DOI:
10.1039/D2BM01413K
(Paper)
Biomater. Sci., 2023,
11, 278-287
Injectable composite hydrogels encapsulating gelatin methacryloyl/chitosan microspheres as ARPE-19 cell transplantation carriers
Received
31st August 2022
, Accepted 18th November 2022
First published on 21st November 2022
Abstract
Retinal pigment epithelial (RPE) cell transplantation is being explored as a feasible approach for treating age-related macular degeneration. The low aggregation ability of RPE cell suspensions or microtissues after transplantation has limited cell utilisation. Therefore, alternative transplantation strategies should be explored to induce cell aggregation and maintain cell viability. Herein, we propose a composite hydrogel that encapsulates gelatin methacryloyl (GelMA)/chitosan microspheres (GCMSs) as ARPE-19 cell transplantation carriers. The diameter of the GCMS was adjusted by tuning the parameters of the microfluidic devices, yielding a cell-adhering platform that induced uniform cell spreading. The live/dead assay and immunofluorescence results showed that ARPE-19 cells adhered and spread uniformly around the microspheres. Moreover, the hydrogel sheets were used to provide an aggregated protective shell, and the ARPE-19 cells on the microspheres encapsulated within these hydrogel sheets remained viable post-injection and produced fewer reactive oxygen species after cyclic stretching. Furthermore, we found that the composite hydrogel was biodegradable and biocompatible in vivo. Therefore, GCMSs provide an injectable microcarrier for ARPE-19 cells, and the hydrogel provides an aggregated protective shell in this novel platform, which has considerable potential for an alternative injectable and highly aggregated RPE cell transplantation strategy design.
Introduction
Age-related macular degeneration (AMD) is a retinal disease characterised by blurred vision in the macular area, reducing the quality of life.1 The pathological mechanism of AMD mainly involves the degeneration of retinal pigment epithelial (RPE) cells, resulting in structural and functional impairment of the macular area, ultimately resulting in visual impairment.2 In addition, RPE cells cannot regenerate after degeneration; therefore, the implantation of healthy RPE cells to replace degenerate cells is an effective treatment.3 Recently, many alternative strategies for treating AMD, such as cell suspension injection,4 cell patch transplantation,5 and encapsulated-cell therapy (ECT),6 have been reported. Cell utilisation is low for cell suspension injection and uncontrolled diffusion occurs after injection.7 The fragile cell patches can easily fold and break. This has led to high demand for implantation techniques for cell patch transplantation.8 ECT can improve the utilisation rate of cells and requires less use of implantation technology.6,9
Microspheres have recently received attention as a form of ECT that can load a large number of cells and be implanted through non-invasive injections.6,10 In our previous research, hydrogel microspheres were microfabricated for tissue engineering in the context of neurology, chondrology, and cardiomyology.11–13 In this respect, different hydrogel microspheres used to carry or encapsulate cells are effective for cell growth.14 In ophthalmology, genetically modified cells have been encapsulated in microspheres and implanted into the eyeball as microcapsules that secrete cytokines.15 In addition, studies on RPE cell microspheres cultured in vitro showed that the microspheres could secrete functional pigment epithelial-derived factor, but it was easily spread owing to the poor retention of the microspheres.16 Therefore, an aggregated microsphere strategy is crucial for RPE cell transplantation.
Hydrogels play an increasingly vital role in tissue engineering.17,18 They mimic the extracellular matrix to support three-dimensional cell culture, such as the construction of 3D pigment epithelium-choriocapillaris models and ocular surface regeneration.10,19 Additionally, the encapsulation and shape plasticity of hydrogels enable them to encapsulate drugs and personalised recombinant cells, and integrate dispersed microspheres.20,21 In a recent study, hydrogel precursor solutions provided structural stability as polymer networks by integrating large numbers of microspheres together to co-create microscale heterogeneous cellular microenvironments.22 Furthermore, hydrogels can provide protection for 3D cell culture. For example, retinal cell culture inside or on the surface of a hydrogel can deliver retinal cells and promote retinal regeneration.23,24 Moreover, during the process of cell transplantation, cytoprotective, conservative, and natural cellular environment mimicking is very important. Therefore, the transplantation strategy of using hydrogels as an integrated network to aggregate cell microspheres while protecting cell growth is an important venture.6,25
Herein, we propose an injectable cell transplantation strategy that uses gelatin methacryloyl (GelMA) as an aggregative hydrogel and GelMA/chitosan microspheres (GCMSs) as ARPE-19 cell carriers (Fig. 1). GCMSs with a GelMA/chitosan (GC) dual-network were prepared using a microfluidic device, and their microstructures and sustained releases were evaluated. ARPE-19 cells were loaded on the surface of the microspheres and cell activity and proliferation were detected. Furthermore, the cell microspheres were mixed into GelMA precursor solutions, and a composite aggregative hydrogel was fabricated by injecting these mixed solutions. The characteristics of ARPE-19 cells within the composite hydrogel were then evaluated. The in vivo biocompatibility of composite hydrogels implanted subcutaneously was also evaluated, which indicated that GCMSs can be used as ARPE-19 cell microcarriers and have injectable properties. Moreover, GelMA hydrogel aggregating microspheres provide ARPE-19 cell protection, suggesting that the aggregative hydrogel composed of GCMS-GelMA provides a potential strategy for RPE cell transplantation.
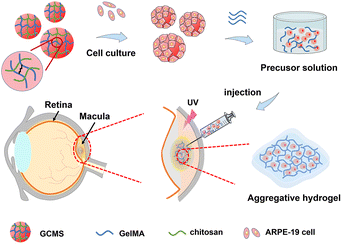 |
| Fig. 1 Schematic design of an injectable ARPE-19 cell transplantation strategy. Microspheres were fabricated based on a GelMA and chitosan double network hydrogel. ARPE-19 cells were loaded on microspheres, and the resulting cell microspheres were mixed with 7.5% (w/v) GelMA as an injectable precursor solution. The precursor solution was injected into the RPE cell layer in the macular area to form a composite aggregate hydrogel. | |
Results and discussion
Fabrication and characterisation of GelMA/chitosan microspheres
In this study, GC dual-network hydrogel microspheres were used as cell carriers because of the aforementioned benefits.13 The GC double network hydrogel synthesis consisted of two steps: firstly, GelMA was cross-linked with lithium phenyl-2,4,6-trimenthylbenzoyphosphinate (LAP) as the photo-initiator under UV irradiation, and secondly, physical cross-linking occurred with chitosan by providing OH− (Fig. 2a). GelMA hydrogels were biocompatible, as they can simulate the extracellular matrix, and they have been extensively used in three-dimensional cell cultures.17,26,27 Chitosan has become one of the components of many medical products owing to its unique properties such as biocompatibility, mucosal adhesion, non-toxicity, and gel-forming ability.28–30 Therefore, considering the strengths of GelMA and chitosan, we chose these two materials to prepare the GC double network hydrogel for microsphere fabrication. The Fourier transform infrared (FT-IR) results showed that specific chemical groups of these two materials were observed in the GC double network hydrogel (Fig. 2b). Compared with the FT-IR spectra of GelMA polymers, the peak at 1640 cm−1 of the GC double network hydrogel was decreased, confirming the photo-cross-linking of the GC double network hydrogel after UV irradiation. However, the two peaks at 1620 cm−1 and 1530 cm−1 of the proton –NH3+ group of the chitosan amine functional group were reduced. These results indicate that chitosan undergoes deprotonation and forms new hydrogen bonds during physical cross-linking. The mechanical properties of biomaterials are considered principal factors for regulating the cell morphology and behaviour.31,32 The mechanical properties of GelMA and GC hydrogels were characterized by rheological properties. The storage modulus (G′) of the GelMA hydrogel ranged from 1360 ± 30 Pa to 1380 ± 60 Pa, and the loss modulus (G′′) ranged from 12.1 ± 9.2 Pa to 23.7 ± 6.5 Pa (Fig. 2c). The G′ of the GC dual-network hydrogel ranged from 1090 ± 190 Pa to 1010 ± 240 Pa, and the G′′ ranged from 91.6 ± 4.9 to 40.8 ± 17.8 Pa (Fig. 2d). Clearly, the G′ of the GelMA and GC hydrogels was much greater than that of G′, and maintained a slight change, indicating that the cross-linking of the hydrogels was very stable.33 In addition, chitosan hydrogels have a higher loss elastic modulus, resulting in the elastic moduli of GC hydrogels being lower than those of GelMA hydrogels and being maintained at approximately 1000 Pa. It has also been recognised that the modulus of retinal tissue is approximately 1 kPa,34 which indicates that the GC double network hydrogel possesses the necessary mechanical properties for retinal tissue engineering.9
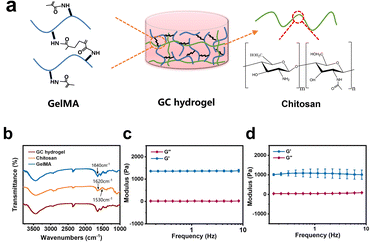 |
| Fig. 2 Synthesis and characterisation of GelMA and GC double-network hydrogels. (a) Schematic representation of the GC hydrogel after the photo-polymerisation of GelMA and the physical cross-linking of chitosan. (b) The FT-IR spectra of GelMA, chitosan, and GC hydrogel. G′ and G′′ of the GelMA hydrogel (c) and GC double-network hydrogel (d). | |
According to previous studies, microfluidic chips produce monodisperse microspheres.35 In our study, uniform GCMSs were produced using the coaxial single-channel structure of the chips (Fig. 3a–e). The microsphere diameter was inversely correlated with the velocity of the external phase and positively correlated with the velocity of the dispersed phase. Finally, microspheres with a diameter of approximately 121.56 ± 11.55 μm were prepared when the velocity of the dispersed phase was 5 μL min−1 and the velocity of the external phase was 1000 μL min−1 (Fig. 3e and i). Microspheres with a diameter of 120 μm met not only the conditions of loading a large number of ARPE-19 cells on the surface area but also the micro-size conditions of a general injection.15,36 The surface of the lyophilised GCMSs was observed using SEM (Fig. 3f–h). GCMS had completely spherical morphologies, and some chitosan nanoparticles with a diameter of 150 nm were observed on the surface (Fig. 3j). The results showed that homogeneous GelMA/chitosan microspheres were successfully prepared.
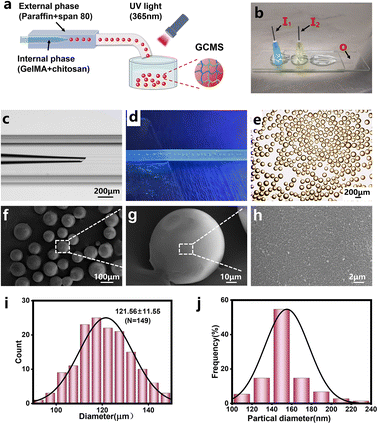 |
| Fig. 3 Fabrication process and SEM images of GCMSs. (a) The schematic diagram of microspheres was fabricated with a coaxial channel. (b) The optical image of the microfluidic chip. (c) The coaxial single-channel structure of the microfluidic ship. (d) The microspheres were generated and photo-cross-linked at the hose outlet. (e) Stable microspheres collected in solution. SEM images depicting (f) the uniformly dispersed morphology of GCMSs, (g) single observation of GCMS, and (h) surface microstructure of GCMS. (i) Corresponding size distribution of the monodisperse microspheres in solution from (e). (j) Diameter distribution of surface particles of GCMS from (h). | |
Encapsulation of bovine serum albumin (BSA) within GCMSs and sustained release behaviour
BSA (molecular weight [MW]: 66.446 kDa) was used as a model protein to explore the sustained release performance of GCMSs on the aforementioned conventional protein drugs because of its similar molecular weight to most macromolecular protein growth factors.37 Herein, the swelling and sustained-release properties of the GCMSs were investigated. The swelling behavior of hydrogel microspheres can effectively lead to adsorption of drugs.38–40 In addition, previous studies found that the higher specific surface area of hydrogels would lead to a faster swelling rate. Therefore, the hydrogel microspheres reached the equilibrium swollen state within a shorter time compared with the bulk hydrogel. The results showed that the diameter of dry GCMSs increased from 62 ± 6 to 86 ± 5 μm after only about 45 s when immersed in Dulbecco's phosphate buffered saline (DPBS) solution (Fig. 4b). The GCMSs exhibited a rapid swelling behavior, which might be beneficial for protein absorption into the microspheres. BSA grafted with fluorescein isothiocyanate (BSA-FITC) was used to explore the sustained-release performance of the GCMSs. Dry GCMSs were immersed in the BSA-FITC solution. The BSA-FITC solution was loaded into the microspheres during swelling (Fig. 4a). The green fluorescence of the GCMSs was observed by confocal laser scanning microscopy after 0, 1, 2, 4, and 6 days. The sustained release behavior was analysed using fluorescence intensity (Fig. 4c–q), which followed the semiquantitative processes in our previous studies.13,41 These results showed that the green fluorescence decreased gradually with time, indicating that BSA-FITC gradually decreased in GCMSs. The fluorescence images of microspheres at different time points were transformed into greyscale surface-plot 3D images. As shown in Fig. 4h–l, the greyscale of the surface graph decreased gradually from day 0 to day 6. The fluorescence images of the microspheres at different time points were also transformed into a distribution histogram of the matrix frequency of the greyscale. As shown in Fig. 4m–q, the grey peak decreases with an increase in the sustained release time. The above results showed that GCMS could sustainably release BSA for 6 days. Therefore, it was inferred that GCMS could also sustainably release drugs with similar molecular weights.
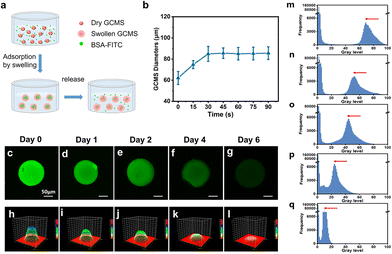 |
| Fig. 4 Adsorption and sustained release analysis of BSA-FITC in GCMSs. (a) The schematic illustration of BSA-FITC release in GCMSs. (b) The swelling ratio analysis of GC-MS immersed in DPBS. (c–g) The confocal fluorescence microscopy image of GCMSs loaded with BSA-FITC. The fluorescence intensity decreases with different time points including 0 day (c), 1 day (d), 2 days (e), 4 days (f), and 6 days (g). (h–l) The greyscale surface plot 3D image converted from confocal fluorescence microscopic image at 0 days (h), 1 day (i), 2 days (j), 4 days (k), and 6 days (l). (m–q) The distribution histogram of the matrix frequency of the grey level converted from the confocal fluorescence microscopy image at 0 day (m), 1 day (n), 2 days (o), 4 days (p), and 6 days (q). | |
For AMD, macromolecular protein medicines, such as ranibizumab (MW: 48 kDa), bevacizumab (MW: 149 kDa), and pegaptanib sodium (MW: 50 kDa), are applied in clinical settings.42–44 The MW of these drugs is similar to that of BSA; therefore, GCMS has potential as a drug carrier for sustained release. Microspheres encapsulated in scaffolds can provide a more stable and controllable, sustained release.45,46 Therefore, in our subsequent experiments, we integrated microspheres into the GelMA hydrogel, which may lead to a more controllable release strategy. The microspheres were encapsulated in GelMA to form a nuclear shell structure. GelMA as a shell has the potential to prolong the sustained release time of protein factors from the microspheres.
Cell viability and proliferation of ARPE-19 cells on GCMSs
ARPE-19 is a cell line derived from the human retinal pigment epithelium (RPE), showing similar functions to natural RPE and providing a reliable source of cells for RPE injury repair-related research.47 ARPE-19 cells were seeded on GCMSs to observe the cell viability and proliferation on GCMSs, and cells on tissue culture polystyrene (TCP) as the control group. The viability of ARPE-19 cells on microspheres and TCP was measured using a live/dead fluorescence assay kit after 1, 3, and 5 days of culture. As shown in Fig. 5, most cells were green, but few were red, indicating that most cells were alive (Fig. 5a–f). The statistical analysis also showed that the viability of ARPE-19 cells in the GCMS group was more than 90% at 1, 3, and 5 days (Fig. 5g). There was no significant difference in the viability of ARPE-19 cells between the GCMS and TCP groups. The proliferation of ARPE-19 cells in the GCMS and TCP groups was detected using Alamar blue viability reagent. The samples were quantitatively analysed after 1, 3, and 5 days of culture by comparing the fluorescence intensity, and the results showed that the fluorescence intensity increased gradually with increasing culture time (Fig. 5h). Statistical analysis showed no significant difference between the GCMS and TCP cell groups after 1, 3, and 5 days of culture. All the above results indicate that the GCMSs have good biocompatibility. ARPE-19 cells cultured on GCMSs exhibited excellent adhesion, viability, and proliferation. GCMSs as a platform was able to induce the adhesion and proliferation of ARPE-19 cells, suggesting their potential as biological and functional injectable cell carriers for the treatment of AMD.
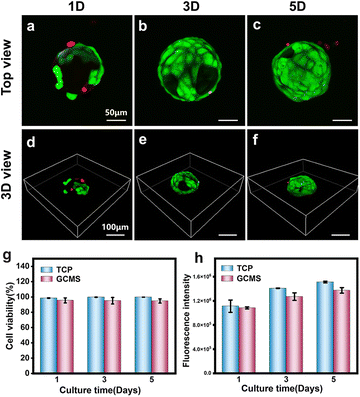 |
| Fig. 5 Activity and proliferation of ARPE-19 cells loaded on GCMSs. (a–f) Confocal fluorescence microscopy image of cell viability staining after 1, 3, and 5 days of culture, living cells in green and dead cells in red. (g) Quantitative analysis of cell viability on GCMSs and TCP, after 1, 3, and 5 days of culture. (h) Quantitative analysis of cell proliferation on GCMSs and TCP, after 1, 3, and 5 days of culture. | |
Cell-carrying GCMS encapsulated in GelMA
Hydrogels with extracellular matrix-like components have been used as delivery media for various bioactive substances.48 In this study, GelMA was chosen as an aggregative biomaterial owing to its biocompatibility and convenience. We investigated the biocompatibility of 7.5% and 10% (weight/volume [w/v]) GelMA hydrogels using cells cultured in these hydrogels. Fig. 6a shows that more cells cultured in the 7.5% (w/v) GelMA hydrogel were alive compared to those in the 10% (w/v) GelMA hydrogel. Quantitative analysis also showed that the cell viability ratio was approximately 98%, which was significant compared to the 10% group (Fig. 6b). The 7.5% (w/v) GelMA hydrogel had a higher porosity than the 10% GelMA hydrogel, making it easier to exchange the nutrients needed by cells, resulting in good spread and growth of cells.49 Then, cell microspheres were encapsulated in the 7.5% GelMA hydrogel to obtain a composite aggregation hydrogel, and cell growth was observed after 2 days of culture (Fig. 6c). To observe the morphology of the cell-carrying GCMS encapsulated in the hydrogel, the cytoskeleton was stained with a rhodamine probe. A cell suspension three-dimensional culture was used as the control group. The fluorescence staining results showed that the cells on the microspheres were well-spread (Fig. 6d–k). In addition, there was an agglomeration phenomenon of microspheres, such as two or three connected microspheres (Fig. 6g and h). However, the cell suspension failed to spread in the hydrogel (Fig. 6d and e). Quantitative analysis of the relative area of cell membranes in each group showed that the membrane area and nuclear area of cells on the microspheres were significantly larger than those of suspended single cells (Fig. 6l and m). These results suggest that using microspheres as cell carriers enabled the maintenance of a better ARPE-19 cell spread morphology compared to the cell suspension in the hydrogel. We found that the cells on the microspheres were mixed with the aggregative GelMA precursor solution in a spread morphology. After GelMA cross-linking, the cells continued to grow in a spread morphology and were connected with each other in the composite hydrogel. However, the cell suspension was a single cell in a suspended state, which could not grow directly in the spread form in the hydrogel. These data suggest that GCMS is an excellent ARPE-19 cell carrier. The composite hydrogel was formed by microspheres combined with GelMA to maintain the cell morphology after transplantation.
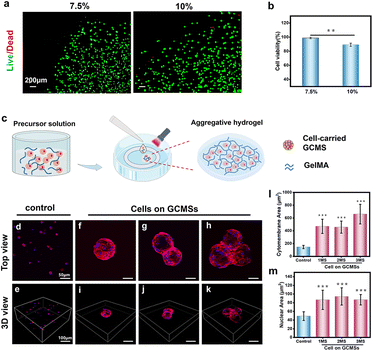 |
| Fig. 6 Viability and morphological analyses of ARPE-19 cells in the hydrogel after culture for 2 days. (a) Fluorescence microscopy image of ARPE-19 cell viability staining in 7.5% (w/v) GelMA and 10% (w/v) GelMA hydrogels. (b) Quantitative analysis of cell viability on the GelMA hydrogel. (c) Schematic illustration of ARPE-19 cell culture in the GelMA hydrogel. The top and 3D views of fluorescence images of the control of cell suspension in the hydrogel (d and e) and cell microspheres in a hydrogel (f–k) stained for F-actin (Rhodamine, red) and nuclei (DAPI, blue). (l) Quantitative analysis of the cytomembrane area in the GCMS group and single-cell group. (m) Quantitative analysis of the nuclear area in the GCMS group and single-cell group. **P < 0.01, and ***P < 0.001. | |
Oxidative stress effect and biocompatibility of the injectable GCMS encapsulated GelMA hydrogel
Injectable biomaterials are ideal medical materials with minimal invasiveness and higher patient compliance, and are thus suitable for cell therapy and tissue engineering.50–52 In this study, cell microspheres were mixed with the 7.5% (w/v) GelMA solution to prepare GelMA injectable solution. The composite solution has injectable properties owing to the small size of the microspheres and fluidity of the GelMA solution. Suspended cells can be damaged by mechanical shear forces during injection.53 To explore the viability of cells after injection, the viability of cells carrying GCMS encapsulated in GelMA was analysed using live/dead fluorescence staining after culturing for 2 and 4 days. Fluorescence images showed that most of the cells were viable (Fig. 7a). In addition, statistical analysis showed that the survival rate of ARPE-19 cells could reach more than 80% on days 2 and 4. The survival rate of cells was not significantly different between the two groups, indicating that the cells were viable for 4 days even after injection (Fig. 7b). Cyclic stretching can induce the release of reactive oxygen species (ROS), resulting in oxidative damage, increased inflammatory factors in RPE cells, and ultimately apoptosis.52,54,55 To explore the stress protection performance of this injectable hydrogel system for cells, hydrogels were prepared on BioFlex stretching plates and cyclically stretched for 6 h (Fig. 7c). ROS and mitochondrial superoxide species (MitoSOX) in ARPE-19 cells were detected using a fluorescent probe to detect oxidative stress in cells after stretching. The results showed that the total ROS and MitoSOX produced by the cells in the hydrogel were lower than those in the positive control group (Fig. 7d and e).
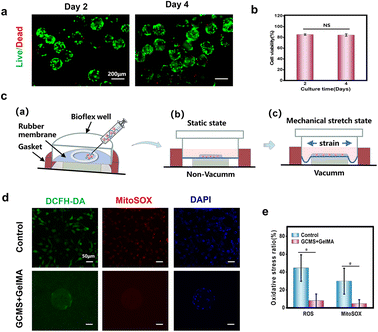 |
| Fig. 7 Cytoprotective and oxidative stress effect of cell-carrying GCMSs encapsulated in a GelMA hydrogel. (a) After injection, the viability of cell staining in the hydrogel sheet after 2 and 4 days of culture. (b) Analysis of cell activity in the hydrogel sheet after injection. (c) Schematic diagram of the preparation of hydrogel sheet encapsulated cell microspheres in a BioFlex well, ((a)) the injection process, and ((b)) side view of the BioFlex well at the static state. ((c)) Side view of the BioFlex well at a mechanical stretch state. (d) The confocal fluorescence microscopy image of ARPE-19 cell expressed ROS (DCFH-DA, green), mitochondrial superoxide species (MitoSOX, red) and nucleus (DAPI, blue) under cyclic stretching. (e) The ratio of oxidative stress cells to all cells. * P < 0.05. NS: not significant. | |
According to the above results, the cells remained highly viable after the injection, and the cell oxide production of the hydrogel sheet group was less than that of the positive control group after cyclic stretching, indicating that the sheet had a certain stress protection performance for cells. Therefore, the injectable GCMS-encapsulated GelMA hydrogel is a good RPE cell carrier.
In vivo biocompatibility and biodegradability of composite hydrogels
The degradation rate of the hydrogel affected the release rate of the encapsulated cells after transplantation. To further observe the in vivo biocompatibility of the composite hydrogels, a subcutaneous transplantation experiment was performed. As shown in Fig. 8, haematoxylin and eosin (H&E) staining showed that both groups had inflammatory responses, and the inflammatory area in the GelMA/GCMS group was relatively larger than that in the GelMA group (Fig. 8a–c). The degree of degradation of the GelMA/GCMS group was higher than that of the GelMA group after 5 days, and both groups were almost completely degraded after 10 days (Fig. 8d). Meanwhile, the GelMA hydrogel was degraded in vitro. The enzyme degradation rate of the GelMA hydrogel in vitro can mimic its degradation rate in vivo to a certain extent.56,57 The 7.5% (w/v) GelMA hydrogel sheets with a diameter of 1 cm and a height of 5 mm were soaked with 0.025 U mL−1 type ii collagenase solution, and the results showed that the GelMA hydrogel sheets were almost completely degraded after 7 days (Fig. 8e).
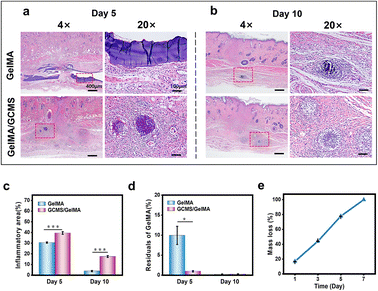 |
| Fig. 8 Composite hydrogel biocompatibility in different groups after subcutaneous transplantation into Sprague–Dawley rats. Microscopy observations of H&E-stained tissue sections of the hydrogel at day 5 (a) and day 10 (b) after subcutaneous transplantation. (c) Quantitative analysis of the inflammatory area for each group. (d) Quantitative analysis of the residuals of GelMA for each group. (e) The enzyme degradation rate of the GelMA hydrogel. * P < 0.05. *** P < 0.001. | |
H&E staining images showed that the residual GCMS of the GelMA/GCMS group was not completely degraded after 5 days, and GelMA components were almost completely degraded. GCMS was released 5 days after GelMA degradation was complete. In vitro degradation experiments showed that the GelMA hydrogel was completely degraded at a stable rate within 7 days. The microspheres in the composite hydrogel were released with stable degradation of the hydrogel in vivo. Therefore, the composite hydrogel is biodegradable and biocompatible, and has the potential to be used as a biomaterial for retinal implants.
Experimental
Materials
Porcine skin gelatin, methacrylic anhydride (MA), 4′,6-diamidine-2-phenylindole dihydrochloride (DAPI), and bovine serum albumin-fluorescein isothiocyanate (BSA-FITC) were purchased from Sigma-Aldrich (Shanghai, China). Chitosan (low viscosity), acetic acid (anhydrous, ≥99.9%), Span 80, and liquid paraffin were purchased from Aladdin Industrial Corporation (Shanghai, China). Sodium hydroxide powder and potassium bromide crystals were purchased from Macklin. LAP was purchased from Stemeasy (Wuxi, Jiangsu, China). The Calcein/PI cell viability/cytotoxicity assay kit and 2,7-dichlorodihydrofluorescein diacetate (DCFH-DA) were purchased from Beyotime (Shanghai, China). The cytoskeleton red fluorescent probes were purchased from KeyGen BioTECH (Jiangsu, China). DPBS, Dulbecco's modified Eagle's medium/nutrient mixture F-12 (DMEM-F12), foetal bovine serum (FBS), penicillin (100 U mL−1), and streptomycin (100 U mL−1) were purchased from Gibco. The MitoSOX Red mitochondrial superoxide indicator was purchased from Invitrogen (Beijing, China).
Preparation and characterisation of GelMA and GC hydrogels
The GelMA polymer was synthesised following a previously reported procedure.58,59 Briefly, porcine skin gelatin was mixed at 10% (w/v) in DPBS at 55 °C for 30 min and stirred until fully dissolved. Subsequently, 20% (v/v) MA was added to the gelatin solution at a 0.5 mL min−1 rate while being stirred at 50 °C and allowed to react for 3 h. Later, the mixture was slowly dripped into 95% alcohol while stirring with a stirring rod, and the flocculant precipitated on the stirring rod. The precipitate was dissolved in 3× DPBS at 60 °C for 1 h. The mixture was loaded into a dialysis tube (cut off at 5 kDa MW) and immersed in deionised water for 5 days to completely remove the residual reagent. The solution was freeze-dried for 2 days to form GelMA polymer foam and stored under vacuum drying conditions. The degree of methacrylation of the free amine group of the synthesised polymer was obtained by 1H NMR spectroscopy. GC double network hydrogels were synthesised following a previously reported synthesis method.13 One percent (w/v) chitosan was dissolved in 1.5% (volume/volume [v/v]) acetic acid, and thereafter 10% (w/v) GelMA and 0.2% (w/v) LAP were added to obtain the GC precursor solution. The GC precursor solution was cross-linked twice, in which GelMA was photo-cross-linked after irradiation with 365 nm UV light for 15 s, and chitosan was precipitated under OH− conditions to undergo physical cross-linking. The rheological properties of the 10% (w/v) GelMA and GC hydrogels were measured using a rheometer (HAAKE Mars 40). The cross-linked gel was approximately 2 mm thick and 2 cm in diameter when pre-solution drops were added to the parallel-plate configuration. The GelMA polymer, chitosan, and GC hydrogels were freeze-dried for 2 days. FT-IR spectra (Nicolet 6700) of the lyophilised samples were measured in the wavelength range of 3700–1000 cm−1 over thirty scans.
Preparation of a coaxial microfluidic chip
Based on our previous study,11 a coaxial microfluidic device was fabricated. This microfluidic chip was a single-channel dispersion device consisting of a capillary tube with an inner diameter of 150 μm inserted into a square quartz tube with an inner diameter of 500 μm. The circular capillary tube had an inner diameter of approximately 80 μm using a capillary puller and was placed in a square quartz tube (Fig. 3c). Then, two dispensing needles were vertically connected with two inlets of a circular capillary and square quartz tube from disperse phase I1 and external phase I2, respectively. The other end of the square quartz tube was outlet O. Finally, it was sealed and fixed with an epoxy resin adhesive (Fig. 3b).
Fabrication of GCMSs
The GC precursor solution mentioned above was connected to the I1 inlet as the disperse phase, and liquid paraffin containing 5% span-80 was connected to the I2 inlet as the external phase. The hose was connected to the outlet of the square quartz tube and used to transport the preliminary microspheres, which were cross-linked by UV irradiation during transport (Fig. 3a–d). A 15 mL centrifuge tube containing 3 mL of 0.3 M NaOH was used to receive the microspheres at the outlet of the hose. The mixed microsphere solution was collected in a 15 mL centrifuge tube and centrifuged at 1500 rpm for 3 min. After centrifugation, the microspheres were immersed in sodium hydroxide solution at the bottom of the tube for 2 min to achieve physical cross-linking. After cross-linking, the microspheres were transferred to an EP tube and washed three times with deionised water. The microspheres were dehydrated with 50%, 70%, 80%, 90%, and 95% alcohol successively, and finally freeze-dried for 2 days. The freeze-dried GCMSs were coated with gold, and their microstructure was observed using a scanning electron microscope.
The adsorption and sustained release of BSA by GCMSs
Hydrogels exhibit particular swelling properties. Therefore, dry GCMSs were immersed in DPBS and their diameters were analysed at 0, 15, 30, and 45 s to evaluate swelling. Dry GCMSs were soaked in BSA-FITC at a concentration of 2 mg mL−1. The soaked microspheres were incubated in a constant-temperature shaker for 24 h and washed with DPBS three times before observation. An inverted laser confocal microscope (LSM 880 with Airyscan) was used to observe the residual fluorescence of BSA-FITC in the microspheres after 0, 1, 2, 4, and 6 days. Confocal microscopy superimposed the 3D scanning microspheres to produce a 2D fluorescence image. Finally, 2D fluorescence images were analysed using ImageJ software.
Cell viability and proliferation analysis of ARPE-19 cells on GCMSs
ARPE-19 cells were purchased from American Type Culture Collection (ATCC) and cultured in a carbon dioxide incubator using DMEM/F12 complete medium. The complete medium contained 89% DMEM/F12 basal medium supplemented with 10% foetal bovine serum and 1% penicillin–streptomycin. The cells were washed with DPBS and then cultured with fresh complete medium every 2 days. When the confluence of ARPE-19 cells in the dish reached about 85%, the cells were digested with trypsin and centrifuged, then resuspended and passaged at a 1
:
3 dilution ratio.
GCMSs were sterilised by soaking them in 75% alcohol for 40 min. The microspheres were washed with DPBS three times before cell implantation and soaked in the medium for 3 min to facilitate cell adhesion. ARPE-19 cells at a density of 10 × 104 mL−1 were seeded on sterilised GCMSs in 48-well plates (n ≥ 3). As a control, cells were seeded on tissue culture polystyrene (TCP) at the same density. ARPE-19 cell viability was detected using the Calcein/PI cell viability/cytotoxicity assay kit (Beyotime). In brief, after culturing for 1, 3, and 5 days, ARPE-19 cells were washed three times with DPBS, and then 300 μL Calcein/PI (0.1% v/v) was added to each well and incubated for 10 min at 37 °C. The stained cells were observed under an inverted laser confocal microscope (LSM 880 with Airyscan). The living cells were stained with Calcein AM and fluoresced green, while dead cells were stained with PI and fluoresced red. Cell activity was quantitatively analysed using ImageJ software.
ARPE-19 cell proliferation was measured using an Alamar blue assay kit (Invitrogen). In brief, after 1, 3, and 5 days of cell culture, cells were washed three times with DPBS, and then 300 μL Alamar blue reagent (10% v/v) was added per well at 37 °C with 5% CO2 for 4 h. One cell-free well was used as the blank group. Subsequently, 100 μL of solution from each well was transferred into a 96-well plate, and the fluorescence values were read using a cell imaging multifunctional detection system (Cytation5). Cell proliferation was quantitatively analysed using Origin software.
The viability and morphology of ARPE-19 cells in the GelMA hydrogel
GelMA solutions of 7.5% (w/v) and 10% (w/v) were sterilised using a 0.45 μm filter. The digested ARPE-19 cells were suspended in 7.5% (w/v) and 10% (w/v) GelMA solutions and cross-linked in a Petri dish, which was then added to the culture medium and cultured in an incubator. The medium was then replaced daily. The activity of ARPE-19 cells in the hydrogel was observed using a live/dead detection kit (Biyuntian). In brief, cells were washed with DPBS three times, and the hydrogel was submerged in appropriate Calcein/PI (0.1% v/v) staining solution and incubated at 37 °C for 10 min. The cells were stained and observed under an inverted fluorescence microscope (BX63, OLYMPUS). Finally, ImageJ software and Origin software were used for the quantitative analysis of cell activity.
Actin filaments were observed in the cytoskeletal tissues of the ARPE-19 cells in the hydrogel. Cell microspheres and single cells were mixed with 7.5% GelMA at the bottom of the confocal dish to form a 500 μm thick hydrogel. After 2 days of growth in the hydrogel, the cells were fixed with 4% (w/v) paraformaldehyde for 20 min, covered with the immunostaining permeable solution Triton x-100 for 10 min, and closed with 1% (w/v) BSA for 2.5 hours. Closed cells were stained with a cytoskeletal red probe (Keygenbio) in 1% (w/v) BSA for 90 min. Finally, the nuclei were stained with DAPI (Sigma-Aldrich). The above operations are carried out at room temperature. Immunofluorescence images were observed using a laser confocal microscope (LSM880, Zeiss).
Fabrication of composite hydrogels based on cell-carrying GCMSs and GelMA
ARPE-19 cells were inoculated in the GCMSs and cultured for 3 days to ensure cell adhesion for the preparation of ARPE-19 cell-carrying hydrogel microspheres. Subsequently, ARPE-19 cell-carrying GCMSs were mixed with 7.5% (w/v) GelMA to develop cell microspheres encapsulated in GelMA injection solution. The composite solution was injected into the polydimethylsiloxane mould (inner diameter: 1.5 cm) in a Petri dish. A microinjection pump (injection speed: 100 μL min−1), syringe (volume: 1 mL), and 20 G needle (inner diameter: 600 μm) were used for injection. After injection, the composite solution was paved and irradiated with UV light for 15 s to form an ARPE-19 cell microsphere hydrogel sheet culture system, and then the culture medium was added and further cultured in the incubator for 4 days. The medium was replaced daily.
Cell viability and oxidative stress in cell-carrying GCMSs encapsulated in hydrogel sheets
Similar to the activity detection of suspended cells in the gel above, the activity of ARPE-19 cells in the hydrogel sheet was observed using the live/dead detection kit (Biyuntian). The oxidative stress in ARPE-19 cells on GCMSs in a hydrogel sheet composite culture system was also observed. Briefly, the composite solution was cross-linked on a six-well BioFlex plate (Flexcell) coated with collagen type I to form a hydrogel sheet culture system that adhered to the elastic sheet of the BioFlex plate. Cells were seeded on BioFlex plates at a density of 10 × 104 cells per mL as a positive control (n ≥ 3). A Flexcell FX-5000TM tension system (Flexcell International Corporation) was used for orderly cyclic mechanical stretching. The elastic sheet of the BioFlex plate was stretched at a frequency of 0.3 Hz, with an amplitude of 20% and a stretch rate of 1
:
1 for 5 h. Live cells were incubated with 2 μM DCFH-DA (Beyotime) working solution for 10 min to detect broad-spectrum reactive oxygen species, followed by 1 μM MitoSOX working solution for 15 min to detect mitochondrial superoxide. Finally, the nuclei were stained with DAPI (Sigma-Aldrich). Immunofluorescence images were observed under a laser confocal microscope (LSM880, Zeiss).
Subcutaneous implantation of hydrogel sheets
The hydrogel sheet was fabricated using a polydimethylsiloxane mould. For subcutaneous implantation, hydrogels were divided into two groups of samples: (1) 7.5% GelMA group and (2) 7.5% GelMA + GCMS group. Sprague–Dawley rats at 7 weeks were selected, and each rat was implanted with two different groups of hydrogel sheets. After 5 and 10 d, the specimens were retrieved. The collected specimens were fixed in 4% formaldehyde for 24 h, embedded in paraffin, and sectioned. H&E staining was used to observe the morphology of the tissues under an optical microscope.
Statistical analysis
The experiment was performed at least three times for each sample (per sample, N ≥ 3), and the results are expressed as mean ± standard deviation. An independent sample t-test was used for statistical analysis of all experimental data, and differences were considered statistically significant as P < 0.05. Data were analysed by using ImageJ and SPSS software.
Conclusions
In summary, we successfully developed injectable composite hydrogel-encapsulated GCMSs loaded with ARPE-19 cells as cell transplantation carriers to induce cell aggregation and maintain cell viability. Recently many studies have made progress in rpe cell transplantation strategies. However, few transplantation carriers can aggregate the original spread of cells using the injection method. In this study, the advantages of a large surface area and small size of GCMSs were utilised to ensure the number, original morphology, and injectability of cells during transplantation. The fluidity of the GelMA solution that encapsulates the cell microspheres was utilised to reduce cell damage during injection. Additionally, GelMA acts as an aggregative shell for cell microspheres for a short time after gelation. The results showed that GCMS as a cell adhering platform was able to maintain the activity and spread of ARPE-19 cells, and GelMA encapsulated and aggregated the cell microspheres as an injection medium. Therefore, injectable aggregative cell-carrying GCMSs encapsulated in a hydrogel may be used for ARPE-19 cell transplantation, potentially facilitating retinal tissue repair in the future.
Author contributions
X. L. Cheng, P. J. Yin and T. Li contributed equally to this work. X. L. Cheng carried out conceptualization, investigation, formal analysis, writing – original draft preparation, review & editing. P. J. Yin and T. Li carried out the task of investigation, review & editing. L. C. Luo carried out investigation and formal analysis. Y. Yang, L. Wang, W. W. Su, Y. L. Wang, and Y. B. Li, Y. F. Wang carried out the task of review & editing. W. H. Huang and Y. B. Wu provided conceptualization, review & editing, and supervision.
Conflicts of interest
There are no conflicts to declare.
Acknowledgements
This work was supported by the National Natural Science Foundation of China (32271181 and 32271423), the Science and Technology Project of Guangdong Province (2018B090944002), Guangdong Basic and Applied Basic Research Foundation (2020B1515120001 and 2021A1515410010), Discipline Construction Project of Guangdong Medical University (G622280009), and grants from the Visiting Scholar Foundation of Key Laboratory of Biorheological Science and Technology (Chongqing University), Ministry of Education (CQKLBST-2020-003).
References
- S. Binder, I. Krebs, R.-D. Hilgers, A. Abri, U. Stolba, A. Assadoulina, L. Kellner, B. V. Stanzel, C. Jahn and H. Feichtinger, Invest. Ophthalmol. Visual Sci., 2004, 45, 4151–4160 CrossRef PubMed.
- F. G. Holz, C. Bellmann, M. Margaritidis, F. Schütt, T. P. Otto and H. E. Völcker, Graefe's Arch. Clin. Exp. Ophthalmol., 1999, 237, 145–152 CrossRef CAS PubMed.
- B. B. Thomas, B. Lin, J. C. Martinez-Camarillo, D. Zhu, B. T. McLelland, G. Nistor, H. S. Keirstead, M. S. Humayun and M. J. Seiler, Front. Neurosci., 2021, 15, 752958 CrossRef PubMed.
- S. D. Schwartz, J.-P. Hubschman, G. Heilwell, V. Franco-Cardenas, C. K. Pan, R. M. Ostrick, E. Mickunas, R. Gay, I. Klimanskaya and R. Lanza, Lancet, 2012, 379, 713–720 CrossRef CAS PubMed.
- L. da Cruz, K. Fynes, O. Georgiadis, J. Kerby, Y. H. Luo, A. Ahmado, A. Vernon, J. T. Daniels, B. Nommiste, S. M. Hasan, S. B. Gooljar, A.-J. F. Carr, A. Vugler, C. M. Ramsden, M. Bictash, M. Fenster, J. Steer, T. Harbinson, A. Wilbrey, A. Tufail, G. Feng, M. Whitlock, A. G. Robson, G. E. Holder, M. S. Sagoo, P. T. Loudon, P. Whiting and P. J. Coffey, Nat. Biotechnol., 2018, 36, 328–337 CrossRef CAS PubMed.
- F. S. Y. Wong, K. K. Tsang, A. M. W. Chu, B. P. Chan, K. M. Yao and A. C. Y. Lo, Biomaterials, 2019, 201, 53–67 CrossRef CAS PubMed.
- R. Sharma, D. Bose, A. Maminishkis and K. Bharti, Annu. Rev. Pharmacol. Toxicol., 2020, 60, 553–572 CrossRef CAS PubMed.
- H. Kamao, M. Mandai, S. Okamoto, N. Sakai, A. Suga, S. Sugita, J. Kiryu and M. Takahashi, Stem Cell Rep., 2014, 2, 205–218 CrossRef CAS PubMed.
- F. Jiang, Z. Tang, Y. Zhang, Y. Ju, H. Gao, N. Sun, F. Liu, P. Gu and W. Zhang, Biomater. Sci., 2019, 7, 2335–2347 RSC.
- K. V. Manian, C. A. Galloway, S. Dalvi, A. A. Emanuel, J. A. Mereness, W. Black, L. Winschel, C. Soto, Y. Li, Y. Song, W. DeMaria, A. Kumar, I. Slukvin, M. P. Schwartz, W. L. Murphy, B. Anand-Apte, M. Chung, D. S. W. Benoit and R. Singh, Cell Stem Cell, 2021, 28, 846–862 CrossRef CAS PubMed.
- L. Lin, Y. Wang, L. Wang, J. Pan, Y. Xu, S. Li, D. Huang, J. Chen, Z. Liang, P. Yin, Y. Li, H. Zhang, Y. Wu, C. Zeng and W. Huang, RSC Adv., 2020, 10, 39662–39672 RSC.
- Z. Wang, L. Wang, T. Li, S. Liu, B. Guo, W. Huang and Y. Wu, Theranostics, 2021, 11, 7948–7969 CrossRef CAS PubMed.
- J. Chen, D. Huang, L. Wang, J. Hou, H. Zhang, Y. Li, S. Zhong, Y. Wang, Y. Wu and W. Huang, J. Colloid Interface Sci., 2020, 574, 162–173 CrossRef CAS.
- L. Yang, Y. Liu, L. Sun, C. Zhao, G. Chen and Y. Zhao, Nano-Micro Lett., 2021, 14, 4 CrossRef PubMed.
- E. Santos, G. Orive, A. Calvo, R. Catena, P. Fernández-Robredo, A. G. Layana, R. M. Hernández and J. L. Pedraz, J. Controlled Release, 2012, 158, 443–450 CrossRef CAS PubMed.
- A. Al-Ani, D. Toms, S. Sunba, K. Giles, Y. Touahri, C. Schuurmans and M. Ungrin, Int. J. Mol. Sci., 2021, 22, 11317 CrossRef CAS PubMed.
- S. Xiao, T. Zhao, J. Wang, C. Wang, J. Du, L. Ying, J. Lin, C. Zhang, W. Hu, L. Wang and K. Xu, Stem Cell Rev. Rep., 2019, 15, 664–679 CrossRef CAS.
- X. Wu, W. Guo, L. Wang, Y. Xu, Z. Wang, Y. Yang, L. Yu, J. Huang, Y. Li, H. Zhang, Y. Wu, G. Li and W. Huang, Adv. Funct. Mater., 2022, 32, 2110066 CrossRef CAS.
- Z. Zhong, X. Deng, P. Wang, C. Yu, W. Kiratitanaporn, X. Wu, J. Schimelman, M. Tang, A. Balayan, E. Yao, J. Tian, L. Chen, K. Zhang and S. Chen, Biomaterials, 2021, 267, 120462 CrossRef CAS.
- J. Bian, F. Cai, H. Chen, Z. Tang, K. Xi, J. Tang, L. Wu, Y. Xu, L. Deng, Y. Gu, W. Cui and L. Chen, Nano Lett., 2021, 21, 2690–2698 CrossRef CAS PubMed.
- N. R. Barros, H.-J. Kim, M. J. Gouidie, K. Lee, P. Bandaru, E. A. Banton, E. Sarikhani, W. Sun, S. Zhang, H.-J. Cho, M. C. Hartel, S. Ostrovidov, S. Ahadian, S. M. Hussain, N. Ashammakhi, M. R. Dokmeci, R. D. Herculano, J. Lee and A. Khademhosseini, Biofabrication, 2021, 13, 035032 CrossRef.
- Y. Fang, Y. Guo, M. Ji, B. Li, Y. Guo, J. Zhu, T. Zhang and Z. Xiong, Adv. Funct. Mater., 2022, 32, 2109810 CrossRef CAS.
- J. H. Park, E. Y. Shin, M. E. Shin, M. J. Choi, C. Carlomagno, J. E. Song and G. Khang, Int. J. Biol. Macromol., 2018, 117, 546–552 CrossRef CAS PubMed.
- H. S. Kim, D. Kim, Y. W. Jeong, M. J. Choi, G. W. Lee, M. Thangavelu, J. E. Song and G. Khang, Int. J. Biol. Macromol., 2019, 130, 220–228 CrossRef CAS.
- H. A. Thomson, A. J. Treharne, L. S. Backholer, F. Cuda, M. C. Grossel and A. J. Lotery, J. Biomed. Mater. Res., Part A, 2010, 95, 1233–1243 CrossRef CAS.
- A. Assmann, A. Vegh, M. Ghasemi-Rad, S. Bagherifard, G. Cheng, E. S. Sani, G. U. Ruiz-Esparza, I. Noshadi, A. D. Lassaletta, S. Gangadharan, A. Tamayol, A. Khademhosseini and N. Annabi, Biomaterials, 2017, 140, 115–127 CrossRef CAS.
- M. Nikkhah, N. Eshak, P. Zorlutuna, N. Annabi, M. Castello, K. Kim, A. Dolatshahi-Pirouz, F. Edalat, H. Bae, Y. Yang and A. Khademhosseini, Biomaterials, 2012, 33, 9009–9018 CrossRef CAS.
- F. S. Buosi, A. Alaimo, M. C. Di Santo, F. Elías, G. García Liñares, S. L. Acebedo, M. A. Castañeda Cataña, C. C. Spagnuolo, L. Lizarraga, K. D. Martínez and O. E. Pérez, Int. J. Biol. Macromol., 2020, 165, 804–821 CrossRef CAS PubMed.
- Z. Shariatinia, Int. J. Biol. Macromol., 2018, 120, 1406–1419 CrossRef CAS PubMed.
- Z. Shariatinia, Adv. Colloid Interface Sci., 2019, 263, 131–194 CrossRef CAS.
- S. Ishihara and H. Haga, Cancers, 2022, 14, 1049 CrossRef CAS.
- B. R. Seo, P. Bhardwaj, S. Choi, J. Gonzalez, R. C. Andresen Eguiluz, K. Wang, S. Mohanan, P. G. Morris, B. Du, X. K. Zhou, L. T. Vahdat, A. Verma, O. Elemento, C. A. Hudis, R. M. Williams, D. Gourdon, A. J. Dannenberg and C. Fischbach, Sci. Transl. Med., 2015, 7, 301ra130 Search PubMed.
- Y. Zhou, H.-L. Gao, L.-L. Shen, Z. Pan, L.-B. Mao, T. Wu, J.-C. He, D.-H. Zou, Z.-Y. Zhang and S.-H. Yu, Nanoscale, 2016, 8, 309–317 RSC.
- Y. Liu, R. Wang, T. I. Zarembinski, N. Doty, C. Jiang, C. Regatieri, X. Zhang and M. J. Young, Tissue Eng., Part A, 2013, 19, 135–142 CrossRef CAS.
- Y. Wu, L. Wang, B. Guo and P. X. Ma, J. Mater. Chem. B, 2014, 2, 3674–3685 RSC.
- K. J. R. Lewis, M. W. Tibbitt, Y. Zhao, K. Branchfield, X. Sun, V. Balasubramaniam and K. S. Anseth, Biomater. Sci., 2015, 3, 821–832 RSC.
- D. Huang, L. Wang, Y. Dong, X. Pan, G. Li and C. Wu, Eur. J. Pharm. Biopharm., 2014, 88, 104–115 CrossRef CAS PubMed.
- A. R. Karimi, B. Rostaminejad, L. Rahimi, A. Khodadadi, H. Khanmohammadi and A. Shahriari, Int. J. Biol. Macromol., 2018, 118, 1863–1870 CrossRef CAS.
- A. R. Karimi, M. Tarighatjoo and G. Nikravesh, Int. J. Biol. Macromol., 2017, 105, 1088–1095 CrossRef CAS PubMed.
- H. Zhang, Y. Liu, G. Chen, H. Wang, C. Chen, M. Li, P. Lu and Y. Zhao, Sci. Bull., 2020, 65, 380–388 CrossRef CAS.
- P. Chen, X. Ning, W. Li, Y. Pan, L. Wang, H. Li, X. Fan, J. Zhang, T. Luo, Y. Wu, C. Ou and M. Chen, Bioact. Mater., 2022, 14, 416–429 CrossRef CAS.
- E. S. Gragoudas, A. P. Adamis, E. T. Cunningham, M. Feinsod and D. R. Guyer, N. Engl. J. Med., 2004, 351, 2805–2816 CrossRef CAS PubMed.
- M. G. Maguire, D. F. Martin, G.-S. Ying, G. J. Jaffe, E. Daniel, J. E. Grunwald, C. A. Toth, F. L. Ferris and S. L. Fine, Ophthalmology, 2016, 123, 1751–1761 CrossRef.
- P. J. Rosenfeld, D. M. Brown, J. S. Heier, D. S. Boyer, P. K. Kaiser, C. Y. Chung and R. Y. Kim, N. Engl. J. Med., 2006, 355, 1419–1431 CrossRef CAS PubMed.
- A. Jaklenec, E. Wan, M. E. Murray and E. Mathiowitz, Biomaterials, 2008, 29, 185–192 CrossRef CAS PubMed.
- K. Song, Y. Liu, H. M. Macedo, L. Jiang, C. Li, G. Mei and T. Liu, Mater. Sci. Eng., C, 2013, 33, 1506–1513 CrossRef CAS PubMed.
- K. C. Dunn, A. E. Aotaki-Keen, F. R. Putkey and L. M. Hjelmeland, Exp. Eye Res., 1996, 62, 155–169 CrossRef CAS PubMed.
- Q. Huang, Y. Zou, M. C. Arno, S. Chen, T. Wang, J. Gao, A. P. Dove and J. Du, Chem. Soc. Rev., 2017, 46, 6255–6275 RSC.
- C.-H. Lin, J. J.-M. Su, S.-Y. Lee and Y.-M. Lin, J. Tissue Eng. Regener. Med., 2018, 12, 2099–2111 CrossRef CAS PubMed.
- R. d. Souza, P. Zahedi, C. J. Allen and M. Piquette-Miller, Biomaterials, 2009, 30, 3818–3824 CrossRef PubMed.
- M. Qu, X. Liao, N. Jiang, W. Sun, W. Xiao, X. Zhou, A. Khademhosseini, B. Li and S. Zhu, J. Biomed. Mater. Res., Part A, 2021, 109, 2091–2100 CrossRef CAS PubMed.
- Z. Tang, F. Jiang, Y. Zhang, Y. Zhang, Y. Yang, X. Huang, Y. Wang, D. Zhang, N. Ni, F. Liu, M. Luo, X. Fan, W. Zhang and P. Gu, Biomaterials, 2019, 194, 57–72 CrossRef CAS PubMed.
- B. G. C. Maisonneuve, D. C. D. Roux, P. Thorn and J. J. Cooper-White, Biomacromolecules, 2013, 14, 4388–4397 CrossRef CAS PubMed.
- X. Liang, Z. Wang, M. Gao, S. Wu, J. Zhang, Q. Liu, Y. Yu, J. Wang and W. Liu, BMC Ophthalmol., 2019, 19, 79 CrossRef PubMed.
- S. Wu, Q. Lu, N. Wang, J. Zhang, Q. Liu, M. Gao, J. Chen, W. Liu and L. Xu, BMC Ophthalmol., 2017, 17, 208 CrossRef PubMed.
- Z. Luo, W. Sun, J. Fang, K. Lee, S. Li, Z. Gu, M. R. Dokmeci and A. Khademhosseini, Adv. Healthcare Mater., 2019, 8, e1801054 CrossRef PubMed.
- X. Zhao, X. Sun, L. Yildirimer, Q. Lang, Z. Y. W. Lin, R. Zheng, Y. Zhang, W. Cui, N. Annabi and A. Khademhosseini, Acta Biomater., 2017, 49, 66–77 CrossRef CAS PubMed.
- J. W. Nichol, S. T. Koshy, H. Bae, C. M. Hwang, S. Yamanlar and A. Khademhosseini, Biomaterials, 2010, 31, 5536–5544 CrossRef CAS PubMed.
- L. Wang, Y. Wu, T. Hu, P. X. Ma and B. Guo, Acta Biomater., 2019, 96, 175–187 CrossRef CAS PubMed.
Footnote |
† These authors contributed equally to this work. |
|
This journal is © The Royal Society of Chemistry 2023 |
Click here to see how this site uses Cookies. View our privacy policy here.