Solution-grown millimeter-scale Mn-doped CsPbBr3/Cs4PbBr6 crystals with enhanced photoluminescence and stability for light-emitting applications†
Received
9th September 2023
, Accepted 21st November 2023
First published on 22nd November 2023
Abstract
Metal halide perovskites are particularly emerging for optoelectronic applications in light-emitting diodes, photodetectors, and solar cells due to their flourishing photophysical properties. However, the poor stability of three-dimensional (3D) lead halide perovskite nanocrystals (NCs) significantly hampers their optoelectronics and photovoltaics applications. Embedding 3D perovskites into zero-dimensional (0D) perovskite crystals and doping ions of appropriate elements into host lattices provide effective approaches to improve the stability and optical-electronic performance. In this study, millimeter-scale Mn-doped and undoped CsPbBr3/Cs4PbBr6 perovskite crystals were successfully fabricated by a one-step slow cooling method. We systematically investigated the effects of Mn2+ ion doping on the PL performance and stability of CsPbBr3/Cs4PbBr6 crystals. Compared with undoped crystals, the existence of Mn2+ ions not only blue-shifted the PL peak but also improved the luminescence performance and stability of the prepared millimeter-sized crystals. Moreover, doping Mn2+ ions can increase the proportion of radiative recombination at low temperature, which may be because Mn2+ ions can effectively accelerate the decay of a dark exciton by the magnetic mixing of bright and dark excitons. In addition, green LED devices with high efficiency packaged as-grown crystals are explored, which promises further application in display backlights.
1 Introduction
All-inorganic lead halide perovskites, such as three-dimensional (3D) CsPbX3 nanocrystals (NCs), have emerged as new promising semiconductor photoelectric materials due to their high photoluminescence quantum yield (PLQY), tunable photoluminescence (PL) wavelength, narrow-band emission, high colour purity, and high carrier mobility.1–6 In particular, perovskites fabricated by wet chemical approaches have potential applications in high-performance displays,7 light-emitting diodes,8–11 photovoltaic cells,12–18 solid-state lasers,19,20 photodetectors,21 and solar fuel production.22 Compared with 3D perovskite NCs, large-sized zero-dimensional (0D) perovskite crystals typically show better stability and are more adaptable for practical applications, while showing relatively weak PL.23 Therefore, the combination of 0D perovskite NCs and 3D perovskites has attracted wide attention and has been used in various types of optoelectronic devices.23 Cesium-lead halide perovskites are normally classified as 3D CsPbBr3, two-dimensional (2D) CsPb2Br5, and 0D Cs4PbBr6 based on the connectivity of the corner-sharing [PbBr6]4− octahedron.24 The PLQY of the core/shell structure of CsPbBr3/Cs4PbBr6 can reach up to 87.23%, compared with the single crystals.25 Many methods have been developed to fabricate those kinds of composites. By solution-phase synthesis,26 the preparation of Cs4PbBr6/CsPbBr3 nanocomposite microcrystals with high PLQY (55%) can be achieved. An HBr-assisted slow cooling method (SCM) also provided a new way to prepare the centimeter-scale CsPbBr3 NCs embedded in Cs4PbBr6 crystals, which showed a strong green PL emission with an absolute PLQY of up to 97%.27 Although the PLQYs of CsPbBr3/Cs4PbBr6 composites have been significantly enhanced via various strategies, the optical and thermal stabilities still lack significant improvements,28,29 which hinder the practical applications of those kinds of composites.
Doping impurity ions into crystals has proven to be an effective way to enhance the environmental stability and adjust the optoelectronic properties of conventional colloidal II–VI and III–V semiconductor materials,30 which also happens to perovskite NCs. For instance, Zou et al. reported that doping Mn2+ ions into the CsPbBr3 NCs can stabilize the exciton emission and improve the thermal stability.31 Doping lanthanide into CsPbCl3 NCs can significantly improve and expand the NCs’ optical properties.32 Moreover, theoretical studies of first-principles calculations have confirmed that CsPbBr3 perovskites doped with Mn2+, Sr2+, Sn2+, Co2+, Zn2+ and Cd2+ ions have enhanced thermodynamic stability.33,34 Many efforts have been dedicated to investigate the effect of doping ions on the luminescence properties of perovskite nanocrystals, while there are relatively few studies on large-scaled perovskite crystals. Recently, doping Na ions into the centimeter-sized CsPb2Br5 single crystals exhibited an interesting self-trapped exciton emission and an enhanced PLQY.35 Although doping ions offers an effective way to improve the optoelectronic properties of perovskite materials, no attempts have been made to investigate the doping influence on the properties of large-scale 3D–0D perovskite crystals. In additional, doping ions typically exhibited a large change in the PL emission,35,36 while the effect of a slight adjustment of the PL emission is relatively rare. Obtaining solution-grown ion-doped large-scale perovskite crystals is not only important for achieving a deeper understanding of the underlining doping mechanism and related photophysics, but also crucial for the practical applications of optoelectronic devices.
In this work, the millimeter-scale Mn2+-doped and undoped CsPbBr3/Cs4PbBr6 crystals were synthesized by one-step slow cooling method under an air atmosphere. Upon adding Mn2+ ions, a blueshift in the PL spectrum was observed, without changing the full-width-at-half-maximum (FWHM) of the PL spectra and generating a new excitonic emission. Compared with the undoped crystals, introducing Mn2+ ions can improve the proportion of radiative recombination when the temperature is below 130 K, while there is almost a negligible effect on the PL decay dynamics at higher temperature. Moreover, Mn2+ ions can improve the stability of the prepared millimeter-scale crystals. Based on the as-grown crystals, high efficiency and finely-tunable electroluminescence green LEDs were successfully fabricated, providing an alternative choice for display backlights.
2 Experimental section
2.1. Chemicals
All chemical materials and reagents were used directly as received without any further purification. PbBr2 (99%) and MnBr2 (99%) were commercially obtained from Shanghai Boer Chemical Reagent Technology Co., Ltd (Shanghai, China). CsBr (99.9%) was purchased from Shanghai Titan Technology Co., Ltd (Shanghai, China). HBr (48 wt% in water) was purchased from Shanghai Aladdin Biochemical Technology Co., Ltd (Shanghai, China). N,N-Dimethylformamide (DMF, 99.8%) was purchased from Shanghai Aladdin Biochemical Technology Co., Ltd (Shanghai, China). Toluene (99.5%) was purchased from Titan Technology Co., Ltd (Shanghai, China).
2.2. Synthesis of millimeter-scale CsPbBr3/Cs4PbBr6 crystals
Millimeter-scale CsPbBr3/Cs4PbBr6 crystals were synthesized according to a one-step slow cooling method.27 The synthesis process is described as follows. A mixture of PbBr2 (2.5 mmol, 0.917 g) and CsBr (10 mmol, 2.130 g) were added at room temperature to a 100 mL round bottom flask, which contained 50 mL DMF and HBr (the volume ratio of DMF and HBr is 5
:
4). The temperature of the crystal growth was controlled using an external water bath, with a temperature accuracy of ± 0.01 °C. The mixture solution was first heated and stirred to 80 °C in a water bath. After it was completely dissolved into a transparent solution, it was taken out and cooled to room temperature. About three days later, the millimeter-scale CsPbBr3/Cs4PbBr6 crystals were successfully fabricated at the bottom of the flask. Thereafter, the pure CsPbBr3/Cs4PbBr6 crystals were obtained by washing and filtering with toluene. The schematic of the synthesis process flow chart is shown in Fig. 1.
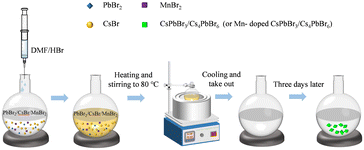 |
| Fig. 1 Schematic diagram of the process of synthesizing Mn-doped and undoped CsPbBr3/Cs4PbBr6 crystals by slow-cooling method. | |
2.3. Procedure for the synthesis of millimeter-scale Mn-Doped CsPbBr3/Cs4PbBr6 crystals
Millimeter-scale Mn-doped CsPbBr3/Cs4PbBr6 crystals were also synthesized according to a one-step slow cooling method,27 and made some improvement. Firstly, the mixture of PbBr2 (2.5 mmol, 0.917 g), CsBr (10 mmol, 2.130 g) and MnBr2 (1 mmol, 0.215 g) were added to a flask at room temperature. Then, 50 mL of the DMF/HBr solution (the volume ratio of DMF and HBr is 5/4) was subsequently mixed with the abovementioned mixture. The solution was then heated and stirred to 80 °C using an external water bath (an accuracy of ± 0.01 °C), and subsequently dissolved completely into a transparent solution and cooled to room temperature. About three days later, the millimeter-scale Mn-doped CsPbBr3/Cs4PbBr6 crystals were successfully grown at the bottom of the flask.
2.4. Fabrication of green LEDs
Green CsPbBr3/Cs4PbBr6 crystals with and without Mn-doping and AB organic silicone (the A-to-B mass ratio of 1
:
2) were added to a mortar. The mixture was stirred fully until all bubbles disappeared completely, and then the obtained mixture was spread evenly. The mixture was coated onto the surface of the blue InGaN LED chip, followed by heating and curing in an oven at 120 °C for 2 hours. The green LED devices were successfully fabricated for further optical performance testing.
2.5. Characterization
Microscopy photographs of the as-grown crystals were obtained using the SZM continuous zoom stereo microscope (Sunny Optical Technology Co., Ltd). PL spectra were recorded by fluorescence spectrometry (Edinburgh Instrument Spectrofluorometer FS5) equipped with a solid-state sample holder. A flashlamp (λex = 405 nm) was used for gathering the data on the decay under time-correlated single-photon counting (TCSPC) mode. X-Ray diffraction (XRD) data were collected with 2θ from 10 to 60° using a Rigaku Ultima IV X-ray diffractometer (40 kV, 40 mA, sealed Cu X-ray tube). Transmission electron microscopy (TEM) and energy dispersive spectrometer (EDS) data of the as-grown crystals were obtained by a JEM-2100 high-resolution transmission electron microscope (HRTEM, JEOL, Tokyo, Japan). Time-resolved PL measurements were characterized at various temperatures using an Edinburgh FLS980 spectrometer. Excitation was provided by an Edinburgh EPL-365 picosecond pulsed diode laser and probed at 520 nm. Temperature-dependent PL experiments were performed using the FX4000-Ex high-resolution spectrometer (Shanghai Fuxiang Optics Co., Ltd) and the MS-H280-Pro heating table (Dalong Xingchuang Experimental Instrument (Beijing) Co., Ltd) with a temperature range of 300 to 400 K. Moisture stability experiments were performed by measuring the PL intensities of the prepared crystals as a function of time using the FX4000-Ex high-resolution spectrometer (Shanghai Fuxiang Optical Co., Ltd) and a humidifier. The humidifier and the crystals were placed together in a plastic box with a humidity control of 70%. The photostability was characterized by recording the corresponding PL intensities of the crystals under the laser irradiation (with a central wavelength of 455 nm and a power density of 5 W cm−2) generated by 100 W optical fiber output laser (Yantai Huachuang Intelligent Equipment Co., Ltd).
3 Results and discussion
3.1. Structure and optical properties
Fig. 2a shows the photographs of an as-grown single CsPbBr3/Cs4PbBr6 crystal and a pure Cs4PbBr6 single crystal without further polishing under the microscope. The CsPbBr3/Cs4PbBr6 crystals show good transparency and an apparent yellow-green colour under daylight with a size of 3.0 × 3.0 × 1.045 mm (see Fig. 2a and Fig. S1, ESI†), while a typical pure Cs4PbBr6 single crystal presents as white and transparent with a size of 2.0 × 2.0 × 1.018 mm (see Fig. 2b and Fig. S1, ESI†). Under UV light (395 nm) irradiation, the CsPbBr3/Cs4PbBr6 crystals show bright green emission, while the pure Cs4PbBr6 single crystals do not emit any light (Fig. S1, ESI†). This indicates that the green emission is attributed to the existence of the CsPbBr3 phase, which is in line with the literature.37 The PL spectrum of the CsPbBr3/Cs4PbBr6 crystals shows a PL peak at 518 nm with a narrow FWHM of 21 nm, which is comparable to that reported for the Cs4PbBr6 crystals with embedded CsPbBr3 NCs with a CsPbBr3 NCs average size of 2.85 nm.27 The PLQY of the as-grown CsPbBr3/Cs4PbBr6 crystals was 46.02% (as shown in Fig S4, ESI†). Moreover, the UV-Vis diffuse reflection spectrum (Fig. 2c) obtained from the grinding powder of the CsPbBr3/Cs4PbBr6 crystals apparently shows two clear absorption peaks at about 310 and 513 nm, corresponding to the characteristic absorption of Cs4PbBr6 caused by the allowed optical transition of 3P1 to 1S0 of the Pb2+ ions38 and the CsPbBr3 NCs, respectively. The existence of 3D CsPbBr3 NCs in the powder XRD is almost undetectable. Minor XRD peaks corresponding to CsPbBr3 can be found due to the low proportion of CsPbBr3 in the composite, which has also been reported in previous studies.39,40 However, the existence of the CsPbBr3 phase can be clearly demonstrated from the comparison of the daylight photographs, as well as the absorption and PL spectra. In the following, we further confirm from the structure that the 3D CsPbBr3 NCs are embedded in the Cs4PbBr6 matrices.
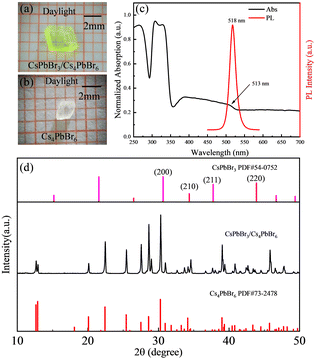 |
| Fig. 2 (a) and (b) Photographs of the as-grown CsPbBr3/Cs4PbBr6 crystal and Cs4PbBr6 single crystal under the microscope under daylight. (c) Absorption (black curve) and PL spectra (red curve, excitation by CW laser wavelength of λex = 365 nm) of CsPbBr3/Cs4PbBr6 crystals. (d) The powder XRD pattern of the as-grown CsPbBr3/Cs4PbBr6 crystals. | |
To further characterize the morphology of the as-grown CsPbBr3/Cs4PbBr6 crystals, we conducted TEM measurements. Since TEM cannot be used to measure large crystals, the as-grown crystals are processed into small particles by ball-milling, which are then dispersed in toluene under sonication for TEM measurement. During this process, the PL emission remains consistent. Fig. 3 shows a high-resolution TEM (HRTEM) of a typical sample. As shown in Fig. 3a, the particles processed from the large-sized crystals have a lattice spacing of 6.3 Å, corresponding to the (110) phase of Cs4PbBr6. Fig. 3b and c show the corresponding enlarged TEM image of panel (a), where the lattice spacing is indicated to be 2.9 and 4.5 Å, respectively, which corresponds to the (200) and (110) phases of CsPbBr3. Thus, from the TEM image shown in Fig. 3, it is directly confirmed that the CsPbBr3 NCs are embedded in the Cs4PbBr6 crystals. Moreover, the HRTEM images of the CsPbBr3/Cs4PbBr6 crystals and the corresponding FFT patterns are shown in Fig. S3 (ESI†), and match well with the standard diffraction patterns of the CsPbBr3 and Cs4PbBr6 phases, further validating the existence of CsPbBr3 NCs embedded in the Cs4PbBr6 crystals.
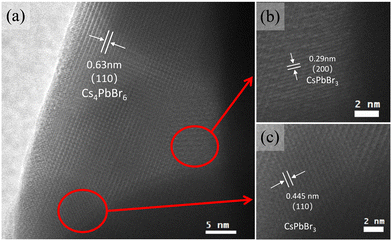 |
| Fig. 3 (a) Typical TEM image of a particle processed from the as-grown CsPbBr3/Cs4PbBr6 crystals. (b) The corresponding enlarged TEM image of panel (a) plotted with the lattice spacing of the CsPbBr3 phase. (c) Another corresponding enlarged TEM image of panel (a) plotted with a lattice spacing of the CsPbBr3 phase. | |
In order to further investigate the effect of ion doping on the luminescence performance and stability of the 3D–0D type perovskites, the Mn2+ ions were introduced into the CsPbBr3/Cs4PbBr6 crystals by adding MnBr2 during the one-step slow cooling process. The prepared CsPbBr3/Cs4PbBr6:Mn2+ crystal with a size of 2.0 × 2.0 mm is shown in the inset of Fig. 4a displays a yellow-green color in daylight and green color under UV light (λex = 395 nm), respectively. Fig. 4a shows the comparison of the PL spectra of the CsPbBr3/Cs4PbBr6 crystals with and without Mn2+ doping. The PL peak of the CsPbBr3/Cs4PbBr6:Mn2+ crystals is blue-shifted by about 7 nm compared with the as-grown CsPbBr3/Cs4PbBr6 crystals, without showing a new PL peak. This phenomenon is contradictory to that of the Mn2+-doped CsPbBr3 system with a higher content of Mn2+ doping,41 while exhibiting a similar blue-shifted PL peak with lower content of Mn2+ doping.31,41 As described in Fig. 2a–c, for the as-grown CsPbBr3/Cs4PbBr6 crystals, the green PL emission comes from the CsPbBr3 phase. Doping of Mn2+ in the CsPbBr3/Cs4PbBr6 crystals alters the PL peak as shown in Fig. 4a, which indicates that the Mn2+ ions were successfully doped into the CsPbBr3 phase. The PLQY was 65.43% (Fig. S5, ESI†), showing a large improvement compared with the CsPbBr3/Cs4PbBr6 crystals (46.02%). This is also consistent with the PL spectra shown in Fig. 4a, indicating that Mn2+ doping can enhance the PL performance. The improved PL performance from doping the Mn2+ ions into the CsPbBr3/Cs4PbBr6 crystals may be attributed to Mn2+ possibly passivating the surface or grain boundary defects of the Mn-doped CsPbBr3/Cs4PbBr6 crystals.41,42 To further confirm the success of Mn2+ doping, the crystal was characterized using powder XRD measurement. The comparison of the powder XRD spectroscopy of the CsPbBr3/Cs4PbBr6 and CsPbBr3/Cs4PbBr6:Mn2+ crystals are shown in Fig. 4b. It can be seen that the powder XRD pattern of the CsPbBr3/Cs4PbBr6:Mn2+ crystals slightly shifts to a higher angle direction (∼0.04 degrees) compared to that of the CsPbBr3/Cs4PbBr6 crystals, which may be caused by the lattice contraction due to the replacement of the larger Pb2+ with the relatively smaller Mn2+.36 However, the observed shift in the angle is rather small, which may be due to the fact that it is more difficult to dope ions into those 3D–0D type perovskites. Thus, X-ray spectroscopy was applied to gain more insight into the incorporation of Mn2+ ions into the CsPbBr3/Cs4PbBr6 crystals during doping via high-angle annular dark-field scanning transmission microscopy (HAADF-STEM) equipped with EDS element mapping. The corresponding results are shown in Fig. 4c. From Fig. 4c, the Mn2+ ions are homogeneously distributed among the particles, providing solid evidence verifying the successful doping of Mn2+ ions into the CsPbBr3 phase. The content distribution of Mn elements in Fig. S8 and Table S1 (ESI†) shows the relatively small Mn-doping ratio, which is consistent with the rather small shifted angle observed in the XRD patterns and the undetectable new excitonic emission in the PL spectrum.
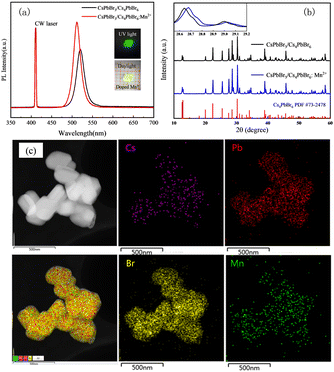 |
| Fig. 4 (a) The PL spectra of CsPbBr3/Cs4PbBr6 and CsPbBr3/Cs4PbBr6:Mn2+ crystals (irradiated by 410 nm continuous laser (CW) laser). The inset shows the dimensions of the Mn-doped CsPbBr3/Cs4PbBr6 crystals under UV light (395 nm) and daylight. (b) XRD patterns of the Mn-doped and undoped CsPbBr3/Cs4PbBr6 crystals. The inset is the enlarged XRD patterns. (c) High-angle annular dark-field scanning transmission microscopy image and EDS elemental mapping (Br, Cs, Pb and Mn) of the particles from the CsPbBr3/Cs4PbBr6:Mn2+ crystals by ball-milling. | |
Consequently, from the above results, it can be confirmed that the millimeter-scale Mn-doped CsPbBr3/Cs4PbBr6 crystals can be easily obtained via one-step slow cooling. In the following section, we will investigate the impact of doping Mn2+ ions on the CsPbBr3/Cs4PbBr6 crystals.
3.2. The effect of Mn2+ ions on the PL dynamics of the CsPbBr3/Cs4PbBr6 crystals
To investigate the change of the photophysical properties upon doping with Mn2+, the time-resolved PL decay of the CsPbBr3/Cs4PbBr6 crystals with and without doping Mn2+ ions was carried out at different temperatures under pulsed 365 nm excitation. Fig. 5a and b show the experimental and fit curves of the PL decays of both crystals at various temperatures, ranging from 80 to 300 K. All of the measured PL decay traces can be well fitted. |  | (1) |
where A1 and τ1 refer to the fast component of the decay and the corresponding amplitude, respectively. A2 and τ2 refer to the slow component of the decay and the corresponding amplitude, respectively. The fast component τ1 is typically attributed to trap-mediated nonradiative recombination, and the slow component τ2 is assigned to the lifetime of radiative recombination.43,44 The PL lifetimes (τ) and the corresponding amplitudes (A) as a function of temperature are plotted in Fig. 5c–f. For both CsPbBr3/Cs4PbBr6 and CsPbBr3/Cs4PbBr6:Mn2+ crystals, both τ1 and τ2 vary with temperature and have nearly the same trend. Changing the temperature has a significant influence on τ2 (varying from 5.5 to 39.9 ns for the CsPbBr3/Cs4PbBr6 crystals, and from 3.35 to 35.1 ns for the CsPbBr3/Cs4PbBr6:Mn2+ crystals), while the fast component τ2 remains almost consistent (varying from 2.3 to 7.2 ns for the CsPbBr3/Cs4PbBr6 crystals, and from 1.6 to 6.5 ns for the CsPbBr3/Cs4PbBr6:Mn2+ crystals). For the CsPbBr3/Cs4PbBr6 crystals, the proportion of the trap-mediated nonradiative recombination (A1) as a function of temperature is nearly in line with the temperature-dependent integrated PL emission intensity reported by M. Chen et al.45 There exists some difference between the A1/A2 ratios of the Mn-doped and undoped crystals. At 300 K, the ratios of A1/A2 equal 1.5 for both Mn-doped and undoped crystals, indicating that the effect of doping Mn2+ ions on the relaxation rates of trap-mediated recombination and radiative recombination is negligible, even though the PL lifetime of the CsPbBr3/Cs4PbBr6:Mn2+ crystals is shorter than that of the CsPbBr3/Cs4PbBr6 crystals. However, at 80 K, the ratios of A1/A2 become 1.6 and 3.1 for the Mn-doped and undoped crystals, respectively, indicating that doping Mn2+ ions can enhance the proportion of radiative recombination. At low temperatures, the lack of thermal excitation leads to the trapped carriers decaying through nonradiative pathways.46 Meanwhile, doping Mn2+ ions can effectively accelerate the decay of a dark exciton due to the magnetic mixing of bright and dark excitons.47 Thus, to some extent, it also can improve the proportion of radiative recombination. Therefore, doping Mn2+ ions has little influence on the ratio of radiative and nonradiative recombination at the temperature range from 180 to 300 K, while it enhances the proportion of radiative recombination at low temperatures (below 130 K).
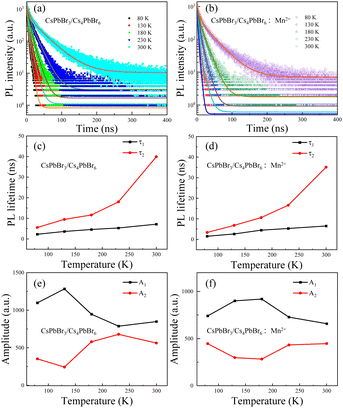 |
| Fig. 5 (a) and (b) Time-resolved PL decay of millimeter-scale CsPbBr3/Cs4PbBr6 and CsPbBr3/Cs4PbBr6:Mn2+ crystals at different temperatures, respectively. (c) and (d) The corresponding PL lifetimes in panels (a) and (b) as functions of temperature. (e) and (f) The corresponding amplitudes in panels (a) and (b) as functions of temperature. | |
3.3. Stability of the Mn2+ doped and undoped CsPbBr3/Cs4PbBr6 crystals
The stability of the perovskite materials, especially the thermal stability, hinders their practical applications. Intuitively, the observed luminescence (under 395 nm UV irradiation) of the as-grown CsPbBr3/Cs4PbBr6 crystals stored in a sealed centrifuge tube under an air atmosphere almost remains the same after one year (see Fig. S2, ESI†), indicating that the millimeter-scale CsPbBr3/Cs4PbBr6 crystals show high air stability. In order to further quantitatively confirm the long-term air stability, the PLQYs were obtained for CsPbBr3/Cs4PbBr6 crystals with and without doping Mn2+ that had been directly placed under an air atmosphere for three months. The results show that the PLQY of the undoped crystals was 42.95% (Fig. S6, ESI†), while that of the Mn-doped crystals was 61.49% (Fig. S7, ESI†). Compared with the just-prepared undoped and doped crystals (the PLQYs are 46.02 and 65.43%, respectively, for the undoped and doped crystals), doping Mn ions can slightly enhance the air stability, even though both crystals show long-term stability under air atmosphere. Those results further indicate that the introduction of Mn2+ can enhance the luminescence of the crystals and improves the air stability of the crystal.
Moreover, the thermal stability, moisture stability and photostability of the Mn-doped and undoped CsPbBr3/Cs4PbBr6 crystals were carried out. Fig. 6a shows the intensities of the PL peak of the Mn-doped and undoped CsPbBr3/Cs4PbBr6 crystals as functions of temperature, ranging from 300 to 400 K, respectively. The corresponding detailed PL spectra are shown in Fig. S9 (ESI†). It is clearly seen that upon increasing the temperature, the PL intensity of both Mn2+ doped and undoped crystals was significantly quenched. However, in comparison with the undoped crystals, the decrease in the intensities of the PL peak of the Mn-doped crystals was slightly slower. For instance, at 350 K, the PL intensity of the CsPbBr3/Cs4PbBr6:Mn2+ crystals remained at around 25% of the initial intensity, while that of the undoped crystals only remained at 20% of the initial intensity. These results indicate that the Mn-doped CsPbBr3/Cs4PbBr6 crystals exhibit relatively higher thermal stability than the pure CsPbBr3/Cs4PbBr6 crystals.
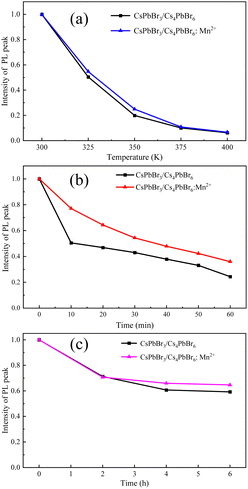 |
| Fig. 6 (a) Comparison of the PL peaks of the temperature-dependent PL spectra of the Mn2+ doped and undoped CsPbBr3/Cs4PbBr6 crystals. (b) Intensities of the PL peaks as a function of time of the Mn2+ doped and undoped CsPbBr3/Cs4PbBr6 crystals under a humidity of 70%. (c) Comparison of the PL peaks of the Mn2+ doped and undoped CsPbBr3/Cs4PbBr6 crystals under 455 nm laser irradiation with a power density of 5 W cm−2. | |
Fig. 6b shows the intensities of the PL peak of the CsPbBr3/Cs4PbBr6 crystals and Mn-doped CsPbBr3/Cs4PbBr6 crystals at a humidity of 70% in the time range of 0 to 60 min. The corresponding detailed PL spectra are also shown in Fig. S10 (ESI†). The PL intensity of both Mn2+ doped and undoped crystals decreases under a humid condition. However, compared with undoped crystals, the PL peak intensity of the Mn-doped crystals decreases much more slowly. After 10 min, the PL intensity of the CsPbBr3/Cs4PbBr6:Mn2+ crystals remains at about 77% of the initial intensity, while that of the undoped crystals is only maintained at 50%. In addition, the PL intensity of the Mn-doped crystals still remains at 36% of the initial intensity after 60 min, while that of the undoped crystals decreased to 24% of the initial intensity. Those results show that the Mn-doped CsPbBr3/Cs4PbBr6 crystals exhibited higher humidity stability than the pure CsPbBr3/Cs4PbBr6 crystals.
Next, we examined the photostability of the as-grown crystals by applying a 455 nm laser irradiation with a power density of 5 W cm−2. Fig. 6c shows the variations of intensity of the PL peak of both crystals under laser irradiation, and the corresponding specific PL spectra are shown in Fig. S11 (ESI†). It is clear that the PL intensities of the Mn2+ doped and undoped crystals decrease slowly with increasing illumination time. After two hours, the PL intensity of both samples decreased to about 70% of the initial intensity. Upon increasing the laser irradiation time, the intensity of the PL peak of the Mn-doped crystals decreased relatively slowly. After 6 hours, the PL intensity of the CsPbBr3/Cs4PbBr6:Mn2+ crystals remained at about 64% of the initial intensity, while that of the undoped crystals stayed at 60% of the initial intensity. Therefore, the Mn-doped CsPbBr3/Cs4PbBr6 crystals exhibit relative better photostability than the pure CsPbBr3/Cs4PbBr6 crystals.
The enhanced stability of the Mn-doped CsPbBr3/Cs4PbBr6 crystals can be mainly due to the Mn2+ ions replacing a portion of the Pb2+ ions, and results in an increase of the absolute value of the formation energy of each Pb2+ (or Mn2+).31,48 We note that the content of Mn2+ in our paper is smaller than the 2.08 mol% value mentioned in ref. 31. Furthermore, the substitution of the Pb2+ ion (ionic radius of Pb2+ is 1.33 Å) with the smaller Mn2+ ion (ionic radius of Mn2+ is 0.97 Å) in the lattices of the CsPbBr3/Cs4PbBr6 crystals might induce lattice contraction and improve the stability. This phenomenon was also found in the previous literature.31 Accordingly, those results suggest that doping Mn ions into the CsPbBr3/Cs4PbBr6 crystals can effectively enhance the luminescence performance and stability.
3.4. Applications
Saturated green PL emission with good thermal stability makes the Mn-doped and undoped CsPbBr3/Cs4PbBr6 crystals a promising candidate for display backlighting. The packaging experiments were carried out by combining the green emission Mn-doped and undoped CsPbBr3/Cs4PbBr6 crystals with blue-emitting GaN chips to fabricate green LEDs. Fig. 7a shows the device configuration of the green LED. Fig. 7b presents the EL spectra of the CsPbBr3/Cs4PbBr6 and CsPbBr3/Cs4PbBr6:Mn2+ crystals obtained at a current of 60 mA. The insets of Fig. 7b are the corresponding pictures of the green LED. Fig. 7c shows the CIE 1931 chromaticity diagram of the CsPbBr3/Cs4PbBr6 crystals and CsPbBr3/Cs4PbBr6:Mn2+ crystals. The color coordinates of the CsPbBr3/Cs4PbBr6 and CsPbBr3/Cs4PbBr6:Mn2+ crystals are (0.0949, 0.6917) and (0.0697, 0.7114), respectively. From the color coordinates, it can be seen that the crystals doped with Mn2+ have a further shift, which is identical to that for the previous PL spectra. Fig. S12 (ESI†) further proves that the introduction of different amounts of Mn2+ ions can finely adjust the PL of the CsPbBr3/Cs4PbBr6 crystals, which is promising for achieving applications in finely-tunable high color quality display backlighting.
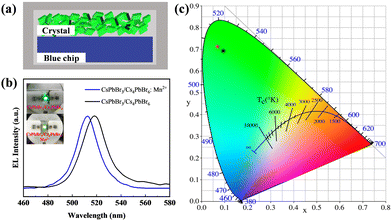 |
| Fig. 7 (a) Schematic diagram of the configuration of the prototype device. (b) EL spectra of the prototype green LED devices driven at a current of 60 mA. (c) CIE color coordinates of the green LED for the CsPbBr3/Cs4PbBr6 crystals (black spots) and Mn-doped CsPbBr3/Cs4PbBr6 crystals (red spots). | |
4 Conclusions
In summary, we successfully synthesized millimeter-scale Mn-doped and undoped CsPbBr3/Cs4PbBr6 crystals by the one-step slow cooling method. The effect of doping Mn2+ ions into the millimeter-scale CsPbBr3/Cs4PbBr6 crystals on the PL dynamics and stability were investigated. Compared with undoped crystals, the existence of Mn2+ ions blue-shifted the PL peak, although there was no new excitonic emission and the narrow FWHM of the PL spectra was retained. Doping Mn2+ ions can significantly enhance the proportion of radiative recombination at low temperature, while has little influence on the ratios of radiative and nonradiative recombination at the temperatures ranging from 180 to 300 K. In the presence of Mn2+ ions, the stability of the prepared millimeter-scale crystals can also be improved. Moreover, green LED devices packaged with both crystals were explored, and were promising for further display backlighting applications. Consequently, those results indicate that the addition of Mn2+ ions into millimeter-scale CsPbBr3/Cs4PbBr6 crystals provides effective strategies to improve the optoelectronic performance and stability. We hope that the large size crystals could also be a promising candidate for investigations of photophysical processes dynamics and optoelectronics practical applications.
Conflicts of interest
There are no conflicts to declare.
Acknowledgements
This work was supported by the National Key R&D Program of China (Grant No. 2021YFB3501700), the National Natural Science Foundation of China (Grant No. 12104311, 12004239), the Shanghai Chenguang Program (Grant No. 22CGA74), Shanghai Science and Technology Committee (STCSM) Science and Technology Innovation Program (Grant No. 22N21900400, 23N21900100), and the Science and Technology Talent Development Fund for Young and Middle-aged Teachers of Shanghai Institute of Technology (Grant No. ZQ2022-3).
References
- D. Yang, Y. Zou, P. Li, Q. Liu, L. Wu, H. Hu, Y. Xu, B. Sun, Q. Zhang and S. Lee, Nano Energy, 2018, 47, 235–242 CrossRef.
- A. Zhao, J. Zhang, Y. Di, Y. Guo, X. Shan, W. Zhou and Z. Gan, J. Alloys Compd., 2020, 830, 154731 CrossRef.
- L. Protesescu, S. Yakunin, M. I. Bodnarchuk, F. Krieg, R. Caputo, C. H. Hendon, R. X. Yang, A. Walsh and M. V. Kovalenko, Nano Lett., 2015, 15, 3692–3696 CrossRef PubMed.
- K. Lin, C. Yan, R. P. Sabatini, W. Feng, J. Lu, K. Liu, D. Ma, Y. Shen, Y. Zhao, M. Li, C. Tian, L. Xie, E. H. Sargent and Z. Wei, Adv. Funct. Mater., 2022, 32, 2200350 CrossRef.
- Y. Yuan, G. Yan, R. Hong, Z. Liang and T. Kirchartz, Adv. Mater., 2022, 34, 2108132 CrossRef CAS PubMed.
- V. K. Ravi, G. B. Markad and A. Nag, ACS Energy Lett., 2016, 1, 665–671 CrossRef CAS.
- F. Zhang, H. Zhong, C. Chen, X. Wu, X. Hu, H. Huang, J. Han, B. Zou and Y. Dong, ACS Nano, 2015, 9, 4533–4542 CrossRef CAS PubMed.
- J. Song, J. Li, X. Li, L. Xu, Y. Dong and H. Zeng, Adv. Mater., 2015, 27, 7162–7167 CrossRef CAS PubMed.
- Y. Wang, X. Li, J. Song, L. Xiao, H. Zeng and H. Sun, Adv. Mater., 2015, 27, 7101–7108 CrossRef CAS PubMed.
- Z. Li, L. Kong, S. Huang and L. Li, Angew. Chem., Int. Ed., 2017, 56, 8134–8138 CrossRef CAS PubMed.
- S. Kumar, J. Jagielski, N. Kallikounis, Y. Kim, C. Wolf, F. Jenny, T. Tian, C. J. Hofer, Y. Chiu, W. J. Stark, T. Lee and C. Shih, Nano Lett., 2017, 17, 5277–5284 CrossRef CAS PubMed.
- P. Qin, S. Tanaka, S. Ito, N. Tetreault, K. Manabe, H. Nishino, M. K. Nazeeruddin and M. Grätzel, Nat. Commun., 2014, 5, 3834 CrossRef CAS PubMed.
- N. J. Jeon, J. H. Noh, W. S. Yang, Y. C. Kim, S. Ryu, J. Seo and S. I. Seok, Nature, 2015, 517, 476–480 CrossRef CAS PubMed.
- P. Docampo, J. M. Ball, M. Darwich, G. E. Eperon and H. J. Snaith, Nat. Commun., 2013, 4, 2761 CrossRef PubMed.
- H. Kim, I. Mora-Sero, V. Gonzalez-Pedro, F. Fabregat-Santiago, E. J. Juarez-Perez, N. Park and J. Bisquert, Nat. Commun., 2013, 4, 2242 CrossRef PubMed.
- D. Liu, J. Yang and T. L. Kelly, J. Am. Chem. Soc., 2014, 136, 17116–17122 CrossRef CAS PubMed.
- J. J. Choi, X. Yang, Z. M. Norman, S. J. L. Billinge and J. S. Owen, Nano Lett., 2014, 14, 127–133 CrossRef CAS PubMed.
- J. A. Christians, R. C. M. Fung and P. V. Kamat, J. Am. Chem. Soc., 2014, 136, 758–764 CrossRef CAS PubMed.
- G. Xing, N. Mathews, S. S. Lim, N. Yantara, X. Liu, D. Sabba, M. Grätzel, S. Mhaisalkar and T. C. Sum, Nat. Mater., 2014, 13, 476–480 CrossRef CAS PubMed.
- S. Cheng, Z. Qiao, Z. Wang, L. Xiao, S. Das, Y. T. Thung, Z. Yuan, V. D. Ta, W. Fan, Y. Chen and H. Sun, Adv. Opt. Mater., 2023, 11, 2203133 CrossRef CAS.
- L. Dou, Y. Yang, J. You, Z. Hong, W. Chang, G. Li and Y. Yang, Nat. Commun., 2019, 10, 1866 CrossRef PubMed.
- Y. Chen, J. S. Manser and P. V. Kamat, J. Am. Chem. Soc., 2015, 137, 974–981 CrossRef CAS.
- H. B. Cho, J. W. Min, H. J. Kim, N. S. M. Viswanath, T. Samanta, J. H. Han, Y. M. Park, S. W. Jang and W. B. Im, ACS Appl. Electron. Mater., 2023, 5, 66–76 CrossRef CAS.
- L. Wang, H. Liu, Y. Zhang and O. F. Mohammed, ACS Energy Lett., 2020, 5, 87–99 CrossRef CAS.
- C. Wang, L. Yan, J. Si, T. Huo and X. Hou, J. Alloys Compd., 2023, 946, 169272 CrossRef CAS.
- Y. Wang, D. Yu, Z. Wang, X. Li, X. Chen, V. Nalla, H. Zeng and H. Sun, Small, 2017, 13, 1701587 CrossRef.
- X. Chen, F. Zhang, Y. Ge, L. Shi, S. Huang, J. Tang, Z. Lv, L. Zhang, B. Zou and H. Zhong, Adv. Funct. Mater., 2018, 28, 1706567 CrossRef.
- M. I. Saidaminov, O. F. Mohammed and O. M. Bakr, ACS Energy Lett., 2017, 2, 889–896 CrossRef CAS.
- L. N. Quan, R. Quintero-Bermudez, O. Voznyy, G. Walters, A. Jain, J. Z. Fan, X. Zheng, Z. Yang and E. H. Sargent, Adv. Mater., 2017, 29, 1605945 CrossRef PubMed.
- H. Nishimura, K. Enomoto, Y. Pu and D. Kim, RSC Adv., 2022, 12, 6255–6264 RSC.
- S. Zou, Y. Liu, J. Li, C. Liu, R. Feng, F. Jiang, Y. Li, J. Song, H. Zeng, M. Hong and X. Chen, J. Am. Chem. Soc., 2017, 139, 11443–11450 CrossRef CAS PubMed.
- G. Pan, X. Bai, D. Yang, X. Chen, P. Jing, S. Qu, L. Zhang, D. Zhou, J. Zhu, W. Xu, B. Dong and H. Song, Nano Lett., 2017, 17, 8005–8011 CrossRef.
- T. Xuan, S. Guo, W. Bai, T. Zhou, L. Wang and R. Xie, Nano Energy, 2022, 95, 107003 CrossRef.
- C. Chen, T. Xuan, W. Bai, T. Zhou, F. Huang, A. Xie, L. Wang and R. Xie, Nano Energy, 2021, 85, 106033 CrossRef.
- S. Cheng, Y. Chen and H. Zhong, Cryst. Growth Des., 2022, 22, 4025–4030 CrossRef.
- C. Lin, T. Liu, S. Lin, K. M. Boopathi, C. Chiang, W. Tzeng, W. C. Chien, H. Hsu, C. Luo, H. Tsai, H. Chen, P. Kuo, J. Shiue, J. Chiou, W. Pong, C. Chen and C. Chen, J. Am. Chem. Soc., 2022, 144, 15718–15726 CrossRef.
- Z. Zhang, Y. Zhu, W. Wang, W. Zheng, R. Lin, X. Li, H. Zhang, D. Zhong and F. Huang, Cryst. Growth Des., 2018, 18, 6393–6398 CrossRef CAS.
- J. Yin, Y. Zhang, A. Bruno, C. Soci, O. M. Bakr, J. Brédas and O. F. Mohammed, ACS Energy Lett., 2017, 2, 2805–2811 CrossRef CAS.
- J. Zhang, C. Liu, S. Wu, Y. Di, F. Xing, Z. Ren, X. Zhang, L. Yang, C. Ma and Z. Gan, J. Phys. Chem. C, 2022, 126, 8777–8786 CrossRef CAS.
- S. Wang, Y. Xu, R. Chen, M. Zhu, M. Wang, M. Cao, Y. Liu, H. Ding, S. Zhang, J. Bai, J. Ren, T. Xuan and H. Li, Coatings, 2022, 12, 512 CrossRef CAS.
- L. Wang, H. Mao, J. Li, Y. Li, M. Li, J. Zhu, B. Fan, W. Liu, G. Shao, H. Xu, H. Wang, R. Zhang and H. Lu, Nanotechnology, 2022, 33, 455703 CrossRef.
- D. Parobek, Y. Dong, T. Qiao and D. H. Son, Chem. Mater., 2018, 30, 2939–2944 CrossRef CAS.
- D. Shi, V. Adinolfi, R. Comin, M. Yuan, E. Alarousu, A. Buin, Y. Chen, S. Hoogland, A. Rothenberger, K. Katsiev, Y. Losovyj, X. Zhang, P. A. Dowben, O. F. Mohammed, E. H. Sargent and O. M. Bakr, Science, 2015, 347, 519–522 CrossRef CAS.
- E. V. Péan, S. Dimitrov, C. S. De Castro and M. L. Davies, Phys. Chem. Chem. Phys., 2020, 22, 28345–28358 RSC.
- M. Chen, S. Yang, Y. Yuan, X. Shen, Y. Liu, Q. Wang, D. Cao and C. Xu, J. Phys. Chem. C, 2021, 125, 11278–11284 CrossRef CAS.
- Y. Ling, L. Tan, X. Wang, Y. Zhou, Y. Xin, B. Ma, K. Hanson and H. Gao, J. Phys. Chem. Lett., 2017, 8, 3266–3271 CrossRef CAS.
- T. Qiao, X. Liu, D. Rossi, M. Khurana, Y. Lin, J. Wen, J. Cheon, A. V. Akimov and D. H. Son, Nano Lett., 2021, 21, 9543–9550 CrossRef CAS.
- Z. Li, L. Li, H. Liu, F. Li, J. Zhao and Y. Wang, New J. Chem., 2020, 44, 2980 RSC.
|
This journal is © the Owner Societies 2024 |