount of sulfur is oxidized after calcination at 500 °C for the same period of time, and the crystal phase undergoes a large transformation (the main crystal phase is In2O3). Therefore, ZnIn2S4 with a calcination temperature below 500 °C is mainly studied. Fig. 1c shows that the initial ZnIn2S4 is composed of nanosheets, but the nanosheets are severely agglomerated after calcination at 350 °C for 4 h (Fig. 1d). The N2 absorption–desorption isotherm also confirms the agglomeration of the ZnIn2S4-350-4h sample. As shown in Fig. S1,† its hysteresis loop is weaker than that of the initial ZnIn2S4, indicating that the amount of slit-shaped mesopores formed by the nanoplate aggregation decreases.25 The BET surface area is also reduced from 105.7 m2 g−1 for the initial ZnIn2S4 to 41.4 m2 g−1 for ZnIn2S4-350-4h. The elemental mapping image of ZnIn2S4-350-4h shows that an O element is introduced, indicating that a part of the sulfur atoms is oxidized. Although agglomeration results in a decrease in specific surface area, the presence of oxygen doping and vacancies caused by the oxidation of sulfur may improve the carrier separation efficiency.
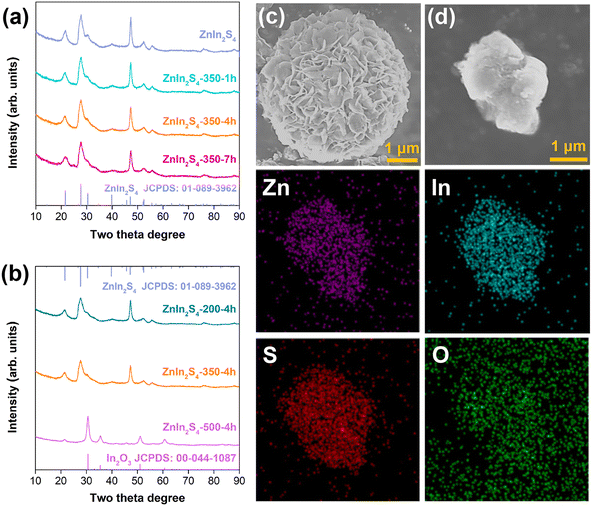 |
| Fig. 1 (a) X-ray diffraction (XRD) patterns of the initial ZnIn2S4 and the ZnIn2S4 calcined at 350 °C for different times; (b) XRD patterns of the ZnIn2S4 calcined for 4 h at different temperatures; (c) field emission scanning electron microscopy (FESEM) image of the initial ZnIn2S4; (d) FESEM image and the corresponding elemental mapping images of ZnIn2S4-350-4h. | |
3.2 Surface chemical analysis
As shown in Fig. 2a and b, the binding energies of Zn 2p do not change after the initial ZnIn2S4 is calcined in air, while the binding energies of In 3d increase by 0.1 eV for all the calcined samples except the ZnIn2S4-500-4h sample. This phenomenon can be assigned to the O atom replacing the S atom in the In–S bond but not that in the Zn–S bond, because the lower negativity of the O atom decreases the electron density of the surrounding In atom and the more “oxidizing” environment increases its binding energies.26 The In 3d binding energies of ZnIn2S4-500-4h located at 444.48 eV and 452.03 eV are ascribed to In3+ in In2O3.27 It is further demonstrated that the high temperature calcination changes the crystal phase, which is consistent with the XRD results. Fig. 2c shows that the binding energies of the S element also increases after calcination, indicating the presence of oxygen atoms around S. According to the change in binding energies of O 2p, the binding energies located at 529.90 eV indicate the presence of lattice oxygen with the increase of calcination temperature or calcination time. The binding energy located at 169.00 eV of the ZnIn2S4-350-4h, ZnIn2S4-350-7h and ZnIn2S4-500-4h samples (Fig. 2c and S2†) is ascribed to the characteristic peak of the O–S bond.21,28 XPS was also used to detect the chemical states of the cocatalysts. As shown in Fig. S3,† the binding energies of Pt 4f are located at 75.73 eV and 72.34 eV, and the binding energies of Cr 3d are located at 586.00 eV and 576.10 eV (the peak at 585.00 eV is the Auger electron peak of Zn (ref. 29)). This indicates that the cocatalyst was successfully loaded on the surface of ZnIn2S4-350-4 h by Pt–S bonds and Cr–S bonds.30–33 The TEM mapping (Fig. S4†) further indicates that the cocatalysts are uniformly dispersed on the surface of ZnIn2S4-350-4h. FTIR spectra show that the characteristic peaks of bidentate bonded SO42− (1048 cm−1, 1112 cm−1) appear after calcination in air.34 Therefore, the increase of S 2p binding energies is attributed to the formation of sulfate anions. The EPR signal at g = 2.005 for all the samples indicates the presence of surface sulfur vacancies.21,35 The enhanced EPR signal intensity of the calcined sample indicates that S vacancies can be introduced by calcination. With the increase of calcination time, the surface S vacancies first increased and then decreased, indicating that sulfur vacancies are generated first and then gradually filled via oxygen atom doping. Therefore, it can be inferred that calcination in air results in the formation of surface sulfur vacancies and O atom doping.
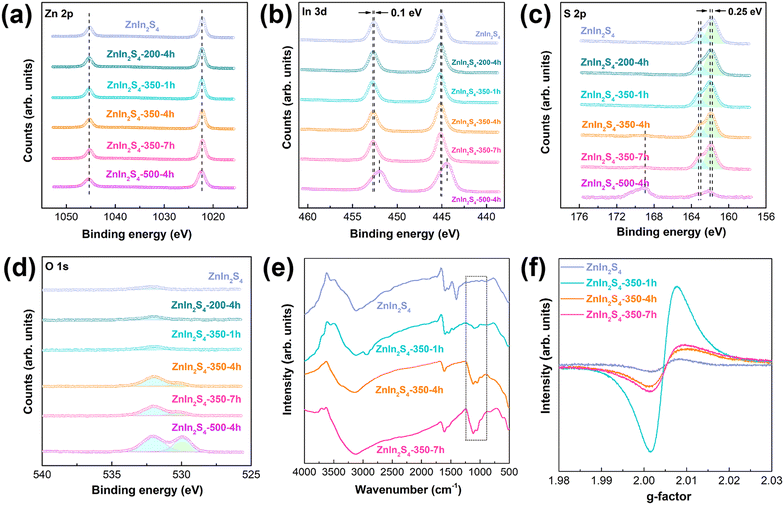 |
| Fig. 2 X-ray photoelectron spectroscopy (XPS) spectra of the samples: (a) Zn 2p peaks, (b) In 3d peaks, (c) S 2p peaks and (d) O 1s peaks; (e) Fourier transform infrared (FTIR) spectra and (f) electron paramagnetic resonance (EPR) spectra of the samples. | |
3.3 Band gap characterization
Fig. 3a shows that all the samples have a wide visible-light absorption range. Comparing the initial ZnIn2S4 with the calcined ZnIn2S4, it can be seen that both sulfur vacancies and O atom doping can improve the light absorption ability. As shown in Fig. 3b, the band gap value decreases from 2.53 eV to 2.43 eV. Comparing the ZnIn2S4-350-1h, ZnIn2S4-350-4h and ZnIn2S4-350-7h samples, an appropriate amount of sulfur vacancies and O doping results in the optimal light absorption ability. In addition, the band gap value of the ZnIn2S4-500-4h sample is greatly increased due to the crystal phase change caused by excessive oxidation. The band structure of the samples is further obtained by Mott–Schottky measurements. Fig. 3c shows that the samples have a positive MS slope, indicating that they are n-type semiconductors. The flat-band potential of the two samples is located at −0.65 eV. The XPS valence band (VB) spectra of the samples indicate that the energy gaps of ZnIn2S4-350-4h and ZnIn2S4 between the Fermi level and the valence band maximum (VBM) are 1.53 and 1.45 eV, respectively (Fig. S5†). The Fermi level of an n-type semiconductor is approximately equal to the flat band potentials,36–38 so the VBM potentials of ZnIn2S4-350-4h and ZnIn2S4 are calculated to be +0.88 and +0.80 eV, respectively. Based on the band gap, the conduction band minimum (CBM) potentials of ZnIn2S4-350-4h and ZnIn2S4 are calculated to be −1.55 eV and −1.73 eV, respectively. The band structures of the initial ZnIn2S4 and ZnIn2S4-350-4h are shown in Fig. 3d. It can be seen that the synergistic effect of O atom doping and surface sulfur vacancies moves the CBM and VBM downward.
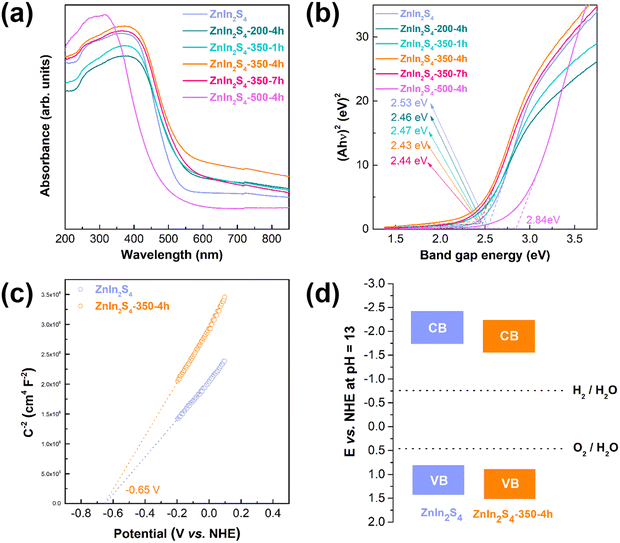 |
| Fig. 3 (a) UV-vis absorption spectra and (b) band gap energies of the prepared samples; (c) Mott–Schottky plot and (d) band edge positions of the initial ZnIn2S4 and ZnIn2S4-350-4h samples. | |
3.4 Photocatalytic activity and mechanism
Fig. 4a and b show that all the hexagonal ZnIn2S4 samples have photocatalytic overall water splitting activity under visible-light irradiation. The molar ratio of H2 to O2 is close to 2
:
1, indicating that stoichiometric water splitting actually occurs. The ZnIn2S4-500-4h sample does not have the ability for overall water splitting due to the change of its crystal phase. This indicates that the structure of ZnIn2S4 is beneficial to the utilization of charge carriers, and appropriate modification can further enhance its photocatalytic activity. As shown in Fig. 4c and d, when abundant sulfur vacancies are introduced into the initial ZnIn2S4, the photoluminescence (PL) intensity of ZnIn2S4-350-1h decreases significantly and the electron lifetime is increased from 10.24 ns to 12.35 ns (the average lifetime 〈τ〉 is calculated through the following equation: τ = (A1τ12 + A2τ22)/(A1τ1 + A2τ2)39,40). Compared with the initial ZnIn2S4, the water splitting rate of ZnIn2S4-350-1h has a two-fold increase, indicating that the abundant sulfur vacancies can inhibit carrier recombination by prolonging the electron lifetime. As shown in Fig. 4e, the photocurrent density of the ZnIn2S4-350-1h sample is similar to that of the initial ZnIn2S4 sample, indicating that the abundant sulfur vacancies do not increase the photogenerated carrier concentration. The water splitting rate of the ZnIn2S4-350-4h sample is ∼4 times as high as that of the ZnIn2S4-350-1h sample. Compared with ZnIn2S4-350-1h, the sulfur vacancy concentration of ZnIn2S4-350-4h decreases but the oxygen doping concentration increases. The lower PL intensity, longer electron lifetime and higher photocurrent density of ZnIn2S4–350-4h indicate that oxygen doping can increase the photogenerated carrier concentration and decrease recombination. Comparing the PL intensity, electron lifetime, photocurrent density and photocatalytic activity of the ZnIn2S4-350-7h and ZnIn2S4–350-4h samples, it can be seen that the amount of oxygen atom doping should not be too much. The initial ZnIn2S4 is also calcined in a nitrogen atmosphere for 4 h at 350 °C to obtain ZnIn2S4 containing only sulfur vacancies (denoted as ZnIn2S4-350-4h-N2). Its photocatalytic activity is ∼4 times as high as that of the initial ZnIn2S4 (Fig. S6†), which further confirms that the excellent photocatalytic performance of ZnIn2S4-350-4h is due to the synergistic effect of oxygen doping and sulfur vacancies. The photocatalytic activity of ZnIn2S4-350-4h is ∼3 times as high as that of ZnIn2S4-350-4h-N2, so both sulfur vacancies and oxygen atom doping play important roles in improving photocatalytic activity. Fig. 4f shows that the semicircular arcs of the Nyquist plots of the samples are similar, indicating that the introduction of sulfur vacancies and oxygen atom doping provides limited improvement in the transfer efficiency of surface carriers. In other words, they mainly improve the separation efficiency of photogenerated carriers in the bulk. In addition, the synergistic effects of oxygen atom doping and sulfur vacancies of the ZnIn2S4-350-4h sample result in an apparent quantum efficiency (AQE) at 420 nm of 0.21% (Fig. 4g) and a STH efficiency of ∼0.035% (Fig. 4h; Table S1;† the highest value for single-phase ZnIn2S4 reported so far). More importantly, ZnIn2S4-350-4h also has excellent photocatalytic water splitting stability. Fig. 4i shows that the overall water splitting rate basically remains unchanged after four cycles. Fig. S7† shows that the surface state of the elements and the crystal phase do not change before and after the photocatalytic reaction.
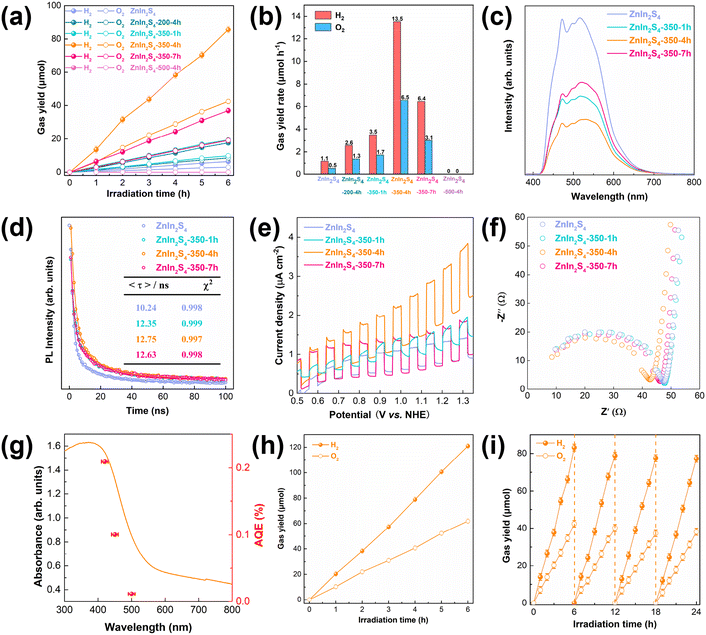 |
| Fig. 4 Photocatalytic overall water splitting performance (a) and the corresponding rates (b) of the as-prepared samples (50 mg; λ ≥ 420 nm); PL spectra (c) and time-resolved PL (TPRL) spectra (d) of the as-prepared samples; liner sweep voltammetry (e) and impedance spectra (f) of the photoanodes prepared from different samples under visible-light illumination (λ ≥ 420 nm); (g) action spectra of the ZnIn2S4-350-4h sample for overall water splitting; (h) photocatalytic overall water splitting of the ZnIn2S4-350-4h sample under AM 1.5G illumination (100 mg; 100 mW cm−2); (i) repeated cycles of photocatalytic overall water splitting for the ZnIn2S4-350-4h sample (50 mg; λ ≥ 420 nm). | |
4. Conclusions
In summary, we explored the effects of sulfur vacancies and oxygen atom doping on the photocatalytic activity of ZnIn2S4 in detail. The calcination temperature and time have a significant effect on the amount of sulfur vacancies and oxygen atom doping. The synergistic effect of sulfur vacancies and oxygen atom doping can increase the photogenerated carrier lifetime and concentration as well as inhibit carrier recombination in the bulk. Therefore, the photocatalytic hydrogen rate of ZnIn2S4-350-4h with an appropriate amount of sulfur vacancies and oxygen atoms is ∼13 times as high as that of the initial ZnIn2S4. Through the selective introduction of sulfur vacancies or oxygen atom doping, it is found that they have the same importance for photocatalysis. This work may provide a reference for the design of other semiconductor photocatalysts.
Conflicts of interest
There are no conflicts to declare.
Acknowledgements
This work was supported by the National Natural Science Foundation of China (Grant no. 51872186, 22066024, and 52062023), the Shanghai Sailing Program (Grant No. 20YF1435100), and a project funded by the China Postdoctoral Science Foundation (Grant No. 2021M702316).
Notes and references
- S. Chen, T. Takata and K. Domen, Nat. Rev. Mater., 2017, 2, 1–17 Search PubMed
.
- R. Pan, M. Hu, J. Liu, D. Li, X. Wan, H. Wang, Y. Li, X. Zhang, X. Wang, J. Jiang and J. Zhang, Nano Lett., 2021, 21, 6228–6236 CrossRef CAS PubMed
.
- S. Wei, S. Chang, J. Qian and X. Xu, Small, 2021, 17, 2100084 CrossRef CAS
.
- S. Chang, J. Yu, R. Wang, Q. Fu and X. Xu, ACS Nano, 2021, 15, 18153–18162 CrossRef CAS
.
- T. Hisatomi, K. Takanabe and K. Domen, Catal. Lett., 2015, 145, 95–108 CrossRef CAS
.
- C.-F. Fu, J. Sun, Q. Luo, X. Li, W. Hu and J. Yang, Nano Lett., 2018, 18, 6312–6317 CrossRef CAS PubMed
.
- K. Maeda and K. Domen, J. Phys. Chem. Lett., 2010, 1, 2655–2661 CrossRef CAS
.
- Q. Wang, M. Nakabayashi, T. Hisatomi, S. Sun, S. Akiyama, Z. Wang, Z. Pan, X. Xiao, T. Watanabe, T. Yamada, N. Shibata, T. Takata and K. Domen, Nat. Mater., 2019, 18, 827 CrossRef CAS PubMed
.
- G. Zhang and X. Wang, Angew. Chem., Int. Ed., 2019, 58, 15580–15582 CrossRef CAS PubMed
.
- Z. Wang, Y. Inoue, T. Hisatomi, R. Ishikawa, Q. Wang, T. Takata, S. Chen, N. Shibata, Y. Ikuhara and K. Domen, Nat. Catal., 2018, 1, 756–763 CrossRef CAS
.
- J. Xu, C. Pan, T. Takata and K. Domen, Chem. Commun., 2015, 51, 7191–7194 RSC
.
- C. Pan, T. Takata, M. Nakabayashi, T. Matsumoto, N. Shibata, Y. Ikuhara and K. Domen, Angew. Chem., Int. Ed., 2015, 54, 2955–2959 CrossRef CAS
.
- H. Jing, G. Xu, B. Yao, J. Ren, Y. Wang, Z. Fang, Q. Liang, R. Wu and S. Wei, ACS Appl. Energy Mater., 2022, 5(8), 10187–10195 CrossRef CAS
.
- C. Zhu, C. Liu, Y. Zhou, Y. Fu, S. Guo, H. Li, S. Zhao, H. Huang, Y. Liu and Z. Kang, Appl. Catal., B, 2017, 216, 114–121 CrossRef CAS
.
- X. Cai, Z. Zeng, Y. Liu, Z. Li, X. Gu, Y. Zhao, L. Mao and J. Zhang, Appl. Catal., B, 2021, 297, 120391 CrossRef CAS
.
- B. Sun, D. Fan, X. Chen, Z. Li, W. Zhou and Y. Du, Mater. Chem. Front., 2022, 6, 1795–1802 RSC
.
- R. Pan, M. Hu, J. Liu, D. Li, X. Wan, H. Wang, Y. Li, X. Zhang, X. Wang and J. Jiang, Nano Lett., 2021, 21, 6228–6236 CrossRef CAS PubMed
.
- Y. Ding, D. Wei, R. He, R. Yuan, T. Xie and Z. Li, Appl. Catal., B, 2019, 258, 117948 CrossRef CAS
.
- B. Sun, J. Bu, X. Chen, D. Fan, S. Li, Z. Li, W. Zhou and Y. Du, Chem. Eng. J., 2022, 435, 135074 CrossRef CAS
.
- W. Yang, L. Zhang, J. Xie, X. Zhang, Q. Liu, T. Yao, S. Wei, Q. Zhang and Y. Xie, Angew. Chem., Int. Ed., 2016, 55, 6716–6720 CrossRef CAS
.
- W. Xu, W. Gao, L. Meng, W. Tian and L. Li, Adv. Energy Mater., 2021, 11, 2101181 CrossRef CAS
.
- C. Du, Q. Zhang, Z. Lin, B. Yan, C. Xia and G. Yang, Appl. Catal., B, 2019, 248, 193–201 CrossRef CAS
.
- R. Jiang, X. Cai, X. Gu, D. Yang, J. Zhang, Y. Zhao and L. Mao, J. Mater. Sci., 2021, 56, 19439–19451 CrossRef CAS
.
- S. Wei, Y. Chen, P. Wu, X. Liu, J. Ren, B. Yao, H. Xu, W. Dou, Y. Wang and R. Wu, Appl. Catal., A, 2022, 640, 118675 CrossRef CAS
.
- J. Li, C. Wu, J. Li, B. Dong, L. Zhao and S. Wang, Chin. J. Catal., 2022, 43, 339–349 CrossRef CAS
.
- F. Wu, G. Liu and X. Xu, J. Catal., 2017, 346, 10–20 CrossRef CAS
.
- C. Li, T. Ming, J. Wang, J. Wang, C. Y. Jimmy and S. H. Yu, J. Catal., 2014, 310, 84–90 CrossRef CAS
.
- L. Meng, X. Zhou, S. Wang, Y. Zhou, W. Tian, P. Kidkhunthod, S. Tunmee, Y. Tang, R. Long and Y. Xin, Angew. Chem., Int. Ed., 2019, 58, 16668–16675 CrossRef CAS PubMed
.
- H. L. Liu, L. H. Fei, H. B. Liu, J. H. Yang, X. Jin, M. Gao, Y. Liu, X. Cheng and X. Zhang, J. Mater. Sci.: Mater. Electron., 2013, 24, 58–63 CrossRef CAS
.
- J. Zhu, L. Cai, X. Yin, Z. Wang, L. Zhang, H. Ma, Y. Ke, Y. Du, S. Xi and A. T. Wee, ACS Nano, 2020, 14, 5600–5608 CrossRef CAS PubMed
.
- X. Shi, C. Dai, X. Wang, J. Hu, J. Zhang, L. Zheng, L. Mao, H. Zheng and M. Zhu, Nat. Commun., 2022, 13, 1–10 Search PubMed
.
- L. Zhang, J. Qiu, D. Dai, Y. Zhou, X. Liu and J. Yao, J. Cleaner Prod., 2022, 341, 130891 CrossRef CAS
.
- C. Fan, R. Yang, Y. Liu, D. Chen, L. Chen, R. Liu, Y. Yan, Y. Zou and Y. Xu, Int. J. Energy Res., 2021, 45, 10304–10316 CrossRef CAS
.
- R. Zhang, L. Wang, L. Pan, Z. Chen, W. Jia, X. Zhang and J.-J. Zou, Appl. Catal., B, 2020, 277, 119237 CrossRef CAS
.
- L. Zeng, S. Chen, J. van der Zalm, X. Li and A. Chen, Chem. Commun., 2019, 55, 7386–7389 RSC
.
- W. Wang, P. Xu, M. Chen, G. Zeng, C. Zhang, C. Zhou, Y. Yang, D. Huang, C. Lai and M. Cheng, ACS Sustainable Chem. Eng., 2018, 6, 15503–15516 CrossRef CAS
.
- H. Huang, K. Xiao, N. Tian, F. Dong, T. Zhang, X. Du and Y. Zhang, J. Mater. Chem. A, 2017, 5, 17452–17463 RSC
.
- V. Subramanian, E. E. Wolf and P. V. Kamat, J. Am. Chem. Soc., 2004, 126, 4943–4950 CrossRef CAS PubMed
.
- C.-L. Tan, M.-Y. Qi, Z.-R. Tang and Y. J. Xu, Appl. Catal., B, 2021, 298, 120541 CrossRef CAS
.
- C. Du, B. Yan and G. Yang, Nano Energy, 2020, 76, 105031 CrossRef CAS
.
|
This journal is © The Royal Society of Chemistry 2023 |