DOI:
10.1039/D2BM01455F
(Paper)
Biomater. Sci., 2023,
11, 119-127
Oxygen self-supplied upconversion nanoplatform loading cerium oxide for amplified photodynamic therapy of hypoxic tumors†
Received
7th September 2022
, Accepted 31st October 2022
First published on 4th November 2022
Abstract
Photodynamic therapy (PDT) has been widely used in preclinical trials for treating various tumors. However, the hypoxic environment of tumors and the limited penetration depth of ultraviolet light severely weaken the PDT effect. To solve the above problems, a near-infrared (NIR) light-triggered oxygen (O2) self-supplied phototherapeutic platform (UCNPs/CeO2/Ce6/BSA) for amplified PDT performance against solid tumors by alleviating tumor hypoxia has been rationally developed. The platform has excellent stability and can continuously decompose H2O2 for sustained O2 supply to synergize 1O2 generation, thus inducing an enhanced mortality rate (59%) of ID8 cells in vitro under hypoxic + H2O2 conditions. The growth of solid tumors was effectively inhibited and the mouse survival rate was dramatically enhanced via a superior PDT therapeutic performance. This reported study facilitated the positive development of multifunctional diagnosis and treatment platforms under long-wavelength excitation for O2 self-supplied tumor treatments.
Introduction
Photoinduced tumor therapy, especially photodynamic therapy (PDT), has obtained a rising focus in preclinical trials owing to its easy operation and desirable therapeutic efficacy.1–3 In PDT, photosensitizers (PSs) can translate adjacent substrates into reactive oxygen species (ROS), which can generate oxidant stress for ablating tumor cells under irradiation.4–6 In general, ideal PSs, light, and an abundant oxygen (O2) supply are necessary for superior PDT.7 Though the heavy atom effect, polymerization, and fluorescence resonance energy transfer have indeed improved the 1O2 generation capability of PSs for excellent therapeutic performance, the poor oxygen supply in tumors, limited tissue penetration of light, and the “always-on” mode have significantly hindered their potential clinical applications.8–11 Therefore, it is of great importance to rationally develop a near-infrared (NIR) light-triggered phototherapeutic platform with synergized or activable features for superior outcomes in vitro and in vivo.
To achieve superior therapeutic performance, recently, various PSs (e.g. metal–organic frameworks, transition metal–oxygen/sulfides, and organic semiconductors) with superior enhanced permeability and retention (EPR) effects have been developed for NIR light-regulated PDT.12–15 Among them, upconversion nanoparticle (UCNP)-based phototherapeutic agents have been widely utilized as NIR light-triggered precursors of PSs for phototherapy owing to their capability of transforming NIR light into visible/ultraviolet light.16–18 In addition, hydrogen peroxide (H2O2) was overproduced at the tumor site due to the abnormal metabolism of tumor cells.19–21 Besides, H2O2 could be decomposed by an enzyme-like catalyst (e.g. cerium oxide [CeO2]) to produce O2.22–24 Therefore, the integration of UCNPs and enzyme-like catalysts into a multifunctional phototherapeutic platform contributed to synergizing NIR light-regulated PDT for superior tumor therapeutic performance.
Taking the above into consideration, herein a NIR light-triggered phototherapeutic platform (UCNPs/CeO2/Ce6/BSA) integrating UCNPs, CeO2, and Ce6 was rationally developed for synergizing PDT performance against solid tumors (Scheme 1). Enriched O2 can be obtained by decomposing H2O2via CeO2 in UCNPs/CeO2/Ce6/BSA, which can be transformed into 1O2 by Ce6 upon excitation by luminous resonance energy transfer (LRET) from UCNPs under NIR light. UCNPs/CeO2/Ce6/BSA achieved superior stability and water dispersion, excellent EPR effects, and 1O2 generation capability under hypoxic conditions in vitro/in vivo, thus producing a satisfactory PDT effect on solid tumors and enhancing mouse survival rates under the guidance of fluorescence imaging (FI). Hence, UCNPs/CeO2/Ce6/BSA showed promising prospects as a phototherapeutic platform for preclinical trials. Besides, the strategies reported in the study could be expanded to the rational development of NIR light-regulated phototherapeutic platforms based on UCNPs for O2 self-supplied PDT.
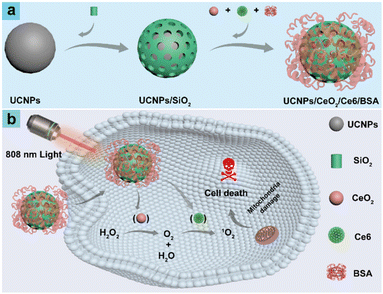 |
| Scheme 1 Synthetic route of the phototherapeutic platform and its enhanced PDT performance. (a) Synthetic route of the phototherapeutic platform (UCNPs/CeO2/Ce6/BSA). (b) Mechanism of enhanced PDT against solid tumor. | |
Results and discussion
Synthesis and characterization of UCNPs/CeO2/Ce6/BSA
The preparations of mesoporous silica-coated UCNPs (UCNPs@SiO2) and the final UCNPs/CeO2/Ce6/BSA are shown in Scheme 1a. As depicted, stable core–shell structured UCNPs were first obtained by the thermal decomposition method according to previous reports.25–27 Their hexagonal-phase structures were firmly confirmed by TEM images (Fig. 1a). UCNPs@mSiO2 was then acquired by coating UCNPs with mesoporous silica (SiO2), which was confirmed by TEM (Fig. 1a). Subsequently, CeO2 was loaded by adding Ce(NO3)3·6H2O under base conditions at 80 °C. Commercial dyes Ce6 as PSs and fluorescent imaging contrast agents were further introduced and subsequent BSA encapsulation formed hydrophilic UCNPs/CeO2/Ce6/BSA nanoparticles. UCNPs/Ce6/BSA nanoparticles without CeO2 served as the control. As shown in Fig. 1a, the sizes of the resulting UCNPs, UCNPs/SiO2, UCNPs/Ce6/BSA, and UCNPs/CeO2/Ce6/BSA nanoparticles were 23 nm, 41 nm, 48 nm, and 52 nm in turn, respectively. The successful introduction of Ce into UCNPs/CeO2/Ce6/BSA could be confirmed by elemental analysis (Fig. 1b). The hydrodynamic diameters of UCNPs, UCNPs/SiO2, UCNPs/Ce6/BSA, and UCNPs/CeO2/Ce6/BSA nanoparticles were 35 nm, 57 nm, 69 nm, and 76 nm (Fig. S1†), which were bigger than the corresponding TEM results, respectively. The above result was attributed to the swelling of nanoparticles in solutions. Besides, the hydrodynamic sizes of UCNPs/Ce6/BSA and UCNPs/CeO2/Ce6/BSA contributed to fulfilling their passive tumor targeting capabilities through EPR effects.28,29 Besides, the hydrodynamic sizes of UCNPs/Ce6/BSA and UCNPs/CeO2/Ce6/BSA showcased a negligible change, and the nanoparticle solutions were transparent in BSA for one week (Fig. S2†), indicating their superior structural stability and biocompatibilities. As shown in Fig. 1c and S3,† the absorption peaks of UCNPs/Ce6/BSA and UCNPs/CeO2/Ce6/BSA matched well with that of Ce6/BSA, manifesting the successful loading of Ce6. The corresponding loading rates of Ce6 were 0.6 and 0.4 wt%, as confirmed by the standard absorption curve (Fig. S4†), respectively. Ce6 showed a large absorption cross-section, which matched well with the emission peaks of UCNPs at 657 nm (Fig. 1c and S3†), which could promote luminous resonance energy transfer (LRET) from UCNPs to Ce6. In addition, UCNPs/Ce6/BSA and UCNPs/CeO2/Ce6/BSA showed decreased emission intensities at 657 nm compared to that of UCNPs under the same exciting conditions, demonstrating the above effective LRET to excite Ce6 under a NIR (808 nm) light.
 |
| Fig. 1 Photophysicochemical properties of UCNPs/CeO2/Ce6/BSA. (a) TEM images of UCNPs, UCNPs/SiO2, UCNPs/Ce6/BSA, and UCNPs/CeO2/Ce6/BSA. (b) Photographs of elemental mapping corresponding to the high-resolution TEM image of a single UCNPs/CeO2/Ce6/BSA nanoparticle. (c) Absorption and emission spectra of samples. (d) O2 production capabilities of samples in the presence of H2O2. (e) A/A0vs. irradiation time. A0 and A are the maximal absorbances of ABDA + H2O2 or its mixture with Ce6/BSA, UCNPs/Ce6/BSA, and UCNPs/CeO2/Ce6/BSA in PBS before and after irradiation. | |
1O2 generation capabilities
CeO2 is a kind of efficient enzyme-like catalyst. It can decompose H2O2 to produce O2.30–33 The O2 generation capabilities of the samples were then investigated. A portable dissolved oxygen meter was used to monitor the concentration of dissolved O2 transformed from H2O2 by samples in 100 μM H2O2 solution referring to the previous report.34 UCNPs/Ce6/BSA without CeO2 served as the control. As shown in Fig. 1d, the dissolved O2 showed a slight increase in H2O2 solution or its mixtures with UCNPs/Ce6/BSA solutions, which was attributed to the decomposition of a few H2O2 molecules, while the UCNPs/CeO2/Ce6/BSA and H2O2 mixtures exhibited higher O2 concentration increases with time, indicating that UCNPs/CeO2/Ce6/BSA can decompose H2O2 to produce O2 for constant oxygen supply over time, which showed a promising potential to synergize PDT performance on solid tumors under hypoxic conditions. The 1O2 generation capacities of samples were then evaluated by comparing the characteristic absorption of the singlet oxygen probe (ABDA) at 400 nm under irradiation (808 nm). As displayed in Fig. 1e and S5,† under irradiation + H2O2 conditions, the absorption of ABDA or its mixture with Ce6/BSA at 400 nm displayed almost no decrease, demonstrating negligible 1O2 generation. Upon comparison, the mixture of UCNPs/CeO2/Ce6/BSA or UCNPs/Ce6/BSA and ABDA showcased decreased absorption at 400 nm, and the degree of decrease of the former was more obvious compared to that of the latter, suggesting the better 1O2 generation performance of UCNPs/CeO2/Ce6/BSA compared to UCNPs/Ce6/BSA. Besides, the mixture of UCNPs/CeO2/Ce6/BSA or UCNPs/Ce6/BSA and ABDA showcased a similar decreasing trend in absorption at 400 nm under irradiation without H2O2 conditions. These results show the promising potential of UCNPs/CeO2/Ce6/BSA to produce enriched 1O2 in solid tumors for amplifying PDT performance.
In vitro PDT
Next, the cytotoxicities of the samples were investigated. The low potential cytotoxicities of UCNPs/Ce6/BSA and UCNPs/CeO2/Ce6/BSA towards tumor cells (ID8) and normal cells (BSC-1) were first confirmed using a CCK-8 assay (Fig. S6†). The ROS production capabilities of the samples in ID8 cells were then explored by confocal laser scanning microscopy (CLSM) imaging. 2′,7′-Dichlorofluorescin diacetate (DCFH-DA) can be transformed into dichlorofluorescein (DCF) with a green fluorescence by ROS. Thus, it was utilized to assess the ROS generation capability of the samples in ID8 cells by comparing the green fluorescence intensities of ID8 cells. ID8 cells treated with PBS + light, DCFH-DA + light, UCNPs/CeO2/Ce6/BSA, or UCNPs/Ce6/BSA showcased weak or negligible green fluorescence (Fig. 2a and S7†) under normoxic and hypoxic conditions, indicating that these treatments made no difference to ROS generation. In stark contrast, under light irradiation, similar strong green fluorescence could be observed in ID8 cells with treatment with DCFH-DA + UCNPs/CeO2/Ce6/BSA or UCNPs/Ce6/BSA under normoxic conditions. In contrast, ID8 cells incubated with DCFH-DA plus UCNPs/Ce6/BSA or UCNPs/CeO2/Ce6/BSA represented weaker and stronger green fluorescence under H2O2 + hypoxic conditions, respectively. These results manifested the excellent ROS generation capabilities of UCNPs/CeO2/Ce6/BSA and UCNPs/Ce6/BSA in ID8 cells under normoxic conditions. However, the hypoxic conditions can greatly weaken the ROS generation performance of UCNPs/Ce6/BSA. Fortunately, the introduction of CeO2 can effectively enhance the O2 concentration by decomposing H2O2 to enhance the ROS generation performance of UCNPs/CeO2/Ce6/BSA. To further confirm the excellent ROS generation performance of UCNPs/CeO2/Ce6/BSA under hypoxic conditions, ID8 cells were treated with DCFH-DA plus UCNPs/CeO2/Ce6/BSA or UCNPs/Ce6/BSA under normoxic and hypoxic conditions, respectively. The maximal emission intensity of DCF was monitored to value the ROS generation capabilities of the samples in vitro after DCFH-DA was oxidized by ROS. As shown in Fig. 2b, under normoxic conditions, ID8 cells treated with DCFH-DA plus UCNPs/CeO2/Ce6/BSA or UCNPs/Ce6/BSA showcased brighter and similar fluorescence intensities compared to those treated with UCNPs/CeO2/Ce6/BSA without irradiation. Under H2O2 + hypoxic conditions, ID8 cells treated with UCNPs/Ce6/BSA + irradiation exhibited a slightly higher fluorescence intensity compared to those treated with UCNPs/CeO2/Ce6/BSA without irradiation but obviously lower than those treated with UCNPs/CeO2/Ce6/BSA + irradiation. These results further confirmed that the introduction of CeO2 can effectively enhance O2 concentration by decomposing H2O2 for enhancing the ROS generation performance of UCNPs/CeO2/Ce6/BSA.
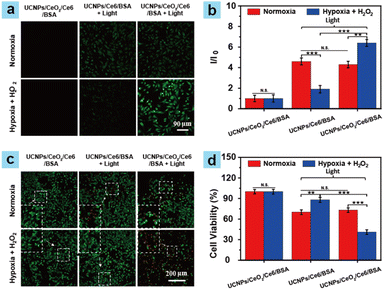 |
| Fig. 2
1O2 production and PDT capabilities of samples in vitro. (a) CLSM imaging of ID8 cells with different treatments for 1O2 generation. (b) I/I0 is the rate of emission intensity at 529 nm. I0 and I are the emission intensities at 529 nm of DCF in ID8 cells treated with UCNPs/CeO2/Ce6/BSA only and UCNPs/Ce6/BSA or UCNPs/CeO2/Ce6/BSA plus light, respectively. (c) CLSM imaging and (d) CCK-8 experiments of ID8 cells for evaluating the PDT capabilities of UCNPs/CeO2/Ce6/BSA and UCNPs/Ce6/BSA on ID8 cells with different treatments, respectively. **P < 0.01 and ***P < 0.001. | |
We then explored the PDT performance of the samples (UCNPs/CeO2/Ce6/BSA and UCNPs/Ce6/BSA) on ID8 cells using CLSM imaging. Live (green) and dead (red) ID8 cells were stained via calcein-AM and PI. ID8 cells were treated with UCNPs/CeO2/Ce6/BSA, UCNPs/CeO2/Ce6/BSA + light, and UCNPs/Ce6/BSA + light under normoxic and hypoxic conditions, respectively. ID8 cells incubated with samples without light irradiation served as the control. As shown in Fig. 2c, ID8 cells in control groups showed intense green luminescence under hypoxic or normoxic conditions, indicating almost no dead ID8 cells, further confirming the negligible dark cytotoxicity of samples to ID8 cells. Under normoxia and irradiation conditions, ID8 cells exhibited similar red and green luminescence after they were treated with UCNPs/CeO2/Ce6/BSA and UCNPs/Ce6/BSA, respectively, demonstrating that UCNPs/CeO2/Ce6/BSA and UCNPs/Ce6/BSA exhibit excellent PDT performance on ID8 cells under normoxic conditions. However, under hypoxic + H2O2 + irradiation conditions, ID8 cells treated with UCNPs/Ce6/BSA displayed more green luminescence but less red luminescence compared to those treated with UCNPs/CeO2/Ce6/BSA, suggesting that UCNPs/CeO2/Ce6/BSA still presented superior PDT capabilities even under hypoxic conditions, which was attributed to the fact that UCNPs/CeO2/Ce6/BSA (CeO2) can decompose H2O2 and produce abundant O2 for superior PDT performance. To further demonstrate the superior PDT performance of UCNPs/CeO2/Ce6/BSA under hypoxic conditions, we further performed a CCK-8 assay to evaluate the PDT performance under the same conditions as above. As shown in Fig. 2d, ID8 cells showcased a high cell viability of about 100% after they were treated with the samples only; the viabilities of ID8 cells were 73% and 70% after treatment with UCNPs/CeO2/Ce6/BSA and UCNPs/Ce6/BSA under normoxic conditions, respectively. However, the viabilities of ID8 cells were 88% and 41% after treatments with UCNPs/Ce6/BSA and UCNPs/CeO2/Ce6/BSA under H2O2 plus hypoxic conditions, respectively, which matched well with their flow cytometric analysis (Fig. S8†). These results collectively confirmed the excellent PDT performance of UCNPs/CeO2/Ce6/BSA and UCNPs/Ce6/BSA under normoxic conditions. In particular, UCNPs/CeO2/Ce6/BSA still represented superior PDT effects under hypoxic conditions, which were well in line with their excellent 1O2 generation performance in ID8 cells, further demonstrating that UCNPs/CeO2/Ce6/BSA showed a great potential to relieve tumor hypoxia for enhancing tumor PDT performance.
Pharmacokinetic study
The superior PDT performance results of UCNPs/CeO2/Ce6/BSA in ID8 cells under hypoxic conditions inspired us to implement tumor therapeutic experiments in vivo. Before the tumor therapy tests, pharmacokinetic exploration of the samples was first carried out because it showed great importance in assessing tumor PDT performance.35 The maximal absorption change of samples was used to explore the dose of samples in mouse blood after their intravenous injection at different time points. As shown in Fig. 3a, the maximal absorbance values of UCNPs/CeO2/Ce6/BSA and UCNPs/Ce6/BSA rapidly decreased. Less than 10% of the samples were left after 4 h of the intravenous injection of samples. Finally, UCNPs/CeO2/Ce6/BSA and UCNPs/Ce6/BSA exhibited short half-lives of 0.84 h and 0.68 h, respectively, which were attributed to their small sizes, indicating that the prepared samples (UCNPs/CeO2/Ce6/BSA and UCNPs/Ce6/BSA) could be rapidly metabolized from blood.
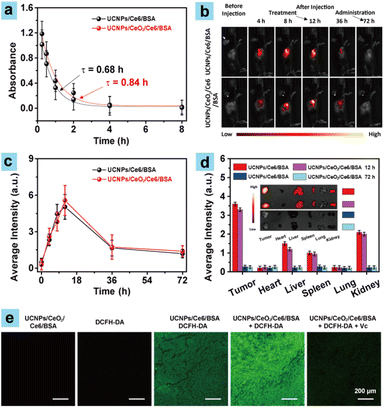 |
| Fig. 3 Pharmacokinetic study and FI of mice. (a) The concentration of samples (UCNPs/Ce6/BSA and UCNPs/CeO2/Ce6/BSA) in mouse blood was recorded by monitoring the absorption of samples in the serum after injection over time. (b) Fluorescence intensity images of mice with ID8 tumors before and after the injection of UCNPs/Ce6/BSA and UCNPs/CeO2/Ce6/BSA, respectively. (c) Average fluorescence intensity of mouse tumors after the injection of UCNPs/Ce6/BSA and UCNPs/CeO2/Ce6/BSA over time. (d) The average fluorescence intensity of mouse tumors and major organs after the injection of UCNPs/Ce6/BSA and UCNPs/CeO2/Ce6/BSA for 12 h and 72 h, respectively. Inset: fluorescence intensity photos of mouse tumors and major organs (λEx = 660 nm). (e) CLSM imaging of tumor slices from mice after various treatments. | |
In vivo fluorescence imaging
In addition, UCNPs/CeO2/Ce6/BSA and UCNPs/Ce6/BSA showed excellent concentration-dependent fluorescence signals (Fig. S9†), respectively, owing to Ce6 dye being included as a fluorescence imaging contrast agent, which helped to monitor the accumulation of samples at the tumor site. As shown in Fig. 3b and c, the fluorescence signal of the mouse tumor parts increased over time and reached its brightest level after 12 h of the intravenous injections of the samples, manifesting that abundant samples accumulated at the tumor site owing to the excellent tumor passive targeting capabilities of the samples by EPR effects. Finally, negligible fluorescence signals could be observed after 72 h of injection, indicating that the samples can be metabolized from the tumors. We then further recorded the distribution of samples in vivo by monitoring the fluorescence signal of the tumors and major organs after UCNPs/CeO2/Ce6/BSA and UCNPs/Ce6/BSA were intravenously injected for 12 h and 72 h because nanoparticles of large size tend to accumulate in tissues with reticular structures, such as the liver and kidneys.36 As shown in Fig. 3d, the tumor, liver, spleen, and kidneys showed intense fluorescence signals, indicating excellent accumulation of samples (UCNPs/CeO2/Ce6/BSA and UCNPs/Ce6/BSA) there 12 h after the intravenous injections. In addition, the tumors exhibited the brightest fluorescence intensity, suggesting more samples enriched these organs compared to other major organs. After 72 h, a negligible fluorescence signal could be observed, showing that samples could be metabolized from tumors and major organs, which contributed to reducing the biological toxicity of samples during the PDT process. These results contributed to guiding the following tumor PDT tests.
In vivo ROS generation
To demonstrate the enhanced ROS generation capabilities of UCNPs/CeO2/Ce6/BSA in tumors during the PDT process, CLSM imaging of tumor sections was carried out. After UCNPs/CeO2/Ce6/BSA, UCNPs/Ce6/BSA or PBS were intravenously injected for 11.5 h, DCFH-DA was intratumorally injected or not. Tumors were then treated with irradiation or not after another 0.5 h. As displayed in Fig. 3e, tumor slices showcased no green fluorescence after intravenous injection with UCNPs/CeO2/Ce6/BSA only owing to the absence of DCFH-DA. The tumor slices of a mouse with DCFH-DA intratumoral injection displayed weak green fluorescence, which was attributed to few ROS present in the tumor cells. In striking contrast, the tumor slices exhibited distinctly enhanced fluorescence signals after the mice were treated with UCNPs/CeO2/Ce6/BAS + DCFH-DA + light and UCNPs/Ce6/BAS + DCFH-DA + light, respectively. In particular, the former showed brighter fluorescence, showing that the former treatment gave a greater effect for ROS generation in vivo compared to that of the latter, demonstrating that UCNPs/CeO2/Ce6/BSA could produce more ROS for promising enhanced PDT performance on solid tumors because UCNPs/CeO2/Ce6/BSA (CeO2) can decompose H2O2 to produce abundant O2. These results were well in line with the hypoxic immunofluorescence results for tumor slices (Fig. S10†).
In vivo PDT
Motivated by the superior EPR effects and the enhanced ROS generation performance of UCNPs/CeO2/Ce6/BSA in solid tumors, PDT tests on C57BL/6 mice with ID8 tumors were then implemented. 30 mice were randomly distributed into five groups (six mice each) which went through the following treatments: (I) PBS, (II) UCNPs/CeO2/Ce6/BSA, (III) light, (IV) UCNPs/Ce6/BSA + light, and (V) UCNPs/CeO2/Ce6/BSA + light, respectively. Referring to the metabolism of UCNPs/CeO2/Ce6/BSA in vivo, mouse weight, tumor volume, and mouse survival rate were recorded and the tumors were treated once every three days, respectively. Mouse survival rate was a considerably important index to assess PDT performance, and the survival rate curve was constructed by considering mice with a tumor size greater than 1 cm to be dead. Mice in all groups showcased a similar change in trends for body weight (Fig. S11†), manifesting negligible side effects of the treatments including irradiation, UCNPs/Ce6/BSA, and UCNPs/CeO2/Ce6/BSA to mice in the PDT process. As shown in Fig. 4a, mouse tumors in all groups showed an increasing trend over time. In particular, mouse tumors in groups I–III exhibited a dramatic increase. Finally, their sizes were about 10 times the starting value, manifesting that light irradiation or UCNPs/CeO2/Ce6/BSA made no difference to tumor volume. Group IV and especially group V represented much slower tumor volume growth compared to the above groups. Their final sizes were about 6 and 4 times the original ones, respectively. Finally, the average tumor weight in groups I–III was about 1.6 and 3.0 times that of groups IV and V (Fig. 4b), respectively. These results resulted from the excellent ROS generation performance of UCNPs/Ce6/BSA and UCNPs/CeO2/Ce6/BSA against solid tumors; especially, the latter showed better performance because CeO2 can decompose H2O2 in tumor parts and produce O2 to alleviate tumor hypoxia for amplifying the PDT of solid tumors. Besides, the mouse survival rate in groups I–III began to decrease after treatment for 10 d, and no mouse survived after treatment for 33 d. In comparison, mice in group IV began to die after treatment for 24 d. However, group IV and especially group V showed high survival rates of 67% and 100% after final PDT (Fig. 4c), respectively. These results demonstrated that UCNPs/Ce6/BSA and especially UCNPs/CeO2/Ce6/BSA displayed excellent PDT performance in inhibiting tumor growth and improving the mouse survival rate.
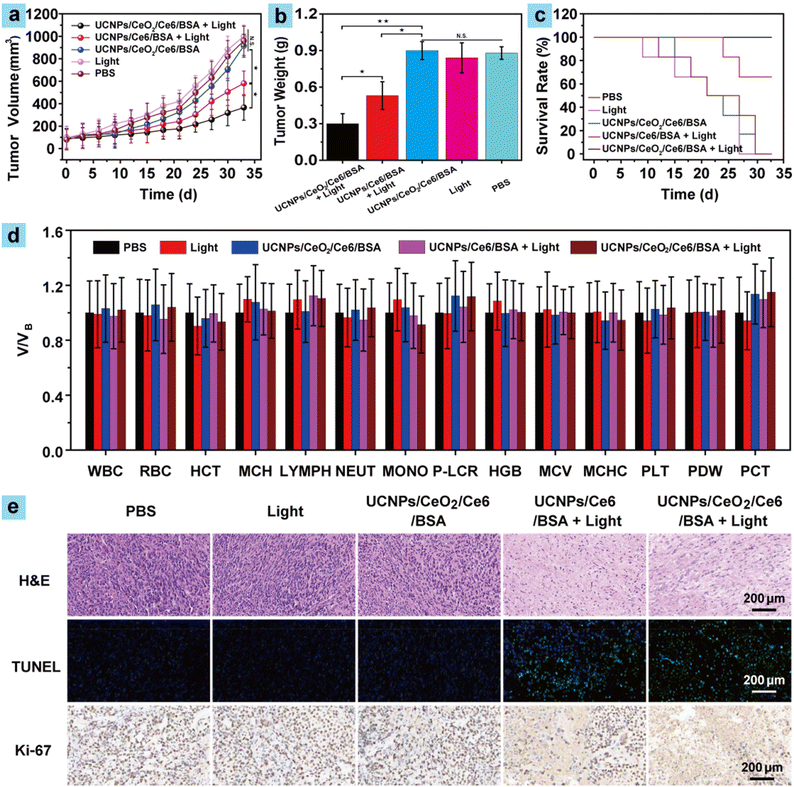 |
| Fig. 4
In vivo PDT. (a) Tumor volume, (b) tumor weight, (c) mice survival, (d) routine blood analysis, and (e) tumor section staining (including H&E, Ki-67, and TUNEL) after mice treated with PBS, light, UCNPs/CeO2/Ce6/BSA, UCNPs/Ce6/BSA + light, and UCNPs/CeO2/Ce6/BSA + light for 33 d, respectively. *P < 0.05, **P < 0.01 and ***P < 0.001. | |
Routine blood analysis and immunohistochemical evaluations
Blood routine analysis and immunohistochemical evaluations including H&E, Ki-67, and/or TUNEL staining of major organs and tumors were then investigated to further assess the systemic toxicity of all treatments and the excellent therapeutic efficiency of samples after the mice went through treatments for 33 d. The key parameters of blood, including lymphocytes (LYMPH), white blood cells (WBC), platelets (PLT), etc., exhibited no obvious statistical distribution differences in all groups (Fig. 4d), showing the negligible blood toxicity of the samples and the effect of various treatments in the PDT process. In addition, H&E staining imaging of all major organs and H&E, Ki-67, and TUNEL staining of tumors in groups I–III showed no apoptotic and necrotic tumor cells, indicating that these treatments did not affect the major organs of mice in the experiment (Fig. 4e and S12†). Besides, large amounts of apoptotic and necrotic tumor cells could be observed in groups IV and V from H&E, Ki-67, and TUNEL staining imaging (Fig. 4e), suggesting the excellent therapeutic efficiency of the samples. All these results firmly confirmed that UCNPs/Ce6/BSA and UCNPs/CeO2/Ce6/BSA showcased negligible blood toxicity and excellent PDT performance, thus demonstrating their promising potential for biological applications.
Conclusions
In summary, a NIR light-regulated phototherapeutic platform (UCNPs/CeO2/Ce6/BSA) integrating UCNPs, CeO2, and Ce6 was rationally designed for enhancing the PDT effects on solid tumors. UCNPs/CeO2/Ce6/BSA (CeO2) can decompose H2O2 to produce abundant O2, which could be changed into 1O2 under NIR light. UCNPs/CeO2/Ce6/BSA possess excellent stability and water dispersion, EPR effects, and superior 1O2 generation capabilities under normoxic/hypoxic conditions in vitro/in vivo, and thus result in amplified PDT performance against solid tumors and improved mouse survival rates under the guidance of fluorescence imaging. In addition, routine blood analysis and H&E, Ki-67, and TUNEL staining tests further firmly demonstrated the negligible blood toxicity and excellent PDT performance of UCNPs/CeO2/Ce6/BSA, thus, confirming their promising potential as a phototherapeutic platform for preclinical trials. The strategies reported in the exploration can be expanded to the rational development of NIR light-regulated multimodal phototherapeutic platforms (including, PDT, photothermal therapy, gas therapy, and chemotherapy) to facilitate the positive development of O2 self-supplied phototherapy.
Experimental
Materials and characterization
Related chemicals and solvents were used directly without further purification. Er(CH3CO2)3, propidium iodide (PI), oleic acid (OA), 1-octadecane (ODE), calcein-acetyl-methoxylated (Calcein-AM), cetyltrimethylammonium bromide (CTAB), Nd(CH3CO2)3, Yb(CH3CO2)3, and Y(CH3CO2)3 were supplied by Sigma-Aldrich. NH4F and tetraethyl orthosilicate (TEOS) were supplied by Aladdin. TEM and dynamic light scattering (DLS) were utilized to acquire particle morphology and size, respectively. An Edinburgh FL 920 spectrophotometer and a ultraviolet-visible-NIR spectrophotometer were utilized to obtain the emission and absorption spectra, respectively. A CLSM was utilized to obtain the confocal images of cells and slices. A small animal living fluorescence imaging (FI) system was used to obtain the FI images of the samples and mice.
Generation of O2 from H2O2
To evaluate the capabilities of the samples to decompose H2O2 and to produce O2, 200 μL of UCNPs/CeO2/Ce6/BSA (300 μg mL−1) was added to 5 mL of H2O2 solution (100 μM). Then, a portable dissolved oxygen meter (JPB-607A) was used to record the amount of increase of O2.
1O2 generation properties of UCNPs/CeO2/Ce6/BSA
Under irradiation (808 nm, 1.0 W cm−2) + H2O2 (100 μM) conditions, the characteristic absorbance of ABDA or its mixture with UCNPs/CeO2/Ce6/BSA (300 μg mL−1), UCNPs/Ce6/BSA (300 μg mL−1) or Ce6/BSA in PBS was recorded over time.
FI signals of UCNPs/CeO2/Ce6/BSA
Concentration-dependent FI signals of UCNPs/CeO2/Ce6/BSA and UCNPs/Ce6/BSA were measured in the point-to-point way. Briefly, 96-well plates filled with UCNPs/CeO2/Ce6/BSA and UCNPs/Ce6/BSA with various concentrations were used for FI measurement (λex = 660 nm). The region of interest was used to evaluate the average signal intensities of the samples, respectively.
Cell viability assay
The cytotoxicities of UCNPs/CeO2/Ce6/BSA and UCNPs/Ce6/BSA in BSC-1 and ID8 cells were measured through methyl thiazolyl tetrazolium tests. The cells were treated with UCNPs/CeO2/Ce6/BSA and UCNPs/Ce6/BSA of different concentrations for 24 h. CCK-8 was then dropped and incubated for 4 h. DMSO was dropped and OD570 was collected. In addition, under normoxic or hypoxic + H2O2 (100 μM) conditions, ID8 cells were treated with UCNPs/CeO2/Ce6/BSA (300 μg mL−1), UCNPs/Ce6/BSA (300 μg mL−1) + light (808 nm, 1.0 W cm−2, 10 min), and UCNPs/CeO2/Ce6/BSA + light. Then, the viabilities of ID8 cells were collected according to the above operation to assess PDT performance.
In vitro
1O2 detection
Under normoxic or hypoxic + H2O2 (100 μM) conditions, ID8 cells were incubated with UCNPs/Ce6/BSA (300 μg mL−1), UCNPs/CeO2/Ce6/BSA (300 μg mL−1) or PBS for 4 h, respectively. DCFH-DA was added to ID8 cells and incubated for another 15 min, accompanying irradiation for 10 min. They were then used for CLSM imaging (λex = 488 nm).
Animals and tumor models
All animal procedures were performed in accordance with the Guidelines for Care and Use of Laboratory Animals of Shenzhen University and approved by the Animal Ethics Committee of Shenzhen University. The Medical Animal Laboratory Center of Guangdong Province offered five-week-old female C57BL/6 mice. ID8 cells (5 × 107) were subcutaneously injected to obtain tumor models.
In vivo FI
ID8 tumor-bearing C57BL/6 mice (about 200 mm3) were chosen to perform FI tests after the samples (UCNPs/CeO2/Ce6/BSA, 150 μL, 1.5 mg mL−1 or UCNPs/Ce6/BSA, 150 μL, 1.5 mg mL−1) were intravenously injected over time, respectively.
In vivo
1O2 detection
To demonstrate the 1O2 generation capabilities of the samples (UCNPs/CeO2/Ce6/BSA or UCNPs/Ce6/BSA) in tumors, UCNPs/CeO2/Ce6/BSA (150 μL, 1.5 mg mL−1) and UCNPs/Ce6/BSA (150 μL, 1.5 mg mL−1) were intravenously injected. After 11.5 h, 40 μmol kg−1 DCFH-DA was intratumorally injected, accompanying irradiation (808 nm, 1.0 W cm−2, 10 min) after a further 0.5 h. Tumor sections (about 30 μm thickness) from the above mice were used for CLSM imaging.
In vivo PDT
C57BL/6 mice with ID8 tumors (about 100 mm3) were distributed into five groups. The above mice went through the following treatments: (I) PBS, (II) UCNPs/CeO2/Ce6/BSA (150 μL, 1.5 mg mL−1), (III) light (808 nm, 1.0 W cm−2, 10 min), (IV) UCNPs/Ce6/BSA (150 μL, 1.5 mg mL−1) + light, and (V) UCNPs/CeO2/Ce6/BSA + light. The mouse survival rate, tumor size, and mouse weight were recorded, and PDT was performed every three days. Routine blood analysis and immunohistochemistry analysis (such as H&E, Ki-67, or TUNEL staining) were carried out after treatments for 33 d.
Synthesis of NaYF4:Yb(18%),Er(2%)
0.78 mmol Y(CH3CO2)3, 12 ml OA, 0.18 mmol Yb(CH3CO2)3, 18 ml of ODE, and Er(CH3CO2)3 (0.02 mmol) were added to a flask (100 mL). Oxygen and the low-boiling-point components were removed under vacuum and high-temperature (150 °C) conditions for 30 min. A mixture solution of NH4F (2.5 mmol) and NaOH (4 mmol) in methanol (10 ml) was then dropped into the above solution at room temperature. Methanol was then evaporated (100 °C, 30 min). The as-prepared solution was then kept at 300 °C for 1 h. The NaYF4:Yb(18%) and Er(2%) solutions were obtained after the above mixture was centrifuged, washed, cooled, and dispersed in cyclohexane.
Preparation of NaYF4:Yb,Er@NaYF4:Nd(20%) (UCNPs)
The mixture of Y(CH3CO2)3 (0.80 mmol), OA(12 ml), Nd(CH3CO2)3 (0.20 mmol), and ODE (18 ml) was poured into a flask (100 mL). Oxygen and the low-boiling-point components were removed under vacuum and high-temperature (150 °C) conditions for 30 min. Subsequently, NaYF4:Yb(18%), Er(2%) (10 mL) and the mixture of NaOH (4 mmol) and NH4F (2.5 mmol) in methanol (10 mL) were poured into the above system in turn. Methanol was then evaporated (100 °C, 30 min). The as-prepared solution was then kept at 300 °C for 1 h. UCNP solution was obtained after the above mixture was centrifuged, washed cooled, and dispersed in cyclohexane.
Synthesis of NaYF4:Yb, Er@NaYF4:Nd@mSiO2 (UCNPs@mSiO2) nanoparticles
The obtained UCNPs in chloroform (0.5 mL) were added to a CTAB solution (0.2 M, 5 mL) under vigorous ultrasound. The as-prepared mixture was then stirred (70 °C, 30 min). Pure water (5 ml) was then poured into the mixture (5 ml) at 40 °C. NaOH solution (0.1 M, 150 μL) was then poured and stirred (5 min). TEOS in ethanol (150 μL, 20%) was then dropped and stirred heavily (40 °C, 24 h). The UCNPs@mSiO2 solution was obtained after the above mixture was washed twice with ethanol and extracted for 3 h by sodium chloride (1 wt%) solution in methanol.
Synthesis of UCNPs/CeO2/Ce6/BSA
BSA (30 mg) was dissolved in a UCNPs@mSiO2 solution (3 mL). Cerium nitrate hexahydrate solution (63 μL, 0.1 mol L−1) was added and stirred (80 °C, 15 min). Then, a NaOH solution (120 μL, 0.5 mol L−1) was quickly dropped and stirred (80 °C, 2 h). Ce6 (0.5 mg) in DMSO (1 mL) was added to the above mixture (or UCNPs@mSiO2 solution + 30 mg BSA or deionized water + 30 mg BSA) under ultrasound for 10 min. The as-prepared mixture was then treated with a dialysis bag (8–14 kDa) for 5 h. UCNPs/CeO2/Ce6/BSA, UCNPs/Ce6/BSA, and Ce6/BSA were obtained for the following tests. The loading capacity (η) of Ce6 was quantitatively calculated according to the following equation: η(%) = (weight of loaded Ce6)/(weight of UCNPs/(CeO2/)Ce6/BSA) × 100%.
Author contributions
Yunjian Xu: Conceptualization, methodology, investigation, data curation, validation, formal analysis, writing – original draft, and writing – reviewing & editing. Kexin Wang: Conceptualization, methodology, investigation, data curation, formal analysis, and writing – original draft. Zhenjiang Chen: Investigation and formal analysis. Rui Hu: Investigation and formal analysis. Yihua Zhao: Investigation and formal analysis. Xiang Li: Investigation and formal analysis. Junle Qu: Visualization, writing – review & editing, and funding acquisition. Liwei Liu: Conceptualization, supervision, visualization, writing – review & editing, and funding acquisition.
Conflicts of interest
There are no conflicts to declare.
Acknowledgements
This work was supported in part by The National Natural Science Foundation of China (61961136005, 61835009, 61935012, 62127819, and 62175163), the Shenzhen Key Projects (JCYJ20200109105404067), and the Shenzhen International Cooperation Project (GJHZ20190822095420249).
References
- Y. Xu, T. Feng, T. Yang, H. Wei, H. Yang, G. Li, M. Zhao, S. Liu, W. Huang and Q. Zhao, ACS Appl. Mater. Interfaces, 2018, 10, 16299–16307 CrossRef CAS.
- Y. Xu, S. Wang, Z. Chen, R. Hu, S. Li, Y. Zhao, L. Liu and J. Qu, J. Nanobiotechnol., 2021, 19, 37 CrossRef CAS PubMed.
- Y. Xu, S. Wang, Z. Chen, R. Hu, Y. Zhao, K. Wang, J. Qu and L. Liu, Biomaterials, 2021, 276, 121017 CrossRef CAS.
- K. Chen, P. He, Z. Wang and B. Tang, ACS Nano, 2021, 15(4), 7735–7743 CrossRef CAS PubMed.
- G. T. Nash, T. Luo, G. Lan, K. Ni, M. Kaufmann and W. Lin, J. Am. Chem. Soc., 2021, 143(5), 2194–2199 CrossRef CAS PubMed.
- T. Pham, V. Nguyen, Y. Choi, S. Lee and J. Yoon, Chem. Rev., 2021, 121(21), 13454–13619 CrossRef CAS.
- X. Li, S. Lee and J. Yoon, Chem. Soc. Rev., 2018, 47(4), 1174–1188 RSC.
- J. Sun, X. Cai, C. Wang, K. Du, W. Chen, F. Feng and S. Wang, J. Am. Chem. Soc., 2021, 143(2), 868–878 CrossRef CAS PubMed.
- Y. Xu, M. Zhao, L. Wu, F. Li, M. Li, M. Xie, S. Liu, W. Huang and Q. Zhao, ChemMedChem, 2019, 14(15), 1378–1383 CrossRef CAS.
- M. Li, T. Xiong, J. Du, R. Tian, M. Xiao, L. Guo, S. Long, J. Fan, W. Sun, K. Shao, X. Song, J. W. Foley and X. Peng, J. Am. Chem. Soc., 2019, 141(6), 2695–2702 CrossRef CAS PubMed.
- Y. Xu, M. Zhao, L. Zou, L. Wu, M. Xie, T. Yang, S. Liu, W. Huang and Q. Zhao, ACS Appl. Mater. Interfaces, 2018, 10(51), 44324–44335 CrossRef CAS PubMed.
- B. Xie, Y. Yu, X. Liu, J. Zeng, M. Zou, C. Li, X. Zeng and X. Zhang, Biomaterials, 2021, 272, 120782 CrossRef CAS PubMed.
- W. Zhou, Y. Chen, Y. Zhang, X. Xin, R. Li, C. Xie and Q. Fan, Small, 2020, 16(5), e1905641 CrossRef.
- W. Chen, Z. Sun, C. Jiang, W. Sun, B. Yu, W. Wang and L. Lu, Angew. Chem., Int. Ed., 2021, 60(30), 16641–16648 CrossRef CAS.
- X. Zheng, L. Wang, Y. Guan, Q. Pei, J. Jiang and Z. Xie, Biomaterials, 2020, 235, 119792 CrossRef CAS.
- Y. Liu, Y. Liu, W. Bu, C. Cheng, C. Zuo, Q. Xiao, Y. Sun, D. Ni, C. Zhang, J. Liu and J. Shi, Angew. Chem., Int. Ed., 2015, 54(28), 8105–8109 CrossRef CAS PubMed.
- Y. Liu, X. Meng and W. Bu, Coord. Chem. Rev., 2019, 379, 82–98 CrossRef CAS.
- M. Huo, P. Liu, L. Zhang, C. Wei, L. Wang, Y. Chen and J. Shi, Adv. Funct. Mater., 2021, 31(16), 2010196 CrossRef CAS.
- L. Fu, Y. Wan, C. Li, C. Qi, T. He, C. Yang, Y. Zhang, J. Lin and P. Huang, Adv. Funct. Mater., 2021, 31(14), 2009848 CrossRef CAS.
- J. Shen, H. Yu, Y. Shu, M. Ma and H. Chen, Adv. Funct. Mater., 2021, 31(50), 2106106 CrossRef CAS.
- Y. Sun, L. Xu, X. Liu, Y. Shen, Y. Zhang, N. Gu and F. Xiong, Chem. Eng. J., 2022, 429, 132303 CrossRef CAS.
- L. Zeng, H. Cheng, Y. Dai, Z. Su, C. Wang, L. Lei, D. Lin, X. Li, H. Chen, K. Fan and S. Shi, ACS Appl. Mater. Interfaces, 2021, 13(1), 233–244 CrossRef CAS.
- G. Yang, L. Xu, Y. Chao, J. Xu, X. Sun, Y. Wu, R. Peng and Z. Liu, Nat. Commun., 2017, 8(1), 902 CrossRef.
- M. R. Younis, G. He, J. Qu, J. Lin, P. Huang and X. H. Xia, Adv. Sci., 2021, 8(21), e2102587 CrossRef PubMed.
- M. Wang, J. Song, F. Zhou, A. R. Hoover, C. Murray, B. Zhou, L. Wang, J. Qu and W. R. Chen, Adv. Sci., 2019, 6(10), 1802157 CrossRef.
- T. Jia, J. Xu, S. Dong, F. He, C. Zhong, G. Yang, H. Bi, M. Xu, Y. Hu, D. Yang, P. Yang and J. Lin, Chem. Sci., 2019, 10(37), 8618–8633 RSC.
- C. Liu, B. Liu, J. Zhao, Z. Di, D. Chen, Z. Gu, L. Li and Y. Zhao, Angew. Chem., Int. Ed., 2020, 59(7), 2634–2638 CrossRef CAS.
- J. Fang, W. Islam and H. Maeda, Adv. Drug Delivery Rev., 2020, 157, 142–160 CrossRef CAS PubMed.
- Y. Ding, Y. Xu, W. Yang, P. Niu, X. Li, Y. Chen, Z. Li, Y. Liu, Y. An, Y. Liu, W. Shen and L. Shi, Nano Today, 2020, 35, 100970 CrossRef CAS.
- Y. Sun, L. Han and P. Strasser, Chem. Soc. Rev., 2020, 49(18), 6605–6631 RSC.
- C. Zhou, K. Cui, Y. Liu, L. Li, L. Zhang, S. Hao, S. Ge and J. Yu, ACS Appl. Mater. Interfaces, 2021, 13(28), 32780–32789 CrossRef CAS PubMed.
- D. F. Henning, P. Merkl, C. Yun, F. Iovino, L. Xie, E. Mouzourakis, C. Moularas, Y. Deligiannakis, B. Henriques-Normark, K. Leifer and G. A. Sotiriou, Biosens. Bioelectron., 2019, 132, 286–293 CrossRef CAS.
- S. Dong, Y. Dong, B. Liu, J. Liu, S. Liu, Z. Zhao, W. Li, B. Tian, R. Zhao, F. He, S. Gai, Y. Xie, P. Yang and Y. Zhao, Adv. Mater., 2022, 34, e2107054 CrossRef PubMed.
- R. Zhou, T. Y. Ohulchanskyy, H. Xu, R. Ziniuk and J. Qu, Small, 2021, 17(41), e2103569 CrossRef PubMed.
- V. Tvrdy, J. Pourova, E. Jirkovsky, V. Kren, K. Valentova and P. Mladenka, Med. Res. Rev., 2021, 41(4), 2195–2246 CrossRef CAS PubMed.
- L. Zhao, W. Yuan, J. Li, L. Yang, Y. Su, J. Peng, R. Chen, H. P. Tham, H. Chen, W. Q. Lim, H. Xiang, P. Xing, F. Li and Y. Zhao, Adv. Funct. Mater., 2018, 28(50), 1806162 CrossRef.
|
This journal is © The Royal Society of Chemistry 2023 |
Click here to see how this site uses Cookies. View our privacy policy here.