DOI:
10.1039/D2BM01493A
(Paper)
Biomater. Sci., 2023,
11, 288-297
Biodegradable hydrogels with photodynamic antibacterial activity promote wound healing and mitigate scar formation†
Received
14th September 2022
, Accepted 2nd November 2022
First published on 9th November 2022
Abstract
Bacterial proliferation and the disordered extracellular matrix (ECM) at the wound site are the major reasons for delayed healing and abnormal scarring. The development of new multifunctional dressing materials that can effectively prevent scar formation without delaying wound healing remains a challenge. In this study, we construct a verteporfin-loaded biodegradable hydrogel (VP-gel) using hyaluronic acid and thiol-terminated 4-arm polyethylene glycol (PEG). The injectable VP-gel sustainably releases small doses of verteporfin in the wound microenvironment that generates reactive oxygen species (ROS) under red light irradiation to kill bacteria efficiently. Importantly, the sustained release of VP could also regulate TGF-β family-induced cellular responses and the downstream signaling molecule Smad2 in fibroblasts to reduce myofibroblast differentiation, promoting ECM reconstruction and scarless wound healing. Immunohistochemical examination of wound healing and histomorphology in a mouse full-thickness wound model demonstrates excellent acceleration effects of VP-gel for infected wound healing. Therefore, VP-gel with anti-scarring and antibacterial activity, as well as enhanced infection wound healing ability shows great potential in the clinical treatment of scar healing for infected wounds.
1. Introduction
Wound healing is a dynamic, complex and multicellular process aimed at rebuilding the skin's barrier function.1 Bacterial proliferation and the disordered extracellular matrix (ECM) at the wound site are the major reasons for delayed healing and excess scar formation.2,3 Unlike normal skin, scar tissue is characterized by hyperproliferation of fibroblasts and excess deposition of collagen. Scar tissue has a dense, parallel fibrous extracellular matrix that makes it more fragile than normal tissue, leading to growth restriction and permanent loss of function.4 Many drugs such as corticosteroids,5 bleomycin,6 interferon,7 verapamil,8etc. have been reported to alleviate scarring. However, as wound healing is a complex and dynamic process with risks of infection and limitations of existing treatment strategies, scar-free wound repair remains a challenge.9
The ECM is a major constituent of the skin layer and is mainly comprised of collagens, fibronectin, hyaluronic acid (HA), etc.10 Fibroblasts are the most important cells for remodeling the ECM and maintaining the skin integrity.11 During wound healing, TGF-β1 plays a major role in converting fibroblasts into myofibroblasts to facilitate wound closure, but excessive TGF-β1 expression leads to uncontrolled cell proliferation and the development of abnormal scar tissue.12 Methods that promote wound closure while inhibiting excessive cell proliferation, therefore, are the key to scar-free wound healing.
Verteporfin (VP) is a photosensitizer that produces singlet oxygen under 680 nm light irradiation and has been clinically approved for the treatment of acute macular degeneration.13 Two key downstream transcription coactivators in the Hippo pathway, Yes-associated protein (YAP) and transcriptional coactivator with PDZ-binding motif, can modulate the wound healing process by regulating the activity of TGF-β1 and TGF-β1-related signaling factors such as the Smad2 protein.14 As an inhibitor of the YAP pathway, the antitumor and anti-inflammatory effects of VP have been demonstrated by a number of studies.15–17 Recently, VP has been reported to promote wound regeneration and scar-reducing, possibly due to the prevention of increased TGF-β1 activity leading to the overproduction of fibroblasts and overdeposition of collagen.4,18 However, high concentrations of VP inhibit cell proliferation and delay wound healing. Therefore, we hypothesize that wound dressings with sustained release of low-concentration VP might inhibit scar formation without delaying wound closure. Meanwhile, VP can generate singlet oxygen under red light irradiation to eliminate bacteria and promote wound healing.
Materials of natural origin with similar properties to the extracellular matrix (ECM) are often used in tissue engineering.19 Wound dressings play an important role in the skin healing process, especially for traumatic, chronic, and thermal wounds.20,21 Conventional wound dressings, such as gauze, bandages and cotton wool, do not prevent wound beds from drying and bacterial infection and cause repeated injury when removed, which often results in damage to the regenerating skin tissue.22 Compared to traditional dressings, hydrogels can cool the surface of the wound to relieve pain, maintain a moist wound environment, and absorb tissue exudate.23 More importantly, hydrogels are easily removed from wounds without tissue adhesion.24 In addition, porous networks within the hydrogel enable fibroblast proliferation and keratinocyte migration, which are necessary for complete epithelialization and healing of the wound.22 Therefore, encapsulating VP in hydrogels with good biocompatibility, degradability and tissue repair function for the sustained release of VP would provide a novel strategy for wound healing without scarring.
In this work, VP-gels were prepared through a Michael addition reaction based on a hyaluronic acid-containing methacrylate anhydride unit and 4-arm-PEG-SH coloaded with VP. The VP-gel can generate singlet oxygen under red light irradiation to improve the bactericidal effect while inhibiting scar formation by directing Smad2 activity to regulate the TGF-β family-induced cellular responses. Methicillin-resistant Staphylococcus aureus (MRSA) was selected as the model bacterium, and the synergistic antibacterial effect of red light irradiation was extensively evaluated by agar plate tests, scanning electron microscopy (SEM) morphology changes and a MRSA-infected mouse model. In vitro results clearly show excellent synergistic antimicrobial and anti-scarring properties with improved wound healing performance compared to conventional dressing materials. Furthermore, the results of wound closure, histopathological examinations, biochemical analysis and immunohistochemistry were evaluated in vivo to investigate the therapeutic effects of the hydrogel dressing in a full-thickness skin wound model (Fig. 1).
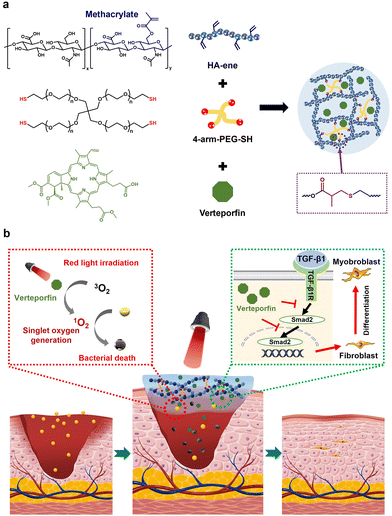 |
| Fig. 1 (a) Schematic illustration showing the synthesis of VP-gel. (b) VP-gel sustainably released VP in the wound microenvironment and promoted wound healing without scar formation by inhibiting the TGF-β pathway and providing antibacterial effects under red light irradiation. | |
2. Experimental section
2.1. Materials
Hyaluronic acid (Shanghai Acmec Biochemical Co. Ltd), 4-arm-PEG-SH (Psnsure Biological), verteporfin (Beijing OKA Biological Technology Co. Ltd), methacrylic anhydride (Energy Chemical), NaOH (Aladdin), DMEM medium (Hyclone), 3T3 cell line (ATCG), calcein/PI cell viability/cytotoxicity assay kit (Beyotime), FBS (Sigma), and hyaluronidase (Shanghai Yuanye Bio-Technology Co. Ltd), FBS (Sigma)
2.2. Animals
Balb/c mice (female, 6 to 8 weeks) were purchased from the Shanghai Slack Laboratory Animal Co. Ltd. All animal procedures were performed in accordance with the Guidelines for Care and Use of Laboratory Animals of the University of Science and Technology of China and approved by the Animal Ethics Committee of the university (USTCACUC192201049).
2.3. Preparation and characterization of VP-gel
2.3.1. Synthesis of methacrylic-functionalized HA.
The methacrylated HA was synthesized according to a previously described method.25 Briefly, sodium hyaluronate (1 g) was dissolved in deionized water at a concentration of 1% w/v, and 1.11 ml methacrylic anhydride was added dropwise into the sodium hyaluronate solution with continuous stirring at 4 °C. pH value of the reaction solution was adjusted to 7.0–8.0 with 1 mol L−1 NaOH, and the solution was continuously stirred overnight. The methacrylated HA solution was precipitated using ethyl alcohol and was further purified via dialysis (molecular weight cut off 1 kDa) at 4 °C for 2 d. Finally, the dialyzed product was frozen at −80 °C and lyophilized to obtain methacrylated HA (HA-ene).
2.3.2. Preparation of hydrogels.
First, 4-arm-PEG-SH was dissolved in water to obtain a 10 wt% aqueous solution (solution 1), and HA-ene was dissolved in water to obtain a 2 wt% aqueous solution (solution 2). Then, 0.1 g, 0.2 g, and 0.3 g of solution 1 were added to 1 g of solution 2. After mixing for 10 min, HA-PEG1, HA-PEG2 and HA-PEG3 were obtained.
2.3.3. Rheological analysis of hydrogel.
The rheological behavior of the hydrogels was characterized with a rheometer (TA AR-G2, TA Instruments) equipped with 40 mm parallel plates. Frequency sweeps were performed at 25 °C at 0.1% strain, and the storage modulus (G′) and the loss modulus (G′′) were measured as a function of the angular frequency from 0.1 to 100 rad s−1.
2.3.4. Swelling and degradation test.
The swelling ratio of the hydrogels was tested by freeze-drying them in phosphate buffered saline (PBS, pH = 7.4) at 37 °C. At regular time intervals, the swollen samples were removed from the PBS and then immediately weighed after the surface solution was discarded using filter paper. The water uptake ability was calculated using the equation:
Swelling ratio (%) = [(Ws − Wd)/Wd] × 100% |
where Ws and Wd refer to the weight of the swollen hydrogel at the given time and the weight of the original freeze-dried hydrogel, respectively.
The initial weights of the completely gelled wet hydrogels were recorded and the samples were then submerged in PBS (0.01 M pH 7.4) containing hyaluronidase at a concentration of 30–50 U mL−1, and the residual mass of the samples in hyaluronidase were recorded at regular time intervals until the samples completely degraded. The degradation study was performed for three parallel samples. The degradation rate was calculated using the following equation:
Remaining weight (%) = Wt/Wi, |
where
Wi and
Wt represented the weight of the wet hydrogels at the initial and pre-set measurement time points, respectively.
2.3.5. ROS production of VP.
ROS production of VP was determined by the 1,3-diphenylisobenzofuran (DPBF) bleaching method. An ethanol solution of DPBF (40 μl, 2 mmol L−1) was added to the VP solution. The samples were continuously irradiated with 680 nm light at 0.4 W cm−2 power density for 10 min, and the absorbance of DPBF at 410 nm was collected every 2 min. As a control, the solution of DPBF without VP was measured under light irradiation.
2.4. Cytotoxicity studies
Cell viability was measured by the quantitative MTT cytotoxicity assay. 3T3 mouse fibroblasts were suspended in cell culture medium, seeded into 96-well microculture plates at a density of 1 × 104 cells per 100 μl per well and incubated for 24 h at 37 °C in a 5% CO2 humidified incubator to obtain a monolayer of cells. Different concentrations of VP were added to the cell culture medium and further incubated for an additional time (12 h, 24 h, 36 h or 48 h). The sample solution was removed, and the cells were incubated with 100 μl of 1 mg ml−1 MTT in PBS for 2 h. Finally, the PBS solution was replaced with 150 μl of DMSO to dissolve the formazan, and the absorbance of the DMSO solution was detected at 570 nm (reference 650 nm). The relative cell viability was calculated as the ratio between the mean absorbance value of the sample and that of cells cultured in the medium. Samples with relative cell viability less than 80% were deemed to be cytotoxic. For each sample, 3 independent cultures were prepared, and the cytotoxicity test was repeated 3 times for each culture.
2.5. Cell viability determined by live/dead assay
Hydrogels were prepared in a 96-well plate with a volume of 100 μl and sterilized before use. Then, 3T3 cells were seeded in wells at a density of 5 × 103 cells per well. Dulbecco's modified Eagle's medium (DMEM, HyClone) with 10% (v/v) FBS, 100 U ml−1 penicillin, and 100 μg ml−1 streptomycin was added. Cells were incubated under a humidified atmosphere containing 5% CO2 at 37 °C. At predetermined times, the cells were stained with calcein-AM (2 μM) and propidium iodide (PI) (4.5 μM) and observed by fluorescence microscopy.
2.6. Hemolysis test
Fresh whole blood was acquired from mice using a vacuum blood collection tube containing heparin sodium, which was washed to obtain a red blood cell (RBC) suspension. Then, the RBCs were diluted with normal saline (volume ratio, 4/5). Then, 0.2 ml of the RBC suspension was mixed with different solutions, 1 ml of normal saline (blank control), distilled water (positive control), 2% HA, 10% PEG or hydrogels with varying concentrations of VP. The mixtures were incubated for 60 min at 37 °C. The supernatant was carefully removed and transferred to 96-well plates. The optical density (OD) was determined by spectroscopy at 576 nm. Three replicates were set for each sample. The hemolysis ratio was calculated using the equation hemolysis (%) = [OD value (sample) − OD value (saline)]/[OD value (water) − OD value (saline)] × 100%.
2.7.
In vitro drug release study
VP was encapsulated in situ into the hydrogel matrix to obtain VP-gel. After placing VP-gel in a phosphate buffer solution, the absorbance at 680 nm of the solution was measured after a certain period of time. According to the absorption value, the amount of VP released can be calculated so that the proportion of VP released can be obtained.
2.8. Inhibition zone test
MRSA was employed to evaluate the antibacterial effect of the sample hydrogels. MRSA incubated overnight were collected by centrifugation at 10
000 rpm for 5 min and rinsed twice with PBS. A certain quantity of bacteria was obtained by dilution according to the optical density at 600 nm (OD 0.5) using PBS. 100 μl of diluted bacterial suspension with a concentration of 106 CFU ml−1 was uniformly spread onto LB agar plates and then coated with 10 μl of hydrogels with varying concentrations of VP or solutions with varying concentrations of VP. The samples were continuously irradiated with 680 nm light at 0.4 W cm−2 power density for 10 min. Colony formation was observed, and the diameter of the inhibition zones was measured after incubation at 37 °C for 24 h.
2.9. SEM characterization
The bacteria received from the above experiment were divided into several parts and further detected by SEM. Briefly, 1 ml of the resuspended bacterial suspension obtained above was centrifuged at 8000 rpm, and the bacterial pellets were washed with PBS three times. VP was added to the bacterial suspension (200 μl) and incubated at 37 °C for 15 min without light. Another 1 ml of the bacterial suspension underwent centrifugal washing three times and then was immobilized with 2.5% glutaraldehyde. After dehydration with graded ethanol and metal spraying, the bacteria were observed under SEM.
2.10.
In vivo antibacterial activity and wound healing properties
For the wound healing experiment, the mouse wound infection model was prepared as follows: all mice were acclimatized for 3 days and shaved a day before surgery. Mice with intraperitoneal injection of pentobarbital sodium (50 mg per kg body weight) were prepared first, and then 6 mm diameter full thickness skin wounds were created on the region above the tail and below the back. Finally, a bacterial suspension (20 μl) containing MRSA (106 CFU ml−1) was placed on the wounds. On the second day, 20 μl samples (8 μg ml−1 VP hydrogel, 8 μg ml−1 VP, 8 μg ml−1 vancomycin) were used to cover the wound, while untreated wounds were used as negative controls treated with 20 μl of PBS. For the light irradiation group, the wounds were continuously irradiated with 680 nm light at 0.4 W cm−2 power density for 10 min. The area of the wound was measured at preset times post treatment. The wound closure was calculated according to the following equation: wound closure (%) = (W0 − Wn)/W0 × 100%, where W0 and Wn indicate the wound area at day 0 and sampling times, respectively. Hematoxylin and eosin (H&E) and Masson's trichrome staining were used to evaluate the wound healing process. Skin samples were fixed in 10% formaldehyde solution, dehydrated, and embedded in paraffin. Tissues were then cut into sections of 5 μm thickness by microtome, which were stained with H&E or Masson's trichrome staining. The H&E staining protocol included sequential deparaffinization, alcohol pass, 0.5% hematoxylin staining for 10 min, 0.5% hydrochloric alcohol washing and 0.5% eosin staining for 10 min. Masson's trichrome staining was implemented according to a series of steps, including deparaffinization, alcohol pass, and staining by Masson blue, reichun/magenta and aniline blue.
3. Results and discussion
3.1. Preparation and characterization of VP-gel
Hyaluronic acid was selected as the hydrogel component because of its biocompatibility as a naturally occurring polysaccharide in the ECM.26 The ECM is a major constituent of the skin layer and provides structural support for cells, acting as a reservoir of growth factors that are rapidly mobilized to stimulate cell proliferation and migration upon injury during wound healing.9 The alcoholysis reaction between the hydroxyl groups of hyaluronic acid and methacrylate anhydride leads to the incorporation of methacrylate units on hyaluronic acid (HA-ene). Representative peaks at δ 5.7 and δ 6.2 from the 1H NMR spectrum indicated successful incorporation of methacrylic units onto hyaluronic acid (Fig. S1†). Since the sulfhydryl group can react with methacrylic groups by the Michael addition reaction, we used 4-arm PEG-SH as a crosslinking agent. PEG was selected due to its biocompatibility, minimal toxicity and immunogenicity, high solubility in water, and extensive use for numerous drug delivery and tissue engineering applications.27 Hydrogel formation was observed within 10 min of mixing the 4-arm PEG-SH and HA-ene solutions (Fig. 2a). By changing the amount of 4-arm PEG-SH, hydrogels with different degrees of cross-linking were synthesized. (The hyaluronic acid hydrogels prepared using 0.1, 0.2, and 0.3 g 10 wt% 4-arm PEG-SH solutions were denoted as HA-PEG1, HA-PEG2 and HA-PEG3, respectively.) To investigate the effect of crosslink density on the mechanical properties of hydrogels, rheological tests were performed on hydrogels with different ratios of 4-arm PEG-SH and HA-ene. In Fig. S2a–c,† the elastic modulus G′ of all three hydrogels was greater than the loss modulus G′′ for almost the full range of frequencies, indicating that the VP-hydrogel could maintain the gel state at room temperature. At the same time, we found that the elastic modulus G′ of the corresponding hydrogel gradually increased with the increase in 4-arm-PEG-SH, due to the increased cross-link density.
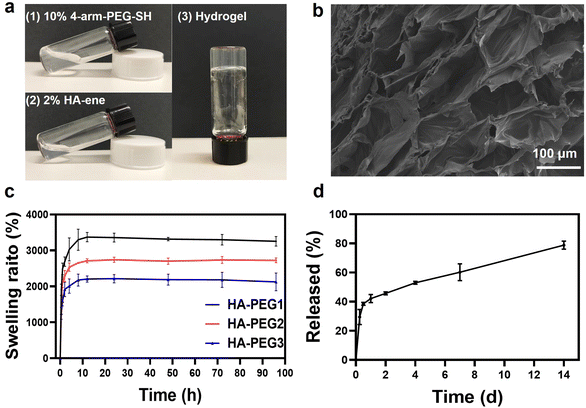 |
| Fig. 2 (a) Images of 4-arm-PEG-SH (a1) and HA-ene solutions (a2); HA-PEG2 hydrogel obtained from mixing above solutions or UV irradiation (a3). (b) SEM image of the HA-PEG2 hydrogel. (c) Swelling ratio of HA-PEG1, HA-PEG2, HA-PEG3 after immersion in PBS for 96 hours. (d) Time-dependent VP release of VP-gel in PBS over 14 days. | |
The microstructure morphologies of the lyophilized hydrogel were characterized via SEM (Fig. 2b), and a tridimensional hydrogel network with an average pore diameter of 100 μm was observed. The porous structure of the hydrogel provides space for the absorption of tissue exudates, hindering the spread of bacteria and limiting further infection. After the hydrogels reached their equilibrium swelling levels within 12 h, the wet weight remained relatively constant for 4 days. The HA-PEG1 hydrogel has the highest swelling property, indicating that the lower the degree of crosslinking, the higher the swelling rate. The water uptake ratio of all hydrogels in swelling equilibrium was above 1800% (Fig. 2c), indicating its favorable water absorption capacity, which is critical for a wound dressing to accelerate the wound healing process by removing wound exudate, providing a moist environment and reducing the probability of infection.28We have investigated the in vitro degradation kinetics of the hydrogels with hyaluronidase (HAse). Hyaluronidase is a ubiquitous enzyme in the body that degrades hyaluronic acid.29 At a concentration of 30–50 U mL−1 HAse, all hydrogels showed complete degradation in 11 to 13 days (Fig. S3†). The degradation rate that matches the wound healing process is an advantage of the HA hydrogel as a wound dressing.30
VP-loaded hydrogels (VP-gels) were synthesized by directly adding VP solutions into 2% HA and 10% PEG solutions. The release of VP was explored to elucidate the possibility of loading drugs into hydrogels with controlled release characteristics. As shown in Fig. 2d, VP-gel maintained a sustained release profile in phosphate-buffered saline (PBS) due to its porous structure and the diffusion of VP. At the initial stage of wound healing, only a small amount of VP is released, which can generate singlet oxygen under red-light irradiation to produce an antibacterial effect, promote wound healing, and inhibit scar formation.
3.2. Biocompatibility of VP-gel
Superior biocompatibility and excellent antibacterial properties are key criteria for material selection in the biomedical field.31–33 To assess the cytocompatibility of the hydrogel components, cell activity was tested after different incubation conditions for 24 h. As shown in Fig. 3a, cell viabilities were maintained above 85% even at high concentrations of 4-arm PEG-SH solution and HA-ene solution, indicating the low cytotoxicity of the hydrogel components. The biocompatibility of the hydrogel was further confirmed with calcein-AM/propidium iodide (PI) staining. Live cells showed green fluorescence, and PI-positive dead cells showed red fluorescence. Few obvious dead cells were observed via microscopy, indicating excellent biocompatibility of the hydrogel (Fig. 3c).
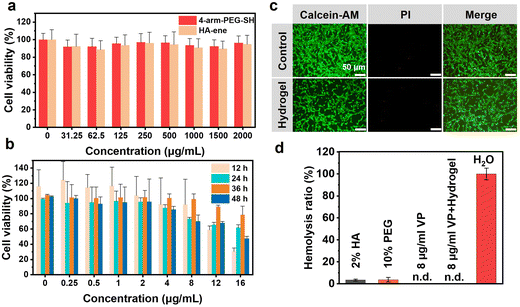 |
| Fig. 3 (a) Cell viability of 3T3 cells cultured with different concentrations of 2% HA and 10% 4-ARM-PEG solution. (b) Cell viability of 3T3 cells cultured with different concentrations of verteporfin. (c) Representative fluorescence images of calcein-AM/PI-stained 3T3 cells after treatment with or without hydrogels. (scale bars: 50 μm) (d) hemolytic properties of all the components of VP-gel (2% HA-ene, 10% 4-arm-PEG-SH, 8 μg ml−1 VP and VP-gel containing 8 μg ml−1 VP, solvents are PBS.) | |
To evaluate the cytotoxicity of VP, different concentrations of VP were incubated with 3T3 cells for 48 hours, and the cell growth morphology was observed under microscope every 12 hours. Cells in the control group without VP had grown from the original circular shape to a spindle shape with a few axons, and visible axons grew longer. Cells cultured with VP exhibited different morphologies. Cells incubated with high VP concentrations were round in shape and less differentiated, indicating that VP can inhibit the differentiation and proliferation of 3T3 cells (Fig. S5†). The MTT assay (Fig. 3b) showed that the cytotoxicity of VP was concentration dependent and cell viability was above 80% at 8 μg ml−1. Therefore, the VP loading in the hydrogel was 8 μg ml−1 in subsequent animal experiments.
The biocompatibility of the samples was also evaluated by hemolysis tests. All four groups (2% HA-ene, 10% 4-arm-PEG-SH, 8 μg ml−1 VP and VP-gel containing 8 μg ml−1 VP) presented light yellow, similar to the PBS control group, while the H2O group was bright red (Fig. S6†), indicating that all the components showed no hemolytic activity for erythrocytes compared to the positive H2O group. The hemolysis ratios of all the samples were lower than 5% (Fig. 3d). The above results demonstrated good biocompatibility of the hydrogel.
3.3. Antibacterial effect of VP-gel
Open, moist, and exuding wounds provide an ideal environment for bacterial colonization, a major factor that delays the wound healing process.34 To explore its capability as a wound dressing, we further investigated the in vitro antibacterial performance of the hydrogels. The hydrogels are placed directly on the surface of the bacterial culture medium, and the antibacterial activity is evaluated from the diameter of the inhibition zone, as shown in Fig. 4a. The VP solution and VP-gel have good antibacterial effects against Gram-positive bacteria (S. aureus and MRSA), which can be significantly enhanced by red light-triggered photodynamic therapy (PDT). With red light irradiation, inhibition zones were observed at all treatment sites in both VP solution and VP-gel groups. Without red light irradiation, the VP-gel group containing low concentration of VP had almost no bactericidal effect, while the VP solution group showed a bacteriostatic zone when the concentration exceeded 4 μg ml−1. These outcomes indicate that the hydrogel provided a sustained release of the photosensitizer VP whose antibacterial effect can be enhanced with light irradiation.
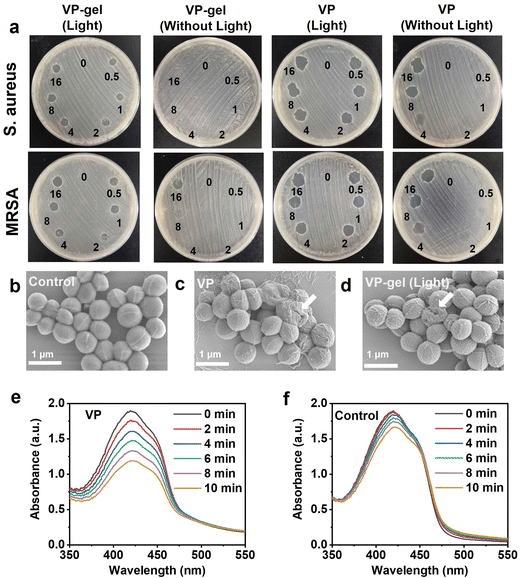 |
| Fig. 4 (a) Antibacterial activity of VP solution and VP-gel against S. aureus (top) and MRSA (bottom). The labelled numbers in plates indicated the VP concentration (μg mL−1). (b–d) SEM images of native MRSA (b) and treated MASA with VP solution (8 μg mL−1) (c) or VP-gel (8 μg mL−1) with light irradiation (d). (e and f) UV–vis absorption spectra of DPBF in the presence or absence of VP under red light irradiation at different time points. | |
To gain an insight into the antimicrobial mechanism of the hydrogel, the morphological changes of bacteria after contact with hydrogels and VP were investigated. As shown in Fig. 4b–d, the SEM images of the MRSA bacteria without treatment show a typical sphere-shaped structure with clear edges and smooth surfaces. In contrast, cellular deformation and structural collapse were observed in the VP and VP-gel treated bacteria. The above results confirmed that VP could destroy the structure of the bacterial membrane to kill bacteria. We also performed the antibacterial effect experiment of VP-gel on E. coli, and we found that the survival rate of Gram-negative bacteria was higher than that of Gram-positive bacteria after treatment with the same dose of VP-gel and red light (Fig. S8†), which should be attributed to the cell wall of Gram-negative bacteria formed by the lipopolysaccharide (LPS) in the outer membrane playing a vital role as a drug barrier. We also performed the antibacterial effect experiment of VP-gel on E. coli, and we found that the survival rate of Gram-negative bacteria was higher than that of Gram-positive bacteria after treatment with the same dose of VP-gel and red light (Fig.S8†), which should be attributed to the cell wall of Gram-negative bacteria formed by lipopolysaccharide (LPS) in the outer membrane playing a vital role as a drug barrier.
To vividly depict the antibacterial mechanism and further support the above results, DPBF was used to monitor the production of singlet oxygen. The characteristic absorption peak of DPBF at 425 nm significantly decreased with increased irradiation time, indicating that red light could trigger the generation of singlet oxygen by VP (Fig. 4e). In contrast, only very little singlet oxygen was generated without VP (Fig. 4f). Unlike antibiotics, PDT uses appropriate excitation light in combination with a photosensitizer and oxygen, allowing nonspecific attack on microorganisms by producing cytotoxic ROS, especially singlet oxygen,35 without inducing resistant strains. As mentioned before, VP-gel has good antibacterial effects against both SA and MRSA. Therefore, photoresponsive hydrogels combine the advantages of light and hydrogels and are increasingly applied in biomedicine, showing great application prospects in preventing wound infection and promoting wound healing.21
3.4. Antibacterial VP-gel promotes wound healing
VP-gel has been proved to be effective in cellular bacterial sterilization in vitro. For further application, the wound healing effects of hydrogels in vivo must be evaluated. To accomplish this, we used hydrogels to treat animals in wound healing experiments. After the wounds were infected with MRSA and suppurated, different treatments were applied to the mice (Fig. 5a). The wound area was recorded every 2 days (Fig. 5b and c). The group of mice treated with VP-gel (light) exhibited better wound closure compared with other groups because red light irradiation could enhance the antibacterial activity of VP in the wound healing process. VP-gel without red light irradiation also expedited wound healing. VP-gel accelerated wound healing and significantly improved epidermal regeneration compared to untreated or vancomycin-treated groups. However, the wound was difficult to heal with single VP treatment, and only 20% of the wound area was closed after 6 days. Significantly slower wound healing occurred compared to the other treatment groups. These results suggest that hyaluronic acid promotes wound repair and the sustained release of VP from the VP-gel can both prevent scarring and accelerate wound healing.
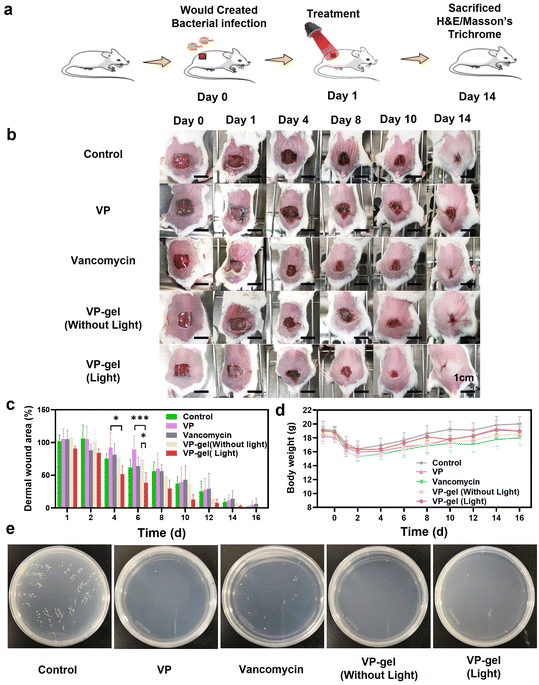 |
| Fig. 5
In vivo wound healing experiment. (a) Schedule of the animal experiments. (b) Representative images of the wounds over 14 days after different treatments. (c) The change in the wound area after different treatments. All data are presented as the mean ± SD (n = 5, *p < 0.05). All statistical analyses were performed by one-way ANOVA. (d) Body weight tracking of mice from different treatment groups. (e) Bacterial colonies of wounds 14 days after different treatments. | |
Furthermore, the epithelial tissue of the repaired wound was sliced and stained with H&E and Masson's trichrome (MT) for histological analysis. H&E-stained slices indicated the inflammation status of the wound (Fig. 6b). In the control group, inflammatory cells in the wound area indicated an intense immune response against bacteria. For the other groups, H&E staining showed fewer inflammatory cells, indicating that there was milder inflammation and demonstrating the antibacterial efficiency of the treatments.
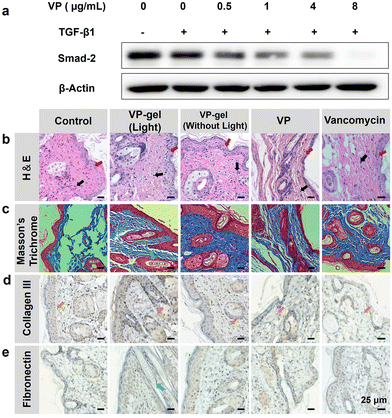 |
| Fig. 6 (a) Effects of different concentrations of VP on the expression of Smad2 protein in fibroblasts treated with TGF-β1. (b and c) Images of skin tissues with H&E and Masson staining of wound sites at 14 days post infection. (d and e) Micrographs of immunohistochemical staining for collagen III and fibronectin. (Red arrows: epidermal layer. Green arrows, hair follicle in skin. Orange arrows, collagen fibers. Black arrows, inflammatory cells. Scale bars: 25 μm.) | |
The excellent antibacterial effects of VP-gel were further verified by the in vivo bacterial counts of the wounds (Fig. 5e). Tissues of the wounds were acquired after different treatments to count the bacterial colonies. Hundreds of MRSA colonies were observed from the control group, while only several colonies grew on the VP-gel (light), VP-gel (without light) and VP groups. Fewer bacterial colonies corresponded to improved wound healing in Fig. 5c. The results obtained from the above experiments indicated that VP-gel effectively killed MRSA in the wound without damaging normal tissue.
To address safety concerns, we also conducted a potential toxicity study of the therapy in vivo. The body weight of the mice was measured during the wound healing experiment, which showed normal weight gain and no significant differences between the groups (Fig. 5d). Finally, in vivo H&E staining of major organs was performed to evaluate the potential toxicity of the hydrogel. The H&E-stained sections of the heart, liver, spleen, lungs and kidneys harvested from sacrificed mice also revealed no obvious signs of damage or inflammatory lesions to the major organs after 14 days of treatment (Fig. S9†). Thus, it could be concluded that the hydrogel possessed excellent biocompatibility and biosafety and was promising for application in scarless healing of infected wounds.
3.5. VP-gel mitigates scar formation in wound healing process through TGF-β1 pathway inhibition
To evaluate the role of VP-gel in mitigating scar formation, the collagen fibers in scar tissues from the different groups were assessed by immunohistochemistry analysis. As expected, the group treated with VP-gel and red light irradiation showed a significant decrease in numerous capillaries, fibroblasts and inflammatory cells compared to other groups. In contrast, the collagen bundles in the PBS-treated group were abundant, thick, and irregularly arranged (Fig. 6d and e). The collagen fibers in VP-gel treated samples were much fewer and thinner, and more well-organized collagen gaps demonstrated internal wound closure. A shorter collagen gap indicated a better wound healing effect. VP-gel with red light irradiation showed the shortest collagen gap, whereas the largest gap was observed in the PBS group, which could be attributed to suppression of skin scarring after initial skin wound closure and re-epithelialization by sustained release of VP. TGF-β1 participates in cellular processes, including stimulation of angiogenesis, proliferation of fibroblasts, differentiation of myofibroblasts, synthesis of collagen, and remodeling of the ECM.36,37 Continuous positive autocrine feedback may lead to tissue fibrosis38 and scar proliferation. Smad proteins, such as intracellular effectors of TGF-β1 signaling, are the only downstream substrates of TGF-β1 receptors known thus far39 and are central to the intracellular signaling of TGF-β1.40 The Hippo pathway effectors TAZ and YAP direct Smad protein activity to regulate TGF-β family-induced cellular responses in stem cells41 and cancers such as malignant mesothelioma42 and breast cancer.43 Therefore, we hypothesized that YAP inhibitors could inhibit Smad2 expression to inhibit scar hyperplasia. To evaluate the anti-scarring mechanism of VP, we stimulated 3T3 cells with TGF-β1, incubated them with different concentrations of VP for 4 hours, and extracted the protein to quantify expression of the Smad2 protein. As shown in Fig. 6a, TGF-β1 stimulated the protein levels of Smad2, which was suppressed in a concentration-dependent manner in the VP group compared to the control. That is, VP can inhibit the formation of scars by inhibiting the expression of Smad2 protein.
Immunohistochemistry analysis further demonstrated that the reduction in collagen III fibers and fibronectin in the experimental group confirmed the anti-scarring effect of VP. However, as the role of TGF-β1 signaling in cutaneous wound repair is multifaceted, appropriate inhibition of TGF-β1 activity inhibits scarring.44 The sustained release effect of the hydrogel effectively inhibits the TGF-β1 pathway and avoids the side effect of inhibiting wound healing with a large amount of VP at the initial stage of wound healing. Taken together, our results provide compelling evidence that the VP-hydrogel can enhance skin wound repair while suppressing tissue fibrosis and scar formation in a mouse model.
4. Conclusions
In summary, we developed a novel biocompatible hydrogel as wound dressing with anti-scarring, antibacterial, and slow-release properties that promotes wound healing. VP-gel combined with red light irradiation treatment showed excellent wound healing and antibacterial effects that significantly accelerated wound closure and prevented scar formation. The histomorphological examination of immunohistochemically stained samples in a mouse full-thickness wound model demonstrated accelerated wound healing for VP-gel treated groups. The biosafety of VP-gel was confirmed at both the cellular and animal levels, making our strategy a promising therapeutic modality for scar-mitigated healing of infected wounds.
Conflicts of interest
There are no conflicts to declare.
Acknowledgements
We are grateful for the financial support from the National Natural Science Foundation of China (8170041559) and the Medical and Health Borrow to Transfer Foundation of Hefei 2020 (J2020Y05).
Notes and references
- G. C. Gurtner, S. Werner, Y. Barrandon and M. T. Longaker, Nature, 2008, 453, 314–321 CrossRef CAS.
- C. K. Nanditha and G. S. V. Kumar, Mater. Today Bio, 2022, 14, 100235 CrossRef CAS PubMed.
- R. G. Frykberg and J. Banks, Adv. Wound Care, 2015, 4, 560–582 CrossRef.
- S. Mascharak, H. E. desJardins-Park, M. F. Davitt, M. Griffin, M. R. Borrelli, A. L. Moore, K. Chen, B. Duoto, M. Chinta, D. S. Foster, A. H. Shen, M. Januszyk, S. H. Kwon, G. Wernig, D. C. Wan, H. P. Lorenz, G. C. Gurtner and M. T. Longaker, Science, 2021, 372, eaba2374 CrossRef CAS.
- B. S. Atiyeh, Aesthetic Plast. Surg., 2020, 44, 1320–1344 CrossRef.
- T. Yamamoto, Br. J. Dermatol., 2006, 155, 869–875 CrossRef CAS.
- R. Saddawi-Konefka and D. Watson, Facial Plast. Surg., 2019, 35, 260–266 CrossRef CAS.
- G. Giugliano, D. Pasquali, A. Notaro, S. Brongo, G. Nicoletti, F. D'Andrea, A. Bellastella and A. A. Sinisi, Br. J. Plast. Surg., 2003, 56, 804–809 CrossRef CAS.
- T. Alster, Dermatol. Surg., 2003, 29, 25–29 Search PubMed.
- H. Cho, M. R. Blatchley, E. J. Duh and S. Gerecht, Adv. Drug Delivery Rev., 2018, 2019, 267–288 Search PubMed.
- Z. S. Zhu, J. Ding, H. A. Shankowsky and E. E. Tredget, J. Cell Commun. Signaling, 2013, 7, 239–252 CrossRef.
- S. Barrientos, O. Stojadinovic, M. S. Golinko, H. Brem and M. Tomic-Canic, Wound Repair. Regen., 2008, 16, 585–601 CrossRef PubMed.
- C. Furlan, J. A. Berenbeim and C. E. H. Dessent, Molecules, 2020, 25, 5280 CrossRef CAS PubMed.
- M. J. Lee, M. R. Byun, M. Furutani-Seiki, J. H. Hong and H. S. Jung, J. Invest. Dermatol., 2014, 134, 518–525 CrossRef CAS PubMed.
- J. Liang, L. Wang, C. Wang, J. Shen, B. Su, A. L. Marisetty, D. Fang, C. Kassab, K. J. Jeong, W. Zhao, Y. Lu, A. K. Jain, Z. Zhou, H. Liang, S. C. Sun, C. Lu, Z. X. Xu, Q. Yu, S. Shao, X. Chen, M. Gao, F. X. Claret, Z. Ding, J. Chen, P. Chen, M. C. Barton, G. Peng, G. B. Mills and A. B. Heimberger, Cancer Immunol. Res., 2020, 8, 952–965 CrossRef CAS.
- X. Zhang, D. Cai, F. Zhou, J. Yu, X. Wu, D. Yu, Y. Zou, Y. Hong, C. Yuan, Y. Chen, Z. Pan, V. Bunpetch, H. Sun, C. An, T. Yi-Chin, H. Ouyang and S. Zhang, Biomaterials, 2020, 232, 119724 CrossRef CAS PubMed.
- Y. Wang, L. Wang, J. T. F. Wise, X. Shi and Z. Chen, Toxicol. Appl. Pharmacol., 2020, 387, 114852 CrossRef CAS PubMed.
- M. Shah, D. M. Foreman and M. W. Ferguson, J. Cell Sci., 1995, 108, 985–1002 CrossRef CAS PubMed.
- Q. Xu, A. Sigen, Y. Gao, L. Guo, J. Creagh-Flynn, D. Zhou, U. Greiser, Y. Dong, F. Wang, H. Tai, W. Liu, W. Wang and W. Wang, Acta Biomater., 2018, 75, 63–74 CrossRef CAS.
- X. Liu, M. Wu, M. Wang, Q. Hu, J. Liu, Y. Duan and B. Liu, Adv. Mater., 2022, 34, e2109010 CrossRef PubMed.
- A. Maleki, J. He, S. Bochani, V. Nosrati, M. A. Shahbazi and B. Guo, ACS Nano, 2021, 15, 18895–18930 CrossRef CAS PubMed.
- W. Liu, W. Ou-Yang, C. Zhang, Q. Wang, X. Pan, P. Huang, C. Zhang, Y. Li, D. Kong and W. Wang, ACS Nano, 2020, 14, 12905–12917 CrossRef CAS PubMed.
- Y.-Q. Wang, X.-Y. Dou, H.-F. Wang, X. Wang and D.-C. Wu, Chin. J. Polym. Sci., 2021, 39, 1421–1430 CrossRef CAS.
- X. Xiao, Y. Zhu, J. Liao, T. Wang, W. Sun and Z. Tong, Biopolymers, 2020, 111, e23354 CrossRef CAS PubMed.
- D. He and H. Li, Adv. Funct. Mater., 2020, 30, 2004709 CrossRef CAS.
- L. E. Tracy, R. A. Minasian and E. J. Caterson, Adv. Wound Care, 2016, 5, 119–136 CrossRef.
- A. Song, A. A. Rane and K. L. Christman, Acta Biomater., 2011, 8, 41–50 CrossRef.
- H. Ye, Y. Xian, S. Li, C. Zhang and D. Wu, Biomater. Sci., 2022, 10, 4218–4227 RSC.
- L. Koivusalo, M. Kauppila, S. Samanta, V. S. Parihar, T. Ilmarinen, S. Miettinen, O. P. Oommen and H. Skottman, Biomaterials, 2019, 225, 119516 CrossRef CAS.
- Y. Liang, M. Li, Y. Yang, L. Qiao, H. Xu and B. Guo, ACS Nano, 2022, 16, 3194–3207 CrossRef CAS.
- C. Jiao, L. Gao, H. Zhang, B. Yu, H. Cong and Y. Shen, Biomacromolecules, 2020, 21, 1234–1242 CrossRef CAS.
- H. Cong, K. Wang, Z. Zhou, J. Yang, Y. Piao, B. Yu, Y. Shen and Z. Zhou, ACS Nano, 2020, 14, 5887–5900 CrossRef CAS PubMed.
- R. Yu, M. Li, Z. Li, G. Pan, Y. Liang and B. Guo, Adv. Healthc. Mater., 2022, 11, e2102749 CrossRef PubMed.
- C. Y. Chen, H. Yin, X. Chen, T. H. Chen, H. M. Liu, S. S. Rao, Y. J. Tan, Y. X. Qian, Y. W. Liu, X. K. Hu, M. J. Luo, Z. X. Wang, Z. Z. Liu, J. Cao, Z. H. He, B. Wu, T. Yue, Y. Y. Wang, K. Xia, Z. W. Luo, Y. Wang, W. Y. Situ, W. E. Liu, S. Y. Tang and H. Xie, Sci. Adv., 2020, 6, eaba0942 CrossRef CAS.
- T. Dai, Y. Y. Huang and M. R. Hamblin, Photodiagn. Photodyn. Ther., 2009, 6, 170–188 CrossRef CAS PubMed.
- T. He, X. Bai, L. Yang, L. Fan, Y. Li, L. Su, J. Gao, S. Han and D. Hu, Cell. Physiol. Biochem., 2015, 37, 666–676 CrossRef CAS PubMed.
- J. Massagué, S. W. Blain and R. S. Lo, Cell, 2000, 103, 295–309 CrossRef.
- Y. H. Li, J. Yang, Z. Zheng, D. H. Hu and Z. D. Wang, J. Cosmet. Dermatol., 2021, 20, 1374–1380 CrossRef.
- R. Derynck and Y. E. Zhang, Nature, 2003, 425, 577–584 CrossRef CAS.
- L. Zunwen, Z. Shizhen, L. Dewu, M. Yungui and N. Pu, Burns, 2012, 38, 404–413 CrossRef.
- T. A. Beyer, A. Weiss, Y. Khomchuk, K. Huang, A. A. Ogunjimi, X. Varelas and J. L. Wrana, Cell Rep., 2013, 5, 1611–1624 CrossRef CAS.
- M. Fujii, T. Toyoda, H. Nakanishi, Y. Yatabe, A. Sato, Y. Matsudaira, H. Ito, H. Murakami, Y. Kondo, E. Kondo, T. Hida, T. Tsujimura, H. Osada and Y. Sekido, J. Exp. Med., 2012, 209, 479–494 CrossRef CAS PubMed.
- S. E. Hiemer, A. D. Szymaniak and X. Varelas, J. Biol. Chem., 2014, 289, 13461–13474 CrossRef CAS.
- L. Lu, A. S. Saulis, W. R. Liu, N. K. Roy, J. D. Chao, S. Ledbetter and T. A. Mustoe, J. Am. Coll. Surg., 2005, 201, 391–397 CrossRef PubMed.
|
This journal is © The Royal Society of Chemistry 2023 |