DOI:
10.1039/D2BM01508K
(Paper)
Biomater. Sci., 2023,
11, 298-306
Light and immunostimulant mediated in situ re-education of tumor-associated macrophages using photosensitizer conjugated mannan nanoparticles for boosting immuno-photodynamic anti-metastasis therapy†
Received
18th September 2022
, Accepted 2nd November 2022
First published on 7th November 2022
Abstract
In an immunosuppressive tumor microenvironment, tumor-associated macrophages (TAMs) are the most abundant cells displaying pro-tumorigenic M2-like phenotypes, encouraging tumor growth and influencing the development of resistance against conventional therapies. TAMs are highly malleable. They can be repolarized into tumoricidal M1-like cells. In this study, we report the synthesis of novel co-operative immuno-photodynamic nanoparticles involving TAM self-targeting acrylic acid grafted mannan (a polysaccharide) conjugated with the chlorin e6 (Ce6) photosensitizer and then loaded with resiquimod (R848), a toll-like receptor (TLR7/8) agonist. The mannan conjugated Ce6 loaded with R848 (MCR) as bioconjugate nanoparticles demonstrated selective targeting of anti-inflammatory M2-like cells. Using photodynamic therapy they were repolarized to pro-inflammatory M1-like cells with combined effects of reactive oxygen species (ROS)-triggered intracellular signaling and a small-molecule immunostimulant. The MCR also demonstrated a TAM-directed adaptive immune response, inhibited tumor growth, and prevented metastasis. Our results indicate that these MCR nanoparticles can effectively target TAMs and modulate them for cancer immunotherapy.
1. Introduction
Immunotherapy has emerged as one of the standard pillars in anti-cancer and conventional therapies, including surgery, chemotherapy, and radiation.1 The immunosuppressive tumor microenvironment is one of the most crucial characteristics that affect therapeutic outcomes when treating solid tumors.2 The tumor immune microenvironment (TIME) comprises a variety of immune cells. Among them, tumor-associated macrophages (TAMs) are the principal tumor-infiltrating innate immune cells.3 TAMs can be polarized to either pro-tumorigenic M2-like or tumoricidal M1-like cells.4 Most TAMs exhibit the M2-like phenotype.5 Efforts have been made to deplete M2-like cells specifically or convert them into M1-like cells.6–9 TAMs play a critical role in tumor progression and growth.
Targeting TAMs has become the prime focus of immunotherapy. Currently, antibodies and small molecule inhibitors that can regulate multiple intracellular pathways are explored in clinical and preclinical studies to target and repolarize TAMs.10 Effective anti-cancer immunotherapy can be acquired through the selective elimination of TAMs or repolarization of M2-like cells into M1-like cells. One of the efficient ways of targeting TAMs is using CD206 receptors,11 which are overexpressed on TAMs.12 Mannan is a highly branched biopolymer comprising an α-(1→6) linked mannopyranose backbone.13 It is a natural ligand for CD206 receptors. It has been reported that TAMs can effectively take up mannan through CD206-mediated endocytosis and phagocytosis.14
Reactive oxygen species (ROS) can act as secondary messengers for TAM repolarization through the oxidation of cysteine residues of proteins. ROS can also activate the adaptive immune response by augmenting the initiation of proteasomes involved in the cross-presentation of antigens.10 Photodynamic therapy (PDT) is now recognized as a clinical strategy for treating cancer and non-cancerous lesions. PDT uses a combination of a photosensitizer (PS) and localized near-infrared (NIR) light radiation to effectively produce ROS, which can cause vascular damage and apoptosis and elicit an immune response.15 PDT can augment dendritic cell maturation and increase the proliferation of cytotoxic CD8 T cells, eliminating untreated tumors in other body locations.16
Another strategy to reprogram TAMs is targeting and activating their toll-like receptors (TLRs), which are innate immunity pattern recognition receptors.17 These TLRs can stimulate and convert TAMs into M1-like cells upon interaction with their ligands. Resiquimod (R848), a TLR7/8 agonist, has gained much attention in recent years because of its capacity to augment the repolarization of TAMs while triggering a highly robust antitumor response and inducing the secretion of the pro-inflammatory cytokine IL-12 by TAMs.4 R848 has also been used with other TLR stimulants, such as poly I:C, a TLR-3 agonist, to synergistically activate TAMs.18
In this study novel TAM-targeted co-operative nanoparticles were synthesized, which are composed of a polysaccharide mannan backbone polymer conjugated to hydrophobic ROS-producing chlorin e6 (Ce6) and loaded with resiquimod (R848), a TLR7/8 agonist. The resulting mannan conjugated Ce6 loaded with R848 (MCR) nanoparticles can selectively target TAMs through overexpressed CD206 receptors. They can trigger the repolarization of protumor M2-like cells into antitumoral M1-like cells by combining the effects of locally produced ROS and immune activation through a small-molecule immunostimulant (Scheme 1). Compared to monotherapy, the MCR demonstrated higher TAM polarization with laser irradiation. Furthermore, upon administration, the MCR demonstrated effective internalization and repolarization of M2-like cells into M1-like cells, thereby suppressing tumor growth in lymph node metastasis, a predictor associated with poor prognosis in colon cancer. To the best of our knowledge, studies demonstrating co-operative immuno-photodynamic therapy nanoparticles with TAM targeting capability and combinatorial repolarization ability that can eradicate tumors and prevent distinct metastasis have not yet been reported.
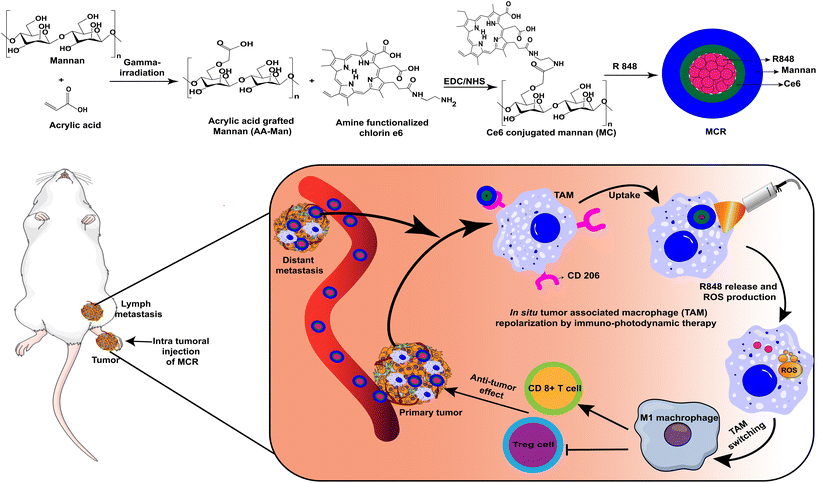 |
| Scheme 1 The schematic illustration of MCR's synthesis route, co-operative immuno-photodynamic mannan-Ce6, and its self-assembled nanoparticle loaded with the TLR7/8 adjuvant R848. Upon intratumoral injection of MCR into the primary tumor in a lymph node metastasis tumor model, the MCR successfully targets TAMs through CD206 and polarizes protumor M2-like cells into antitumoral M1-like cells through combinatorial actions of ROS produced through PDT and R848. In addition to TAM switching, treatment with MCR can decrease the number of immune-suppressive T cells but increase the number of cytotoxic T lymphocytes (CTLs). | |
2. Experimental section
2.1. Cell lines and generation of bone marrow-derived macrophages
A J774A.1 mouse monocyte/macrophage cell line and a CT-26 mouse colon cancer cell line were cultured in Dulbecco's modified Eagle's medium (DMEM) supplemented with 10% fetal bovine serum (FBS) and 1% penicillin–streptomycin (P/S) (Welgene, Gyeongsan, Korea) at 37 °C with 5% CO2. J774A.1 cells were stimulated with lipopolysaccharide (LPS, 100 ng mL−1) + interferon-gamma (IFN-γ, 10 ng mL−1) and interleukin-4 (IL-4, 20 ng mL−1) for 24 h to become M1- and M2-like macrophages, respectively. Bone marrow-derived macrophages (BMDM) were isolated and polarized using previously published methods.19 Briefly, bone marrow was isolated from the femur and tibia of Balb/c mice. Red blood cells were lysed using ammonium–chloride–potassium (ACK) lysing buffer (Gibco, NY, USA). The resulting cells were supplemented with BMDM growth medium consisting of DMEM, 10% FBS, and 15% filtered (0.2 μm) L-929 cell culture supernatant (the supernatant was obtained from L929 cells cultured for seven days). The BMDM growth medium was replenished every three days. On day seven, the cells were collected and stimulated with 100 ng ml−1 LPS and 50 ng ml−1 IFNγ to obtain M1 macrophages or stimulated with 10 ng ml−1 IL-4 to obtain M2 macrophages.
2.2. Synthesis of acrylic acid grafted mannan
Mannan (200 mg) was dissolved in 1, 5, and 10 wt% acrylic acid solutions with 0.01 M Mohr's salt in distilled water. The mannan and acrylic acid mixtures were exposed to gamma rays (60 Co source, ACEL type C-1882) at a dose of 25 kGy and a rate of 10 kGy h−1. After irradiation, the samples were washed with distilled water using a dialysis membrane for 48 h to remove unreacted acrylic acid. They were then freeze-dried to obtain dry acrylated mannan samples. The amount of acrylic acid conjugated to mannan was quantified by a toluidine blue-O (TBO) assay. The resulting acrylated mannan sample was immersed and reacted in a TBO solution for 4 h at room temperature. To remove any unreacted TBO, the samples were dialyzed against distilled water for 48 h using a dialysis membrane. The TBO-treated acrylated mannan was dissolved in an extraction solution (0.1 M NaOH
:
ethanol = 1
:
4, v/v) for complete elution. The extracted TBO was quantified by measuring the absorption at 530 nm using a microplate reader (Powerwave XS, Biotek, VT, USA).
2.3. Synthesis of aminated Ce6
To synthesize aminated Ce6, two molar excess of N-(3-dimethylamino propyl)-N′-ethyl carbodiimide hydrochloride (EDC) and N-hydroxysuccinimide (NHS) were added to 0.042 mmol of Ce6 in DMSO. After 30 min of stirring, 16 μL of ethylenediamine was added and refluxed overnight in the presence of N2 gas. Afterward, the solution was dialyzed against distilled water using a dialysis membrane with a molecular weight cut-off (MWCO) of 1000 Da, freeze-dried, and characterized by 1H-NMR.
2.4. Synthesis of Ce6 conjugated mannan (MC)
For the synthesis of MC, two molar excess of EDC was added to 16 mg mL−1 acrylic acid grafted mannan dissolved in formamide. After 30 min of stirring under a N2 atmosphere, 10 mg mL−1 aminated Ce6 in DMF was added to the above solution and stirred for 16 h. After the conjugation, the solution was collected and subjected to dialysis using a dialysis membrane with an MWCO of 10–14 kDa against distilled water, freeze-dried, and characterized by 1H-NMR.
2.5. Preparation of MCR
For MCR synthesis, 20 mg of MC and 2 mg of R848 were first dissolved in DMSO and then added to 20 mL of distilled water dropwise under sonication for 10 min. Afterward, the solution underwent dialysis against distilled water using a dialysis membrane with an MWCO of 12–14 kDa. It was then lyophilized. The drug loading of R848 was determined using high-performance liquid chromatography (HPLC) analysis.
2.6.
In vitro release of R848
The release pattern of R848 from the MCR was evaluated using the dialysis method. Briefly, 2 mg mL−1 MCR was resuspended in 1 mL of 1× PBS and placed in a dialysis bag with an MWCO of 3.5 kDa. The release medium was 20 mL of 1× PBS. The whole setup was kept inside a shaking (200 rpm) incubator at 37 °C. 1 mL of sample was collected and supplemented with 1 mL of 1× PBS at regular intervals. The quantity of R848 released into the medium was estimated using HPLC.
2.7. Cellular viability
Effects of the MCR on the cellular viability of M2-like macrophages before and after irradiation were assessed using a WST-1 assay (SFC Probes, Gyeonggi, Korea). Briefly, M2-like macrophages prepared from J774.1 cell lines, as described earlier, were seeded in 96-well plates at a density of 5000 cells per well, and cultured overnight at 37 °C with 5% CO2. Afterward, the cells were treated with various concentrations of MCR ranging from 25 μg mL−1 to 1.6 μg mL−1 and incubated for 6 h. After the incubation, the medium was aspirated, and fresh medium was added to each well. The cells were then subjected to laser irradiation for 5 min at 670 nm and 100 mW cm−2. Cell viability was then assessed using WST-1 following the manufacturer's protocol.
2.8. Intracellular uptake
Intracellular trafficking of the MCR was assessed using M1-like or M2-like macrophages obtained from J774.1 cells after stimulation or M2-like primary macrophages derived from BMDMs. Briefly, cells were seeded onto eight-well chamber slides at a seeding density of 5000 cells per well and incubated at 37 °C with 5% CO2 overnight. The medium was subsequently aspirated. The samples were supplemented with new medium and incubated at 37 °C with 5% CO2 for 6 h. Afterward, the medium was aspirated. The cells were washed with 1× PBS, fixed with 4% paraformaldehyde for 10 min, and washed with 1× PBS three times. The nuclei of the cells were counter-stained with 4′,6-diamidino-2-phenylindole (DAPI). The fluorescence of each sample was observed with a fluorescence microscope (Evos FL Auto 2, Life Technologies, Carlsbad, CA, USA).
2.9. Quantification of intracellular ROS production
To quantify intracellular ROS, 2′,7′-dichlorodihydrofluorescein diacetate (DCF-DA) was used. Briefly, 1 × 105 M2-like macrophage cells were seeded. After treatment with MCRL, the cells were irradiated as described previously. The cells were then treated with 25 μM DCF-DA in D-Hank's solution. After incubating the cells at room temperature for 20 min, their fluorescence intensity was visualized using a fluorescence microscope (ZOE fluorescent cell imager, Bio-Rad, Hercules CA, USA) at an excitation wavelength of 434 nm and an emission wavelength of 535 nm.
2.10. Biodistribution, bioluminescence imaging, and antitumor reduction study
All in vivo procedures were approved by the animal care and use committee of Chonnam National University Medical School and Chonnam National University Hospital, South Korea (CNU-IACUC-H-2019-24). To develop a footpad metastasis model to see the effect of MCRL on the metastasis of CRC, one million CT-26 cells were injected into the right soles of the back feet of male Balb/c mice (5 weeks old).20,21 Twelve days after the injection, the mice were randomly divided into five groups (four mice in each group) and intratumorally injected with PBS, R848 (1 mg kg−1), MCR (1.4 mg kg−1 Ce6, 1 mg kg−1 R848), MC + laser (MCL, 1.4 mg kg−1 Ce6), or MCR + laser (MCRL, 1.4 mg kg−1 Ce6, 1 mg kg−1 R848). The tumor volume and overall body weight of the mice were measured every two days. For the biodistribution study, the mice were intratumorally injected with the MCR, and fluorescence images at different time points were obtained by optical imaging using a fluorescence-labeled organism bioimaging instrument (FOBI, NeoScience, Gyeonggi, Korea). For bioluminescence imaging, one million CT-26-Luc cells were injected into the right soles of the back feet of male Balb/c mice (5 weeks old). Bioluminescence imaging was used to track tumor growth using an in vivo system (Berthold Technologies, Germany).
2.11. Immunohistochemical analysis
For immunohistochemical analysis, 6 μm-thick cryosections of tumor tissues were used. These tissue sections were first thawed, blocked with 3% BSA solution, and washed three times with 1× phosphate-buffered saline containing Tween® detergent (PBST). Afterward, the tissue sections were incubated with anti-CD8, anti-FoxP3, and anti-iNOS antibodies in a humidified chamber at 4 °C overnight. Afterward, these tissue sections were washed three times with PBST solution and incubated for 30 min at room temperature with Flamma® 594-conjugated secondary antibodies (BioActs). The nucleus was counterstained with DAPI and observed under a fluorescence microscope (Evos FL Auto 2, Life Technologies, Carlsbad, CA, USA). For hematoxylin and eosin (H&E) staining, paraffin sections were stained with H&E. Pulmonary metastases were investigated after intratracheal injection of 15% India ink solution.22
2.12. Statistical analysis
GraphPad Prism software (GraphPad Software, San Diego, CA, USA) was used for all statistical analyses. All data are expressed as mean ± standard deviation (S.D.). Statistical significance was determined using two-way analysis of variance (ANOVA) and defined as follows: *, p < 0.05; **, p < 0.01; and ***, p < 0.001.
3. Results and discussion
3.1. Preparation of MCR
First, acrylic acid was grafted on mannan (AA-Man) through gamma irradiation at a dose of 25 kGy and a dose rate of 10 kGy h−1. The amount of COOH groups in untreated mannan and AA-Man was estimated with a toluidine blue O (TBO) assay. The results are shown in Fig. S1.† An increase in acrylic acid concentration resulted in more conjugation of COOH on mannan. The maximal conjugation was produced after gamma irradiation of mannan in 10 wt% acrylic acid solution. This sample was used for further experiments. For the synthesis of MC, first aminated Ce6 was synthesized and conjugated to AA-Man through an EDC/NHS reaction. The successful conjugation of Ce6 to the mannan backbone was confirmed through 1H-NMR analysis (Fig. 1a). The characteristic peaks of mannan were observed at 3.5–4.2 pm (–CH2–CH–),23 and those of Ce6 were observed at 6–7 ppm (–CH
CH2–).24 HPLC analysis revealed that the conjugation content of Ce6 in MC was 11.43 ± 2.64%. After successfully synthesizing MC, R848 was loaded into the MC through a dialysis method. The drug loading content (%) of R848 in the MCR was estimated using HPLC. It was found to be 3.83 ± 0.20%. Using dynamic light scattering analysis, the average hydrodynamic diameter and zeta potential of the MCR were determined to be 140.5 ± 16.9 nm and −23.7 ± 0.2 mV, respectively. FE-TEM analyses of the MC (Fig. 1b) and MCR (Fig. 1c) demonstrated a spherical morphology for both MC and MCR. We further evaluated the release of R848 from the MCR. Fig. 1d shows an initial burst release of R848 from the MCR within 24 h, followed by a sustained release.
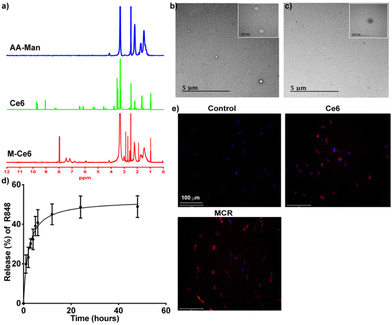 |
| Fig. 1 Physicochemical characterization of MCR. (a) 1H-NMR analyses of AA-Man, Ce6, and MC in DMSO-d6, (b) FE-TEM image of MC, (c) FE-TEM image of MCR, (d) drug release pattern of R848 from MCR, and (e) fluorescence microscopy images illustrating the cellular uptake of MCR in M2-like macrophages derived from BMDMs. | |
3.2. Cellular viability and cellular uptake of the MCR
To investigate the effect of the MCR on cellular viability, M2-like cells were treated with varying concentrations of MCR and subjected to PDT. As shown in Fig. S2,† MCRL significantly reduced the viability of M2-like cells, while no such effect was observed in CT-26 cells. This selective toxicity in M2-like cells suggests the selective uptake of the MCR by M2-like cells. We further evaluated its cellular uptake potential in M1 and M2-like cells. The MCR demonstrated a higher uptake in M2-type cells with negligible uptake in M1-type cells (Fig. S3 and S4†). The intracellular uptake potential of the MCR in M2-like macrophages derived from BMDMs was also explored. As shown in Fig. 1e, after 6 h of incubation, the MCR was intracellularly taken up by M2-like cells. The augmented cellular uptake of the MCR by TAMs can be attributed to mannan being a high-affinity ligand for mannose receptors, which are overexpressed by TAMs.10,25 ROS generated during PDT could modulate the polarization of TAMs by decreasing M2-type cells and increasing M1-type cells.26 To investigate whether the MCR could generate ROS during irradiation, intracellular ROS levels were measured using a DCFDA assay. As shown in Fig. S5,† upon laser irradiation, intracellular ROS levels were significantly increased in the MCRL group than in other groups due to the augmented uptake of the MCR in TAMs.
3.3. Biodistribution
Metastasis through the lymphatic system is a major reason for the reduction of the survival rate of colorectal cancer (CRC) patients.27 Lymph node metastasis is one of the main routes through which metastasis occurs in CRC.28,29 Therefore, precise imaging of lymph nodes and suppression of lymph node metastasis could increase the overall survival rate. To develop a lymph node metastasis model of CRC, CT-26 cells were injected into the hind paws of mice to induce tumors.21 Twelve days after the injection of cancer cells into the hind paws, metastasis into the sentinel lymph node (SLN) was observed. To investigate the TAM targeting of the MCR, we injected the MCR intratumorally into the primary tumor and tracked its migration from the tumor to the SLN through NIRF imaging. As evident from the NIRF signals shown in Fig. 2a and b, the MCR rapidly accumulated in the SLN within one hour after injection. The highest accumulation was observed at 3 h post-injection. These observations suggest that the MCR could effectively migrate to the SLN and target the TAMs.
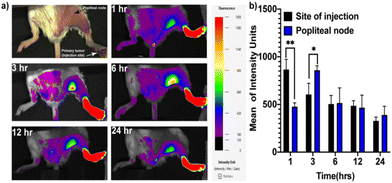 |
| Fig. 2 (a) The in vivo distribution of MCR in a lymph node metastasis model; the white arrow shows the primary tumor (injection site) and the black arrow shows a tumor in the popliteal node. (b) NIR fluorescence quantification at the site of injection and the popliteal node (n = 4 mice). *p < 0.05 and **p < 0.01. | |
3.4. Tumor reduction study and lung metastasis
To explore the MCR as an immuno-photodynamic therapy agent and investigate the metastasis to the SLN, we intratumorally injected a CT-26-Luc lymph node CRC metastasis model with PBS, R848, MC, or MCR. Three hours after the injection, the tumor region in the footpad and the SLN were irradiated with a 670 nm laser (100 mW cm−2) for 15 min. As seen in Fig. 3a, no bioluminescence signal was observed in the SLN of the MRCL group, whereas metastasis to the SLN was observed in other groups. We further measured the tumor volumes of mice treated with PBS, R848, MC + laser, or MCR. No significant reduction in tumor volume was observed in any of these groups.
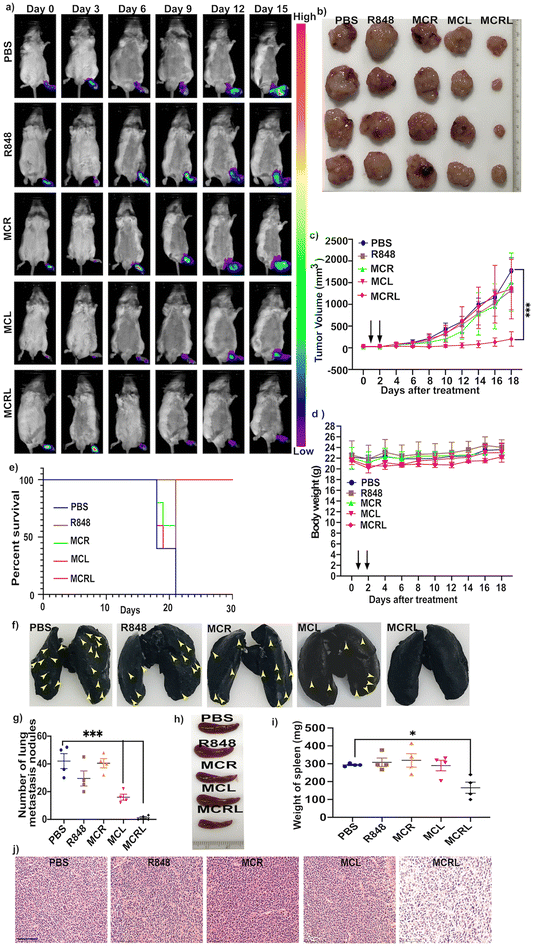 |
| Fig. 3 (a) Bioluminescence images of the CT-26-Luc lymph node metastasis model from different treatment groups (PBS, R848, MCR, MCL, and MCRL) until day 15. (b) Ex vivo tumor images of the CT 26 lymph node metastasis model from each treatment group on day 18. (c) Foot tumor volumes of the lymph node metastasis model after different treatments. (d) Whole-body weights of the lymph node metastasis model after different treatments. (e) Kaplan–Meier survival curves of treatment groups up to day 30. (f) Ex vivo images of lungs stained with India ink showing lung metastasis nodules on day 18. Tumor metastases appear as white nodules and are indicated with the yellow arrow. (g) Quantification of lung metastasis nodules on day 18. (h) Ex vivo images of the spleen and the weight of spleen on day 18. (j) Representative H&E images of the tumor on day 18. Scale bar = 100 μm. *p < 0.05, **p < 0.01 and ***p < 0.001. | |
However, the mice treated with the MCR and subjected to laser irradiation (MCRL) showed a significant tumor reduction compared to the mice in other groups (Fig. 3b, c and e). As shown in Fig. 3d, no significant decrease in body weight was observed in the MCRL group, suggesting the absence of any particle-induced toxicity. We further evaluated the survival of mice after treatment with MCRL. All the mice in the MCRL group survived beyond 30 days (Fig. 3e). To explore the tumor inhibition potential of MCRL, the mice treated with MCRL were euthanized 18 days after the initial treatment. Their lungs were excised and scored with the India-ink staining method for analysing lung metastasis. Fig. 3f and g show that the number of lung metastatic tumor nodules was significantly lower in groups treated with MCL and MCRL. We further investigated the effect of MCRL treatment on splenomegaly, usually seen during metastasis. Treatment with MCRL resulted in a significant reduction in spleen enlargement compared to the treatment with the control (PBS) (Fig. 3h and i), suggesting that the treatment with MCRL could inhibit the trafficking and accumulation of myeloid-derived suppressor cells (MDSC) in the spleen. To explore therapy-induced immunological changes, tumors and other major organs were collected on day 18 and stained with H&E. As shown in Fig. 3j, more tumor cell death was observed in the MCRL group than in other groups. Normal histological structures were observed in all remaining organs, such as the liver, spleen, heart, and kidneys (Fig. S6†).
3.5.
In vivo macrophage polarization and the antitumor effect
As R848 and ROS could activate pathways leading to the polarization of M2-like macrophage cells to M1-like cells, we investigated macrophage polarization in the tumor after treatment with MCRL. As shown in Fig. 4a and b, levels of cytokines TNF-α and IL-6 known to be secreted by M1 macrophages were significantly upregulated in the group treated with MCRL. Moreover, the combination of M2-specific targeted delivery of R848 and ROS resulted in increased levels of TNF-α and IL-6 cytokines in the group treated with MCRL compared to those in other treatment groups. We further investigated TAM polarization after treatment with MCRL by immunostaining tumor sections. An increase in iNOS+ macrophages (M1-like cells) was observed for sections from the MCRL treatment group (Fig. 4c and d). All these results suggest that upon treatment with MCRL, the polarization of M2-like macrophages to M1-like macrophages occurs. To explore the mechanism of tumor reduction when using MCRL treatment, we evaluated the number of cancer-killing immune cells, such as CTLs (CD 8+ T cells), and FOXP3+ regulatory T (Treg) cells known to suppress the immune response. As shown in Fig. 4c and f, the number of CD8 T cells was significantly higher in the group treated with MCRL than that in other groups, whereas the number of Treg showed a reverse trend. These results suggested that the decrease in the number of immune-suppressive T cells and increased CTLs were predominantly responsible for cell-mediated immune responses in the MCRL-treated group.
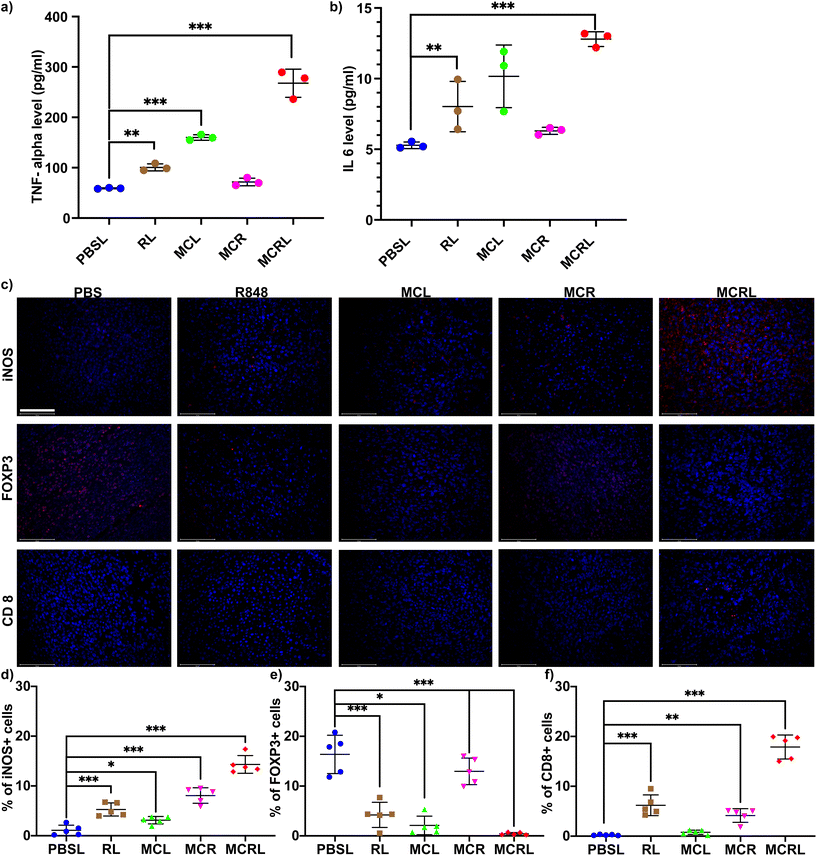 |
| Fig. 4 Levels of (a) TNF-α and (b) IL-6 cytokines in serum samples (n = 3 mice). (c) Fluorescence microscopy images of frozen tumor sections for analysing iNOS, FOXP 3, and CD 8 T cells. Scale bar = 100 μm. (d) Quantification of iNOS+ cells, FOXP3+ cells, and CD8+ T cells. *p < 0.05, **p < 0.01 and ***p < 0.001. | |
4. Conclusion
In conclusion, we successfully prepared novel co-operative immuno-photodynamic therapy nanoparticles, MCR, targeting the TAMs, modulating the TIME, preventing tumor metastasis by skewing TAMs to M1-like cells, changing the number of T immune cells, and preventing lung metastasis. The MCR could target M2-like cells selectively within the TIME and efficiently polarize the M2-like cells into M1-like cells upon laser irradiation, thereby inhibiting tumor growth and preventing metastasis. Furthermore, the repolarization also elevated the secretion of IL-6 and TNF-α, facilitated the proliferation of CD8+ T cells, and downregulated Treg cells that suppress the immune response. This work suggests a novel strategy for targeted co-operative immuno-photodynamic therapy, which can modulate the TAMs, inhibiting tumor growth and metastasis by controlling ROS signaling and TLR activation.
Author contributions
Saji Uthaman conceived the idea, performed the experiments, analyzed the data, and wrote the manuscript. Shameer Pillarisetti assisted in in vitro and in vivo tests. Youn-Mook Lim and Jin-Oh Jeong synthesized the polymer. Rizia Bardhan, Kang Moo Huh and In-Kyu Park supervised all the studies and revised the manuscript.
Conflicts of interest
There are no conflicts to declare.
Acknowledgements
This work was financially supported by the Bio & Medical Technology Development Program (No. NRF-2017M3A9E2056372) through the National Research Foundation of Korea (NRF), funded by the Korean government, MSIP. This work was also supported by a National Research Foundation of Korea (NRF) grant funded by the Korean government (MSIT) (No. 2020R1A2C2005620, 2020R1A5A2031185, NRF-2020M3A9G3080281 and NRF-2019M3E5D1A02068082). The authors are grateful to the Center for Research Facilities at the Chonnam National University for their assistance in the FE-TEM analysis.
References
- Y. R. Murciano-Goroff, A. B. Warner and J. D. Wolchok, Cell Res., 2020, 30, 507–519 CrossRef PubMed.
- K. Li, L. Lu, C. Xue, J. Liu, Y. He, J. Zhou, Z. Xia, L. Dai, Z. Luo and Y. Mao, Nanoscale, 2020, 12, 130–144 RSC.
- M. Oshi, Y. Tokumaru, M. Asaoka, L. Yan, V. Satyananda, R. Matsuyama, N. Matsuhashi, M. Futamura, T. Ishikawa and K. Yoshida, Sci. Rep., 2020, 10, 1–12 CrossRef.
- C. B. Rodell, S. P. Arlauckas, M. F. Cuccarese, C. S. Garris, R. Li, M. S. Ahmed, R. H. Kohler, M. J. Pittet and R. Weissleder, Nat. Biomed. Eng., 2018, 2, 578–588 CrossRef CAS PubMed.
- Z. Fridlender, A. Jassar, I. Mishalian, L.-C. Wang, V. Kapoor, G. Cheng, J. Sun, S. Singhal, L. Levy and S. Albelda, Br. J. Cancer, 2013, 108, 1288–1297 CrossRef CAS.
- L. Ringgaard, F. Melander, R. Eliasen, J. R. Henriksen, R. I. Jolck, T. B. Engel, M. Bak, F. P. Fliedner, K. Kristensen, D. R. Elema, A. Kjaer, A. E. Hansen and T. L. Andresen, Sci. Adv., 2020, 6, eaba5628 CrossRef CAS.
- H. Xiao, Y. Guo, B. Li, X. Li, Y. Wang, S. Han, D. Cheng and X. Shuai, ACS Cent. Sci., 2020, 6, 1208–1222 CrossRef CAS.
- X. Xu, X. Gong, Y. Wang, J. Li, H. Wang, J. Wang, X. Sha, Y. Li and Z. Zhang, Adv. Ther., 2020, 3, 1900181 CrossRef.
- X. Dai, L. Lu, S. Deng, J. Meng, C. Wan, J. Huang, Y. Sun, Y. Hu, B. Wu, G. Wu, J. F. Lovell, H. Jin and K. Yang, Theranostics, 2020, 10, 9332–9347 CrossRef CAS.
- C. Shi, T. Liu, Z. Guo, R. Zhuang, X. Zhang and X. Chen, Nano Lett., 2018, 18, 7330–7342 CrossRef CAS PubMed.
- A. Lepland, E. K. Asciutto, A. Malfanti, L. Simon-Gracia, V. Sidorenko, M. J. Vicent, T. Teesalu and P. Scodeller, Mol. Pharm., 2020, 17, 2518–2531 CrossRef CAS.
- J. M. Jaynes, R. Sable, M. Ronzetti, W. Bautista, Z. Knotts, A. Abisoye-Ogunniyan, D. Li, R. Calvo, M. Dashnyam, A. Singh, T. Guerin, J. White, S. Ravichandran, P. Kumar, K. Talsania, V. Chen, A. Ghebremedhin, B. Karanam, A. Bin Salam, R. Amin, T. Odzorig, T. Aiken, V. Nguyen, Y. Bian, J. C. Zarif, A. E. de Groot, M. Mehta, L. Fan, X. Hu, A. Simeonov, N. Pate, M. Abu-Asab, M. Ferrer, N. Southall, C. Y. Ock, Y. Zhao, H. Lopez, S. Kozlov, N. de Val, C. C. Yates, B. Baljinnyam, J. Marugan and U. Rudloff, Sci. Transl. Med., 2020, 12, eaax6337 CrossRef CAS PubMed.
- S. Uthaman, H. S. Kim, V. Revuri, J. J. Min, Y. K. Lee, K. M. Huh and I. K. Park, Carbohydr. Polym., 2018, 181, 27–33 CrossRef CAS PubMed.
- M. Song, T. Liu, C. Shi, X. Zhang and X. Chen, ACS Nano, 2016, 10, 633–647 CrossRef CAS.
- S. Marrache, J. H. Choi, S. Tundup, D. Zaver, D. A. Harn and S. Dhar, Integr. Biol., 2013, 5, 215–223 CrossRef CAS.
- C. Donohoe, M. O. Senge, L. G. Arnaut and L. C. Gomes-da-Silva, Biochim. Biophys. Acta, Rev. Cancer, 2019, 1872, 188308 CrossRef CAS PubMed.
- C. Anfray, A. Ummarino, F. T. Andón and P. Allavena, Cells, 2020, 9, 46 CrossRef CAS.
- B. Liu, Q. Liu, L. Yang, S. K. Palaniappan, I. Bahar, P. Thiagarajan and J. L. Ding, Sci. Signaling, 2016, 9, ra70–ra70 CrossRef.
- W. Ying, P. S. Cheruku, F. W. Bazer, S. H. Safe and B. Zhou, J. Visualized Exp., 2013, 50323 Search PubMed.
- D. Wan, H. Que, L. Chen, T. Lan, W. Hong, C. He, J. Yang, Y. Wei and X. Wei, Nano Lett., 2021, 21, 7960–7969 CrossRef CAS PubMed.
- Y. Wang, Y. Zeng, L. Zhu, J. Wan, N. Lei, X. Yao, X. Duan, Y. Zhang, Y. Cheng, N. Tao and Z. Qin, Front. Oncol., 2020, 10, 547683 CrossRef PubMed.
- S. Uthaman, S. Pillarisetti, K. M. Huh, C.-S. Cho and I.-K. Park, J. Ind. Eng. Chem., 2021, 97, 476–484 CrossRef CAS.
- M. Rabyk, A. Galisova, M. Jiratova, V. Patsula, L. Srbova, L. Loukotova, J. Parnica, D. Jirak, P. Stepanek and M. Hruby, J. Mater. Chem. B, 2018, 6, 2584–2596 RSC.
- J. Kim, W. Park, D. Kim, E. S. Lee, D. H. Lee, S. Jeong, J. M. Park and K. Na, Adv. Funct. Mater., 2019, 29, 1900084 CrossRef.
- D. Dangaj, K. L. Abbott, A. Mookerjee, A. Zhao, P. S. Kirby, R. Sandaltzopoulos, D. J. Powell Jr., A. Lamaziere, D. L. Siegel and C. Wolf, PLoS One, 2011, 6, e28386 CrossRef CAS.
- Y. Yang, X. Liu, W. Ma, Q. Xu, G. Chen, Y. Wang, H. Xiao, N. Li, X. J. Liang, M. Yu and Z. Yu, Biomaterials, 2021, 265, 120456 CrossRef CAS PubMed.
- H. Shi, R. Yan, L. Wu, Y. Sun, S. Liu, Z. Zhou, J. He and D. Ye, Acta Biomater., 2018, 72, 256–265 CrossRef CAS.
- G. Zhu, M. Zhao, Q. Han, Y. Tan, Y. U. Sun, M. Bouvet, S. R. Singh, J. Ye and R. M. Hoffman, Cancer Genomics Proteomics, 2020, 17, 131–139 CrossRef CAS.
- K. Naxerova, J. G. Reiter, E. Brachtel, J. K. Lennerz, M. van de Wetering, A. Rowan, T. Cai, H. Clevers, C. Swanton, M. A. Nowak, S. J. Elledge and R. K. Jain, Science, 2017, 357, 55–60 CrossRef CAS PubMed.
|
This journal is © The Royal Society of Chemistry 2023 |