DOI:
10.1039/D2BM01691E
(Paper)
Biomater. Sci., 2023,
11, 108-118
A two-pronged strategy to alleviate tumor hypoxia and potentiate photodynamic therapy by mild hyperthermia†
Received
16th October 2022
, Accepted 25th November 2022
First published on 26th November 2022
Abstract
The application of photodynamic therapy (PDT) is limited by tumor hypoxia. To overcome hypoxia, catalase-like nanozymes are often used to catalyze endogenous H2O2 enriched in tumor tissues to O2. Nonetheless, the catalase activity may not be optimal at body temperature and the O2 supply may not meet the rapid O2 consumption of PDT. Herein, we provide a two-pronged strategy to alleviate tumor hypoxia based on hollow mesoporous Prussian blue nanoparticles (HMPB NPs). HMPB NPs can efficiently load the photosensitizer chlorin e6 (Ce6) and exhibit photothermal capability and temperature-dependent catalase activity. Under 808 nm laser irradiation, the photothermal effect of HMPB NPs elevated the catalase activity of HMPB NPs for O2 production. Furthermore, mild hyperthermia reduced cancer associated fibroblasts (CAFs) and induced extracellular matrix (ECM) degradation. The reduction of CAFs and the ECM decreased the solid stress of tumor tissues and normalized the tumor vasculature, which was beneficial for the external supplementation of O2 to tumors. Thereafter, under 606 nm laser irradiation, Ce6-mediated PDT generated excessive reactive oxygen species (ROS) that induced tumor cell apoptosis and achieved a high tumor inhibition rate of 92.2% in 4T1 breast tumors. Our work indicated that the alleviation of tumor hypoxia from both internal and external pathways significantly enhanced Ce6-mediated PDT against breast cancers.
Introduction
Photodynamic therapy (PDT) is a precise, non-invasive, and safe modality to treat cancers.1 To date, the efficacy of PDT against esophageal, lung, and prostate cancers has been tested in dozens of clinical trials.1–6 However, the application of PDT in other solid tumors such as breast cancer is limited by tumor hypoxia, due to the fast growth of cancer cells and insufficient blood supply.1,7–10 To overcome tumor hypoxia, numerous strategies have been developed including exogenous O2 delivery,11–14 hyperbaric oxygen treatment,15–19in situ O2 generation,20–23 reduction of cellular respiration24–26 and tumor vessel normalization.27–29 Thereinto, catalase-like nanozymes proficiently relieve hypoxia by catalyzing the endogenous H2O2 enriched in cancer cells to O2.30–33 Nonetheless, the catalase activity may not be optimal at body temperature and simply utilizing nanozymes to catalyze the endogenous H2O2 for O2 supply might not meet the rapid O2 consumption of PDT.34–39
Considering the temperature dependent catalase activity, photothermal therapy (PTT) is used to warm up nanozymes to speed up O2 generation.34–39 More importantly, mild PTT (≤45 °C) enables local depletion of CAFs and the ECM in the tumor that may provide another pathway to overcome tumor hypoxia by regulating the tumor mechanical microenvironment.8,10,40–45 During the progression of cancer, the activated CAFs and ECM generated by CAFs are key factors that lead to high solid stress in the tumor, which compresses tumor blood vessels, decreases blood perfusion, and reduces external oxygen supply.8,10,45 On the one hand, the activated CAFs generate large contractile forces that contract ECM components.46–48 On the other hand, CAFs secrete excessive extracellular matrix (ECM) proteins including collagen I and fibronectin, which increase tissue volume, and push and displace existing viscoelastic structures inside and outside the tumor tissues.46,48,49 These forces from CAFs and the ECM give rise to solid stresses towards tumor tissues and the surrounding vasculature. The depletion of CAFs and the ECM in the tumor may decrease the tumor solid stress, leading to normalization of the tumor vasculature and improvement of external O2 delivery.8,41,42,45,50,51 Therefore, mild photothermal hyperthermia in combination with a catalase-like nanozyme holds a great possibility to overcome tumor hypoxia by reinforcing both intracellular generation and extracellular provision of O2.
Hollow mesoporous Prussian blue nanoparticles (HMPB NPs) are a multifunctional nanoplatform that integrates excellent photothermal performance, catalase activity, and high drug loading capability.52–57 Owing to these merits, HMPB NPs have been used to boost chemotherapy,56,58 chemodynamic therapy,59 and immunotherapy.57 Here, we provide a two-pronged strategy to alleviate tumor hypoxia and potentiate photodynamic therapy based on HMPB NPs, Scheme 1. In detail, Ce6 was loaded in the cavities and mesopores of HMPB NPs (Ce6@HMPB NPs, Scheme 1A), which augmented the cellular uptake of Ce6 and increased the accumulation of Ce6 in tumor parenchyma. The photothermal effect of HMPB NPs provisionally elevated the catalase activity of HMPB NPs, which enhanced reactive oxygen species (ROS) generation by increasing O2 production. Furthermore, mild hyperthermia reduced intratumoral CAFs and induced ECM degradation. The reduction of CAFs and the ECM decreased the solid stress of the tumor and normalized the tumor vasculature, which was beneficial for the external supplementation of O2 to tumor tissues. In the end, we revealed that the PTT-boosted catalase activity of HMPB NPs and mild hyperthermia-improved O2 supply efficiently disrupted tumor hypoxia and synergized Ce6-mediated PDT against breast cancer (Scheme 1B).
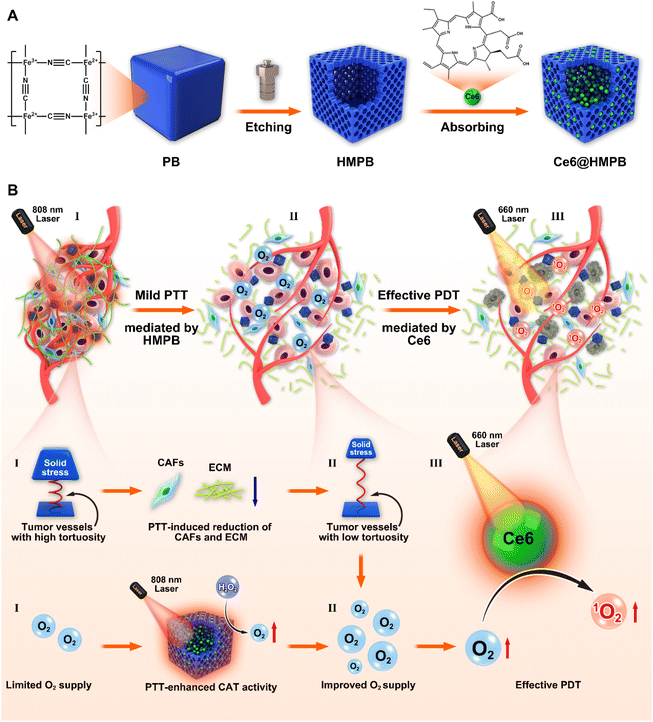 |
| Scheme 1 (A) Schematic illustration of the preparation of Ce6@HMPB NPs. (B) Schematic illustration of the PTT-enhanced catalase activity of HMPB NPs and mild PTT-induced normalization of the tumor vasculature for O2 supply and effective PDT. | |
Results and discussion
Synthesis and characterization of Ce6@HMPB NPs
The synthesis process of Ce6@HMPB NPs is shown in Scheme 1A. Hollow mesoporous Prussian blue nanoparticles (HMPB NPs) were prepared by chemical etching of Prussian blue nanoparticles under the protection of PVP.52 The TEM image of HMPB NPs confirmed the hollow structure of HMPB NPs (Fig. 1A). The N2 adsorption–desorption isotherms of PB NPs and HMPB NPs (Fig. 1B) and the corresponding pore-size distribution (Fig. S1A†) confirmed the existence of mesoporous pores on HMPB NPs, whose average pore width was 26.1 nm. Because of the pores created by chemical etching, the BET surface area of HMPB NPs (109.7 m2 g−1) was larger than that of PB NPs (77.9 m2 g−1). Then, chlorin e6 (Ce6) was absorbed into the mesopore and cavity of HMPB NPs to obtain Ce6@HMPB NPs. The appearance of characteristic absorption peaks (Fig. 1C) and fluorescence peaks of Ce6 (Fig. S1B†) as well as the decrease of the ζ-potential of HMPB NPs (Fig. 1D) indicated the successful and efficient loading of Ce6. When the feeding amount ratio of Ce6 and HMPB NPs was 1
:
10, Ce6@HMPB NPs had a uniform diameter of 237.2 nm and a high encapsulation efficiency of 97.45% (Fig. 1E and Fig. S1B, Table S1†). Ce6@HMPB NPs were stable in PBS and FBS solution for a week (Fig. 1F), suitable for intravenous injection. After chemical etching and Ce6 loading, Ce6@HMPB NPs still maintained high photothermal conversion efficiency (59.3%) and photothermal stability (stable for at least four cycles) (Fig. 1G–I). Besides, the photothermal effect of Ce6@HMPB NPs depended on the power of the 808 nm laser and the concentration of Ce6@HMPB NPs (Fig. S2A and B†). Compared with PB NPs, while the photothermal conversion efficiency decreased slightly for HMPB NPs, it recovered after the loading of Ce6 (Fig. S3†). HMPB NPs were reported to possess catalase activity that could catalyze the generation of O2 from H2O2.54 To test the catalase activity of Ce6@HMPB NPs, an O2 probe [Ru(dpp)3]Cl2 was used to detect O2 generation after the addition of Ce6@HMPB NPs into a 2% H2O2 solution. Since the fluorescence of [Ru(dpp)3]Cl2 could be quenched by O2, the relative fluorescence intensity of [Ru(dpp)3]Cl2 decreased with an increasing concentration of O2.60 The relative fluorescence intensity of [Ru(dpp)3]Cl2 decreased to 66.8%, 50.6% and 44.2% after the addition of PB NPs, HMPB NPs, and Ce6@HMPB NPs, respectively, implying the superior catalase activity of Ce6@HMPB NPs (Fig. 1J). Consistent with that, more bubbles appeared on the wall of the centrifugation tube in HMPB NP and Ce6@HMPB NP groups, implying plentiful O2 generation (Fig. S4A†). The superior catalase activity of the Ce6@HMPB NPs may be ascribed to the hollow mesoporous structure of HMPB NPs that increases the surface area for the reaction.61–63 The O2 generation was dependent on the concentration of Ce6@HMPB NPs and H2O2 (Fig. S4B and C†). Moreover, increasing the temperature from 25 °C to 45 °C decreased the relative fluorescence intensity of [Ru(dpp)3]Cl2 from 59.7% to 45.6%, which suggested that the catalase activity of Ce6@HMPB NPs could be further increased by heat (Fig. 1K). The catalase activity of Ce6@HMPB NPs could provide more O2 in the presence of H2O2 for PDT. Here, 1,3-diphenylisobenzofuran (DPBF) was used as an 1O2 indicator.60 Under 660 nm laser irradiation, the absorption of DPBF at 426 nm decreased by 43.1% in the Ce6@HMPB + 660 nm group, 47.6% in the Ce6@HMPB + H2O2 + 660 nm group and 53.3% in the Ce6@HMPB + H2O2 + 808 nm + 660 nm group, which suggested that more 1O2 was generated when O2 was efficiently produced through PTT boosted-catalase activity (Fig. 1L and Fig. S5†). The above results suggested that Ce6@HMPB NPs could act as a self-amplifier that increased its catalase activity by the photothermal effect. As more O2 was generated from H2O2, stronger PDT could be achieved.
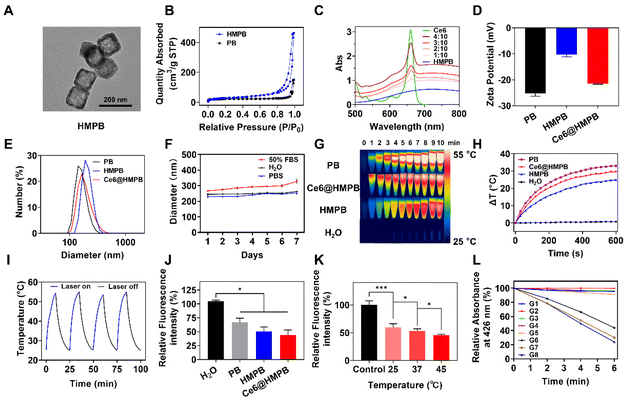 |
| Fig. 1 Characterization of PB NPs, HMPB NPs, and Ce6@HMPB NPs. (A) TEM image of Ce6@HMPB NPs. The scale bar is 200 nm. (B) N2 adsorption–desorption isotherms of PB and HMPB NPs. (C) UV-vis absorbance of Ce6, HMPB NPs and Ce6@HMPB NPs in DMSO with different feeding amount ratios of Ce6 and HMPB NPs from 1 : 10 to 4 : 10. (D) ζ-Potential of PB, HMPB and Ce6@HMPB NPs in water. (E) Hydrodynamic diameter distribution of PB, HMPB and Ce6@HMPB NPs. (F) Diameter change of Ce6@HMPB NPs in 50% FBS, ultrapure water and PBS. (G) Infrared thermal images of different formulations at varying time points and the corresponding temperature changes (H) under 808 nm laser irradiation (1 W cm−2, 50 μg mL−1). (I) Temperature changes of Ce6@HMPB NPs during four on/off cycles of laser irradiation (1 W cm−2, 50 μg mL−1). (J) Relative fluorescence intensity of the O2 probe [Ru(dpp)3]Cl2 in different groups (2% H2O2, 20 μg mL−1 nanoparticles, 25 °C, n = 3). (K) Relative fluorescence intensity of the O2 probe [Ru(dpp)3]Cl2 in Ce6@HMPB NP dispersion at different temperatures (1 × 10−4 M H2O2, 3 μg mL−1 Ce6@HMPB NPs, n = 5). (L) The relative absorbance of DPBF solution at 425 nm in different groups at different time points. G1: DPBF, G2: DPBF + Ce6@HMPB NPs, G3: DPBF + Ce6@HMPB NPs + H2O2, G4: DPBF + Ce6@HMPB NPs + H2O2 + 808 nm, G5: DPBF + 660 nm, G6: DPBF + Ce6@HMPB NPs + 660 nm, G7: DPBF + Ce6@HMPB NPs + H2O2 + 660 nm, G8: DPBF + Ce6@HMPB NPs + H2O2 + 808 nm + 660 nm. Error bars indicate SD. *, P < 0.05; ***, P < 0.001 using the one-way ANOVA test. | |
Cellular uptake, intracellular ROS generation, and photo-cytotoxicity of Ce6@HMPB NPs in vitro
Considering the limited lifetime and diffusion distance of ROS,64 it is important for photosensitizers to enter cells and produce intracellular ROS that destroy organelles and exert cytotoxicity. In accordance with previous work,65 loading Ce6 into HMPB NPs increased the cellular uptake of Ce6 by 4T1 cancer cells. Semi-quantification of CLSM showed the relative fluorescence intensity of intracellular Ce6 in the Ce6@HMPB NP group was 3.3 and 5.1 times that in the Ce6 group at 1 μg mL−1 and 2 μg mL−1 Ce6 (Fig. 2A and B). Flow cytometry also showed 4T1 cancer cells internalized 3.8 and 4.4 times that of Ce6 in the Ce6@HMPB NP group than that in the free Ce6 group (Fig. S6A and B†). Because of the lifted cellular uptake of Ce6 and PTT self-amplified catalase activity of HMPB NPs to convert H2O2 to O2, robust PDT was achieved in vitro in 4T1 cancer cells. The intracellular production of ROS was monitored by DCFH-DA, which could be hydrolyzed to DCFH by esterase in cells and be oxidized by ROS to form DCF with strong fluorescence.66 Flow cytometry analysis exhibited that the intracellular fluorescence intensity of DCF in the Ce6@HMPB NPs + 660 nm laser group was 1.14 times that in the Ce6 + 660 nm laser group (Fig. 2C), which is due to the increased uptake of Ce6 (Fig. 2A, B and Fig. S6A, B†) and catalytic generation of O2 (Fig. 1J and K). The Ce6@HMPB NPs + 808 nm + 660 nm laser group showed the highest fluorescence intensity of DCF, 1.55 times that in the Ce6 + 660 nm laser group and 1.36 times that in the Ce6@HMPB NPs + 660 nm laser group (Fig. 2C). This was because pretreatment with an 808 nm laser before 660 nm laser irradiation temporally boosted the catalase activity of Ce6@HMPB NPs and increased the O2 supply (Fig. 1K). An MTT assay was used to evaluate the dark cytotoxicity and photo-cytotoxicity of Ce6@HMPB NPs. Without laser irradiation, Ce6@HMPB NPs exerted little toxicity towards 4T1 and HUVEC cells, indicating the safety and biocompatibility of Ce6@HMPB NPs (Fig. 2D and Fig. S7†). Mild PTT under an 808 nm laser (Ce6@HMPB NPs + 808 nm) showed limited cytotoxicity to 4T1 cancer cells because of the relatively low temperature (<45 °C) under an 808 nm laser (Fig. 2E). Under a 660 nm laser, Ce6 mediated PDT caused a dramatic decrease of cell viability because of ROS damage. The Ce6@HMPB NPs + 660 nm laser group significantly lowered the IC50 (half maximal inhibitory concentration) of Ce6 to 0.98, compared to 1.86 in the Ce6 + 660 nm group, while mild PTT further reduced the IC50 to 0.34 because the intracellular ROS greatly increased (Fig. 2C). The calcein acetoxymethyl ester (calcein-AM)/propidium iodide (PI) staining assay was carried out to visualize living and dead cells via green and red fluorescence, respectively. In accordance with the result of MTT, Ce6 with 660 nm laser irradiation only killed part of the cells and Ce6@HMPB NPs with 660 nm laser irradiation killed most of the cells in the field, while Ce6@HMPB NPs with 808 nm and 660 nm laser irradiation almost killed all cancer cells (Fig. 2F and G). Next, the lethal mechanism of Ce6@HMPB NP mediated phototherapy was analyzed by flow cytometry using Annexin V-APC and PI double staining in 4T1 cancer cells (Fig. 2H and I). Ce6@HMPB NPs with 808 nm and 660 nm laser treatment resulted in a higher apoptosis rate (9.0% early apoptosis and 34.1% late apoptosis) compared to the control (1.1% early apoptosis and 5.2% late apoptosis), Ce6@HMPB NPs (1.0% early apoptosis and 5.2% late apoptosis) and Ce6@HMPB NPs with 660 nm laser treatment (9.3% early apoptosis and 31.7% late apoptosis), suggesting that the photo-cytotoxicity of Ce6@HMPB NPs mainly came from the apoptosis-induced cell death pathway. The above results illustrated that Ce6@HMPB NPs mediated effective phototherapy in vitro by elevating the ROS levels in cancer cells by both increasing the intracellular photosensitizer content (Fig. 2A, B and Fig. S6A, B†) and reinforcing the O2 supply (Fig. 1J and K).
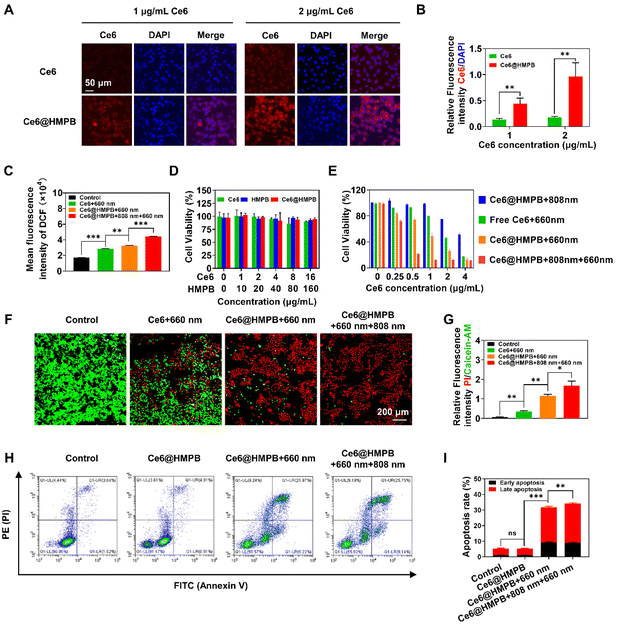 |
| Fig. 2 Cellular uptake, intracellular ROS generation and photo-cytotoxicity of Ce6@HMPB NPs. (A) Cellular uptake of Ce6 in 4T1 cancer cells as observed by CLSM after incubation with Ce6 or Ce6@HMPB NPs for 6 h. The scale bar is 50 μm. (B) Relative fluorescence intensity of Ce6 to DAPI semi-quantified from (A). (C) Intracellular DCF fluorescence intensity detected by flow cytometry. (D) Cell viability of 4T1 cancer cells 24 h after various treatments. Ce6@HMPB NPs contained 0, 1, 2, 4, 8 and 16 μg mL−1 Ce6, respectively. (E) Cell viability of 4T1 cancer cells 24 h after various treatments: 808 nm laser, 1 W cm−2; 660 nm laser, 200 mW cm−2. (F) CLSM images of living and dead cells detected by calcein-AM and PI double staining. The scale bar is 200 μm. (G) Relative fluorescence intensity of PI to calcein-AM semi-quantified from (F). (H) Cell apoptosis assay with Annexin V-FITC and PI double staining in 4T1 cancer cells by flow cytometry, and the corresponding quantitative analysis (I). Error bars indicate SD (n = 3). *, P < 0.05; **, P < 0.01; ***, P < 0.001 by Student's t-test, ns stands for not significant. | |
Ce6@HMPB NPs efficiently accumulated in tumors
To guarantee the in vivo feasibility of Ce6@HMPB NPs, the in vivo pharmacokinetics of Ce6@HMPB NPs was first investigated. The circulating half-life of Ce6@HMPB NPs in the blood stream was calculated to be 3.65 h by detecting the content of ferrum using ICP-OES (Fig. S8†). Then, the biodistribution of Ce6 and Ce6@HMPB NPs was investigated by detecting the fluorescence of Ce6 with an animal fluorescence imaging system at various time points. As shown in Fig. 3A and B, Ce6@HMPB NPs rapidly accumulated in tumor parenchyma and remained at a high level in 24 h. The ex vivo images of main organs and tumors also showed a higher retention of Ce6 in tumors in the Ce6@HMPB NP group than that in the free Ce6 group 12 h and 24 h after intravenous injection, 1.4 and 2.0 times, respectively (Fig. 3C–E). This may be the result of the enhanced permeability and retention effect (EPR effect) and the relatively long circulation of Ce6@HMPB NPs than free Ce6 in blood.67–70 The above results demonstrated that Ce6@HMPB NPs efficiently accumulated and lingered in tumor tissues, which would benefit the following in vivo phototherapy.
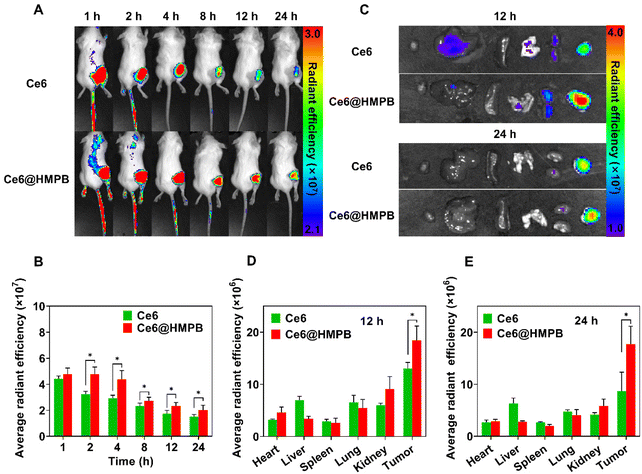 |
| Fig. 3 Ce6@HMPB NPs effectually accumulated in tumors. (A) Real time fluorescence images of mice after the injection of Ce6 or Ce6@HMPB NP dispersions and the corresponding average radiant efficiency in tumor sites (B) (n = 5). (C) Ex vivo fluorescence images of major organs and tumors from Ce6 or Ce6@HMPB NPs treated mice and the corresponding average radiant efficiency at 12 h (D) and 24 h (E), respectively, (n = 3). Error bars indicate SD. *, P < 0.05 by Student's t-test. | |
Regulation of both tumor solid stress and catalase activity by mild PTT alleviated tumor hypoxia in vivo
In vitro, mild PTT had been shown to boost the catalase activity of HMPB NPs which could offer more O2 for PDT. In vivo, mild PTT may not only provide internal O2 supply by boosting the catalase activity of HMPB NPs, but also may provide external O2 delivery by regulating the tumor solid stress and tumor vasculature. It has been reported that mild hyperthermia can induce the reduction of CAFs and the ECM.40–44 These changes in tumor stroma hold great possibility in regulating the tumor solid stress, improving the tumor vasculature, and alleviating tumor hypoxia by an external O2 supply.8,45,71,72 In view of the photothermal ability of Ce6@HMPB NPs in vitro and in vivo (Fig. 1G–I and Fig. S2, S3, S9†), the influence of mild PTT on the ECM, CAFs, tumor solid stress, tumor vasculature and tumor hypoxia was investigated.
Ce6@HMPB NPs exhibited a power-dependent photothermal effect in vivo (Fig. S9†). In the following experiments, the power of the 808 nm laser was adjusted to lower than 1.5 W cm−2 to keep the temperature of the tumor in the 43 °C to 45 °C range. In this case, mild hyperthermia is enough to regulate the tumor microenvironment while causing little harm to normal tissues.40,42
As expected, Ce6@HMPB NPs mediated mild hyperthermia reduced CAFs and the ECM in tumors. Immunofluorescence and Masson staining of tumor slices illustrated that the mean fluorescence intensity of α-SMA (a marker of activated myofibroblasts), collagen I, fibronectin and the area of collagen were significantly decreased by Ce6@HMPB NPs mediated mild hyperthermia, but Ce6@HMPB NPs had no influence on these components (Fig. 4A–E). Along with the changes in tumor stroma, the solid stresses after different treatments were further studied by the tumor opening experiment.45 The results showed that tumor solid stress declined 49.4% after mild PTT compared to the saline treated group (Fig. 5A and B). As a development, the vasculature in tumors was improved after the relief of solid stress.8,45 The area of the tumor vessel and the vessel tortuosity decreases by 19.0% and 57.8%, respectively, after mild PTT (Fig. 5C–E), indicating the normalization of the tumor vasculature.9,18,73,74 The effect of mild PTT and the catalase activity of Ce6@HMPB NPs on reliving tumor hypoxia was investigated by immunofluorescence staining of HIF-1α. While Ce6@HMPB NPs only reduced the 25.6% HIF-1α positive region compared to saline, the Ce6@HMPB NPs plus 808 nm laser decreased the 60.4% HIF-1α positive region. This demonstrated that simply increasing internal O2 generation by the catalase activity of Ce6@HMPB NPs in body temperature was not enough. Mild PTT not only boosted the catalase activity of Ce6@HMPB NPs for internal O2 generation, but also normalized the tumor vasculature for external O2 supply, which was in favor of PDT against tumors.
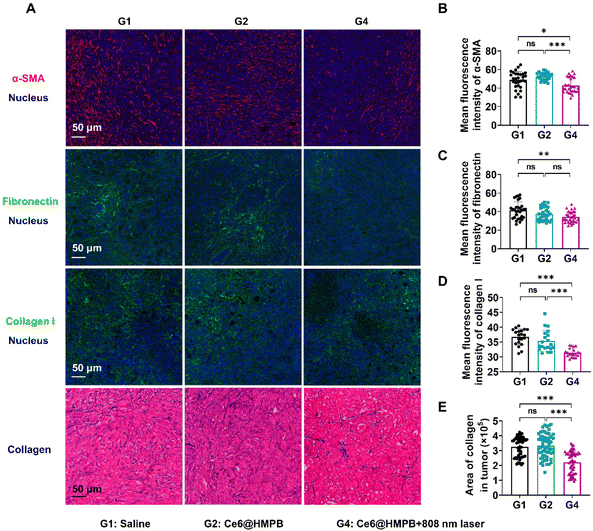 |
| Fig. 4 Mild PTT of Ce6@HMPB NPs reduced CAFs and ECM in tumor tissues. (A) Immunofluorescence staining images of α-SMA, collagen I and fibronectin, and Masson staining images of tumor slices. The scale bar is 50 μm. Quantification of the mean fluorescence intensity of α-SMA (B) (n = 30), collagen I (C) (n = 20), fibronectin (D) (n = 30) and area of collagen (E) (n = 60). Error bars indicate SD. **, P < 0.01; ***, P < 0.001 using the one-way ANOVA test, ns stands for not significant. | |
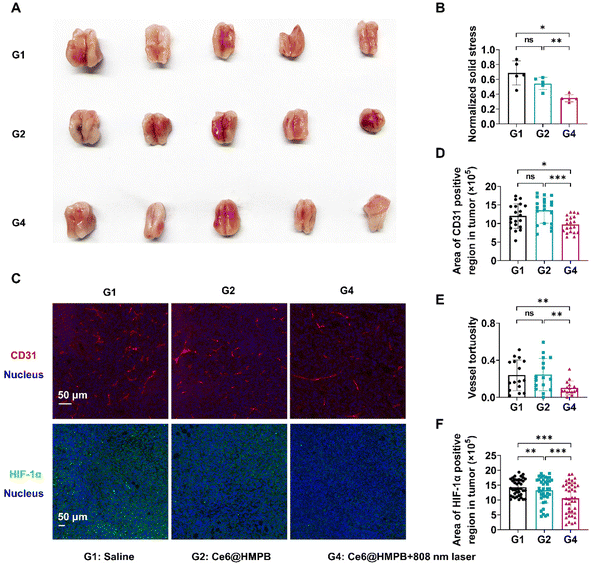 |
| Fig. 5 Mild PTT of Ce6@HMPB NPs decreased the solid stress in tumors and normalized the tumor vasculature. (A) Pictures of the tumor opening of groups 12 h after treatment with saline, Ce6@HMPB NPs, and Ce6@HMPB NPs plus 808 nm laser irradiation. (B) Normalized solid stress calculated from samples in (A) (n = 5). (C) Immunofluorescence staining images of CD31 and HIF-1α in tumor slices. Scale bars are 50 μm. (D) Area of CD31 positive regions (n = 20). (E) Quantification of tumor blood vessel tortuosity (n = 16). (F) Area of HIF-1α positive regions (n = 40). Error bars indicate SD. *, P < 0.05; **, P < 0.01; ***, P < 0.001 using the one-way ANOVA test, ns stands for not significant. | |
Ce6@HMPB NP-mediated mild PTT potentiated PDT against 4T1 breast cancer in vivo
Considering the capability of the Ce6@HMPB NPs plus 808 nm laser to alleviate tumor hypoxia in vivo, Ce6@HMPB NPs were expected to cause strong PDT against tumors. Hence, we tested the efficacy of this phototherapy in 4T1 tumor-bearing mice. The mice in all groups received drug administration only once on day 0. Mild PTT was done 12 h after intravenous injection of Ce6@HMPB NPs. PDT was done 14 h after intravenous injection of Ce6@HMPB NPs or Ce6. For the group receiving both PTT and PDT, PDT was done 2 h after PTT (Fig. 6A). Ce6@HMPB NPs (G2) only worked as catalase to relieve tumor hypoxia (Fig. 5F) but possessed no toxicity to the tumor (Fig. 6B–E). Ce6 plus 660 nm laser irradiation (G3) had a moderate inhibitory effect on the tumor (Fig. 6B–E and G–I) because of the limited accumulation of Ce6 in the tumor (Fig. 3) and the hypoxic tumor microenvironment (Fig. 5F). Ce6@HMPB NPs with 808 nm laser irradiation resulted in partial inhibition of tumors with 64.0% tumor inhibition rate (TIR) because mild PTT was not strong enough to eliminate malignant tumors. Ce6@HMPB NPs with 660 nm laser irradiation (G5) also inhibited part of the tumor with a TIR of 66.3% because of the limited alleviation of tumor hypoxia by Ce6@HMPB NPs (Fig. 5F). Mild PTT in combination with PDT achieved strong tumor inhibition with 92.2% inhibition rate, as a result of efficient O2 supply (Fig. 5F) by mild PTT-boosted catalase activity (Fig. 1K) and mild PTT-normalized tumor vasculature (Fig. 5C–E). Subsequently, hematoxylin eosin (H&E), caspase 3, and Ki67 staining of the tumor sections was performed to further evaluate the treatment effect of each group. In agreement with the tumor suppression assay, mild PTT in combination with PDT led to a higher apoptosis rate and lower proliferation rate compared to other treatments (Fig. 6G–I). Although the treatments caused damage to the tumor tissues, negligible side effects on mice were evidenced by body weight (Fig. 6F), H&E staining of major organs (Fig. S10†), blood routine and blood biochemistry analyses (Fig. S11†), indicating the safety of Ce6@HMPB NP-mediated phototherapy.
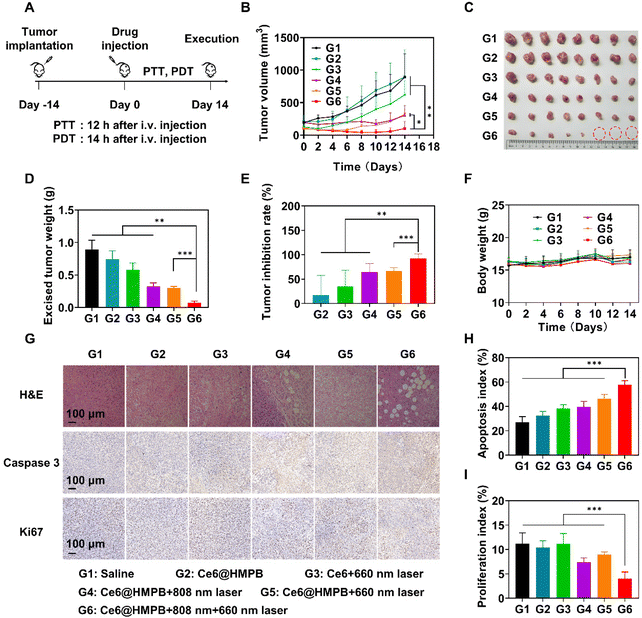 |
| Fig. 6 Mild PTT of Ce6@HMPB NPs potentiated PDT against 4T1 breast cancers. (A) Schematic illustration of treatment. (B) Tumor volume evolvement curves (n = 8). (C) Digital photograph and final tumor weight (D) of the excised tumors by the end of treatment (n = 8). (E) Tumor inhibition rate calculated by the tumor weight (n = 8). (F) Changes in body weight after different treatments (n = 8). (G) H&E, caspase 3, and Ki67 staining of the tumor regions after different treatments. Scale bars are 100 μm. Semi-quantification of the apoptosis index (H) of the tumor tissue in caspase 3 staining and proliferation index (I) of the tumor tissue in Ki67 staining (n = 10). Error bars indicate SD. *, P < 0.05; **, P < 0.01; ***, P < 0.001 using the one-way ANOVA test. | |
Conclusions
In this work, we leveraged HMPB NPs to deliver the photosensitizer Ce6 and boost Ce6 mediated PDT against 4T1 breast tumors by efficiently alleviating tumor hypoxia. Loading Ce6 into HMPB NPs augmented the cellular uptake of Ce6 and increased the accumulation of Ce6 in tumor parenchyma, which provided enough photosensitizer for PDT. More importantly, considering the limited O2 supply in the hostile tumor microenvironment, the photothermal ability and temperature-dependent catalase activity of HMPB NPs were applied to disrupt tumor hypoxia. The mild photothermal effect of HMPB NPs provisionally increased the catalase activity of HMPB NPs for enhanced O2 production. Furthermore, mild hyperthermia reduced CAFs and induced ECM degradation in tumor tissues. The reduction of CAFs and the ECM decreased 49.4% solid stress in tumors and normalized the tumor vasculature, which was beneficial for external supplementation of O2 to tumors. In the end, we revealed that the PTT-boosted catalase activity of HMPB NPs and the PTT-normalized vasculature efficiently disrupted tumor hypoxia and boosted Ce6-mediated PDT against breast cancers. With drug administration once followed by mild PTT and PDT once, a tumor inhibition rate of 92.2% was realized in 4T1 breast cancer-bearing mice. As tumor hypoxia is a leading obstacle for PDT, this work offers a two-pronged strategy to overcome tumor hypoxia by reinforcing both intracellular generation and extracellular provision of O2.
Data availability
The raw/processed data required to reproduce these findings are available from the corresponding author.
Ethical statement
All animal handling procedures were performed in accordance with the internationally accepted principles and Guidelines for the Care and Use of Laboratory Animals of Huazhong University of Science and Technology. Experimental protocols were approved by the Institutional Animal Ethical Committee of the Huazhong University of Science and Technology.
Author contributions
Z. Li and X. Yang supervised and supported this project. Z. Li, Z. Wang, Y. Xiong, and Z. Zhang conceived this project. Z. Wang, Z. Zhang, Y. Xiong, C. Wang, Q. Deng, T. Yang, Q. Xu, and Z. Yong performed the experiments. Z. Zhang, Z. Wang and Z. Li analyzed the data. Z. Zhang wrote the manuscript. Z. Zhang, Y. Xiong, and Z. Li contributed to the revision. All authors discussed and commented on the manuscript.
Conflicts of interest
The authors claim no conflicts of interest.
Acknowledgements
We thank the Research Core Facilities for Life Science (HUST), the Optical Bioimaging Core Facility of WNLO-HUST, and the Analytical and Testing Center of HUST for the facility support. This work was financially supported by grants from the National Research and Development Program of China (2018YFA0208900, 2020YFA0211200, and 2020YFA0710700), the National Science Foundation of China (82172757 and 31972927), the Scientific Research Foundation of Huazhong University of Science and Technology (3004170130), the Program for HUST Academic Frontier Youth Team (2018QYTD01), and the HCP Program for HUST.
References
- X. Li, J. F. Lovell, J. Yoon and X. Chen, Nat. Rev. Clin. Oncol., 2020, 17, 657–674 CrossRef PubMed.
- A. Sibille, R. Lambert, J. C. Souquet, G. Sabben and F. Descos, Gastroenterology, 1995, 108, 337–344 CrossRef PubMed.
- Y. Hayata, H. Kato, C. Konaka, J. Ono and N. Takizawa, Chest, 1982, 81, 269–277 CrossRef PubMed.
- H. Patel, R. Mick, J. Finlay, T. C. Zhu, E. Rickter, K. A. Cengel, S. B. Malkowicz, S. M. Hahn and T. M. Busch, Clin. Cancer Res., 2008, 14, 4869–4876 CrossRef PubMed.
- J. C. Finlay, T. C. Zhu, A. Dimofte, D. Stripp, S. B. Malkowicz, R. Whittington, J. Miles, E. Glatstein and S. M. Hahn, Proc. SPIE, 2014, 5315, 132–142 CrossRef PubMed.
- W. Yan, Y. Zhou, Z. Zhou, Z. Ji and H. Li, Lancet Oncol., 2017, 18, e187 CrossRef.
- Y. Wan, L. H. Fu, C. Li, J. Lin and P. Huang, Adv. Mater., 2021, 33, e2103978 CrossRef.
- T. Stylianopoulos, L. L. Munn and R. K. Jain, Trends Cancer, 2018, 4, 292–319 CrossRef CAS PubMed.
- R. K. Jain, Nat. Med., 2001, 7, 987–989 CrossRef CAS PubMed.
- H. T. Nia, L. L. Munn and R. K. Jain, Science, 2020, 370, eaaz0868 CrossRef CAS.
- W. L. Liu, T. Liu, M. Z. Zou, W. Y. Yu, C. X. Li, Z. Y. He, M. K. Zhang, M. D. Liu, Z. H. Li, J. Feng and X. Z. Zhang, Adv. Mater., 2018, 30, e1802006 CrossRef PubMed.
- Y. Cheng, H. Cheng, C. Jiang, X. Qiu, K. Wang, W. Huan, A. Yuan, J. Wu and Y. Hu, Nat. Commun., 2015, 6, 8785 CrossRef CAS PubMed.
- X. Liang, M. Chen, P. Bhattarai, S. Hameed and Z. Dai, ACS Nano, 2020, 14, 13569–13583 CrossRef.
- S. Gao, P. Zheng, Z. Li, X. Feng, W. Yan, S. Chen, W. Guo, D. Liu, X. Yang, S. Wang, X. J. Liang and J. Zhang, Biomaterials, 2018, 178, 83–94 CrossRef.
- X. Wu, Y. Zhu, W. Huang, J. Li, B. Zhang, Z. Li and X. Yang, Adv. Sci., 2018, 5, 1700859 CrossRef PubMed.
- J. Li, J. Huang, Y. Ao, S. Li, Y. Miao, Z. Yu, L. Zhu, X. Lan, Y. Zhu, Y. Zhang and X. Yang, ACS Appl. Mater. Interfaces, 2018, 10, 22985–22996 CrossRef PubMed.
- X. Liu, N. Ye, S. Liu, J. Guan, Q. Deng, Z. Zhang, C. Xiao, Z. Y. Ding, B. X. Zhang, X. P. Chen, Z. Li and X. Yang, Adv. Sci., 2021, 8, e2100233 CrossRef PubMed.
- X. Liu, N. Ye, C. Xiao, X. Wang, S. Li, Y. Deng, X. Yang, Z. Li and X. Yang, Nano Today, 2021, 40, 101248 CrossRef.
- X. Wang, N. Ye, C. Xu, C. Xiao, Z. Zhang, Q. Deng, S. Li, J. Li, Z. Li and X. Yang, Nano Today, 2022, 44, 101458 CrossRef.
- M. Huo, L. Wang, L. Zhang, C. Wei, Y. Chen and J. Shi, Angew. Chem., Int. Ed., 2020, 59, 1906–1913 CrossRef PubMed.
- H. Chen, J. Tian, W. He and Z. Guo, J. Am. Chem. Soc., 2015, 137, 1539–1547 CrossRef PubMed.
- J. Kim, H. R. Cho, H. Jeon, D. Kim, C. Song, N. Lee, S. H. Choi and T. Hyeon, J. Am. Chem. Soc., 2017, 139, 10992–10995 CrossRef PubMed.
- X. L. Liu, X. Dong, S. C. Yang, X. Lai, H. J. Liu, Y. Gao, H. Y. Feng, M. H. Zhu, Y. Yuan, Q. Lu, J. F. Lovell, H. Z. Chen and C. Fang, Adv. Sci., 2021, 8, 2003679 CrossRef PubMed.
- Y. Deng, P. Song, X. Chen, Y. Huang, L. Hong, Q. Jin and J. Ji, ACS Nano, 2020, 14, 9711–9727 CrossRef PubMed.
- X. Mai, Y. Zhang, H. Fan, W. Song, Y. Chang, B. Chen, J. Shi, X. Xin, Z. Teng, J. Sun and G. Teng, Biomaterials, 2020, 232, 119699 CrossRef PubMed.
- L. P. Zhao, R. R. Zheng, H. Q. Chen, L. S. Liu, X. Y. Zhao, H. H. Liu, X. Z. Qiu, X. Y. Yu, H. Cheng and S. Y. Li, Nano Lett., 2020, 20, 2062–2071 CrossRef PubMed.
- H. Gong, Y. Chao, J. Xiang, X. Han, G. Song, L. Feng, J. Liu, G. Yang, Q. Chen and Z. Liu, Nano Lett., 2016, 16, 2512–2521 CrossRef PubMed.
- Y. Deng, F. Jia, S. Chen, Z. Shen, Q. Jin, G. Fu and J. Ji, Biomaterials, 2018, 187, 55–65 CrossRef PubMed.
- Y. C. Sung, P. R. Jin, L. A. Chu, F. F. Hsu, M. R. Wang, C. C. Chang, S. J. Chiou, J. T. Qiu, D. Y. Gao, C. C. Lin, Y. S. Chen, Y. C. Hsu, J. Wang, F. N. Wang, P. L. Yu, A. S. Chiang, A. Y. Wu, J. J. Ko, C. P. Lai, T. T. Lu and Y. Chen, Nat. Nanotechnol., 2019, 14, 1160–1169 CrossRef CAS PubMed.
- S. A. Castaldo, T. Ajime, G. Serrão, F. Anastácio, J. T. Rosa, C. A. Giacomantonio, A. Howarth, R. Hill and P. A. Madureira, Cancers, 2019, 11, 492 CrossRef CAS PubMed.
- Z. L. Yang, W. Tian, Q. Wang, Y. Zhao, Y. L. Zhang, Y. Tian, Y. X. Tang, S. J. Wang, Y. Liu, Q. Q. Ni, G. M. Lu, Z. G. Teng and L. J. Zhang, Adv. Sci., 2018, 5, 1700847 CrossRef.
- G. Yang, L. Xu, Y. Chao, J. Xu, X. Sun, Y. Wu, R. Peng and Z. Liu, Nat. Commun., 2017, 8, 902 CrossRef.
- J. J. Hu, Y. Chen, Z. H. Li, S. Y. Peng, Y. Sun and X. Z. Zhang, Nano Lett., 2019, 19, 5568–5576 CrossRef CAS.
- L. Song, B. Chen, Z. Qin, X. Liu, Z. Guo, H. Lou, H. Liu, W. Sun, C. Guo and C. Li, Adv. Healthcare Mater., 2022, 11, e2102298 CrossRef.
- P. Xu, X. Wang, T. Li, H. Wu, L. Li, Z. Chen, L. Zhang, Z. Guo and Q. Chen, Nanoscale, 2020, 12, 4051–4060 RSC.
- P. Dong, W. Wang, M. Pan, W. Yu, Y. Liu, T. Shi, J. Hu, Y. Zhou, S. Yu, F. Wang and X. Liu, ACS Appl. Mater. Interfaces, 2021, 13, 16075–16083 CrossRef CAS PubMed.
- Y. Yang, D. Zhu, Y. Liu, B. Jiang, W. Jiang, X. Yan and K. Fan, Nanoscale, 2020, 12, 13548–13557 RSC.
- B. Tian, C. Wang, Y. Du, S. Dong, L. Feng, B. Liu, S. Liu, H. Ding, S. Gai, F. He and P. Yang, Small, 2022, 18, e2200786 CrossRef PubMed.
- H. Yang, B. Xu, S. Li, Q. Wu, M. Lu, A. Han and H. Liu, Small, 2021, 17, e2007090 CrossRef.
- A. Nicolás-Boluda, J. Vaquero, G. Laurent, G. Renault, R. Bazzi, E. Donnadieu, S. Roux, L. Fouassier and F. Gazeau, ACS Nano, 2020, 14, 5738–5753 CrossRef.
- Q. Chen, Q. Hu, E. Dukhovlinova, G. Chen, S. Ahn, C. Wang, E. A. Ogunnaike, F. S. Ligler, G. Dotti and Z. Gu, Adv. Mater., 2019, 31, e1900192 CrossRef.
- Z. Chen, H. Pan, Y. Luo, T. Yin, B. Zhang, J. Liao, M. Wang, X. Tang, G. Huang, G. Deng, M. Zheng and L. Cai, Small, 2021, 17, e2007494 CrossRef PubMed.
- T. Tan, H. Hu, H. Wang, J. Li, Z. Wang, J. Wang, S. Wang, Z. Zhang and Y. Li, Nat. Commun., 2019, 10, 3322 CrossRef.
- T. Teng, R. Lin, Z. Lin, K. Ke, X. Lin, M. Pan, D. Zhang and H. Huang, Biomater. Sci., 2020, 8, 3278–3285 RSC.
- T. Stylianopoulos, J. D. Martin, V. P. Chauhan, S. R. Jain, B. Diop-Frimpong, N. Bardeesy, B. L. Smith, C. R. Ferrone, F. J. Hornicek, Y. Boucher, L. L. Munn and R. K. Jain, Proc. Natl. Acad. Sci. U. S. A., 2012, 109, 15101–15108 CrossRef.
- H. T. Nia, H. Liu, G. Seano, M. Datta, D. Jones, N. Rahbari, J. Incio, V. P. Chauhan, K. Jung, J. D. Martin, V. Askoxylakis, T. P. Padera, D. Fukumura, Y. Boucher, F. J. Hornicek, A. J. Grodzinsky, J. W. Baish, L. L. Munn and R. K. Jain, Nat. Biomed. Eng., 2016, 1, 0004 CrossRef PubMed.
- D. D. Simon, C. O. Horgan and J. D. Humphrey, J. Mech. Behav. Biomed. Mater., 2012, 14, 216–226 CrossRef PubMed.
- E. Sahai, I. Astsaturov, E. Cukierman, D. G. DeNardo, M. Egeblad, R. M. Evans, D. Fearon, F. R. Greten, S. R. Hingorani, T. Hunter, R. O. Hynes, R. K. Jain, T. Janowitz, C. Jorgensen, A. C. Kimmelman, M. G. Kolonin, R. G. Maki, R. S. Powers, E. Puré, D. C. Ramirez, R. Scherz-Shouval, M. H. Sherman, S. Stewart, T. D. Tlsty, D. A. Tuveson, F. M. Watt, V. Weaver, A. T. Weeraratna and Z. Werb, Nat. Rev. Cancer, 2020, 20, 174–186 CrossRef PubMed.
- G. Seano, H. T. Nia, K. E. Emblem, M. Datta, J. Ren, S. Krishnan, J. Kloepper, M. C. Pinho, W. W. Ho, M. Ghosh, V. Askoxylakis, G. B. Ferraro, L. Riedemann, E. R. Gerstner, T. T. Batchelor, P. Y. Wen, N. U. Lin, A. J. Grodzinsky, D. Fukumura, P. Huang, J. W. Baish, T. P. Padera, L. L. Munn and R. K. Jain, Nat. Biomed. Eng., 2019, 3, 230–245 CrossRef PubMed.
- Q. Li, L. Hang, W. Jiang, J. Dou, L. Xiao, X. Tang, Y. Yao and Y. Wang, Biomaterials, 2020, 257, 120235 CrossRef PubMed.
- W. Jiang, Z. Zhang, Q. Wang, J. Dou, Y. Zhao, Y. Ma, H. Liu, H. Xu and Y. Wang, Nano Lett., 2019, 19, 4060–4067 CrossRef PubMed.
- M. Hu, S. Furukawa, R. Ohtani, H. Sukegawa, Y. Nemoto, J. Reboul, S. Kitagawa and Y. Yamauchi, Angew. Chem., Int. Ed., 2012, 51, 984–988 CrossRef PubMed.
- X. Jia, X. Cai, Y. Chen, S. Wang, H. Xu, K. Zhang, M. Ma, H. Wu, J. Shi and H. Chen, ACS Appl. Mater. Interfaces, 2015, 7, 4579–4588 CrossRef.
- J. Zhou, M. Li, Y. Hou, Z. Luo, Q. Chen, H. Cao, R. Huo, C. Xue, L. Sutrisno, L. Hao, Y. Cao, H. Ran, L. Lu, K. Li and K. Cai, ACS Nano, 2018, 12, 2858–2872 CrossRef PubMed.
- B. Liu, W. Wang, J. Fan, Y. Long, F. Xiao, M. Daniyal, C. Tong, Q. Xie, Y. Jian, B. Li, X. Ma and W. Wang, Biomaterials, 2019, 217, 119301 CrossRef PubMed.
- X. Cai, X. Jia, W. Gao, K. Zhang, M. Ma, S. Wang, Y. Zheng, J. Shi and H. Chen, Adv. Funct. Mater., 2015, 25, 2520–2529 CrossRef.
- L. Hou, X. Gong, J. Yang, H. Zhang, W. Yang and X. Chen, Adv. Mater., 2022, 34, e2200389 CrossRef PubMed.
- X. Cai, W. Gao, M. Ma, M. Wu, L. Zhang, Y. Zheng, H. Chen and J. Shi, Adv. Mater., 2015, 27, 6382–6389 CrossRef.
- L. Xing, X. Y. Liu, T. J. Zhou, X. Wan, Y. Wang and H. L. Jiang, J. Controlled Release, 2021, 339, 14–26 CrossRef.
- J. Feng, W. Yu, Z. Xu and F. Wang, Chem. Sci., 2020, 11, 1649–1656 RSC.
- L. Duan, C. Wang, W. Zhang, B. Ma, Y. Deng, W. Li and D. Zhao, Chem. Rev., 2021, 121, 14349–14429 CrossRef.
- W. Zhu, Z. Chen, Y. Pan, R. Dai, Y. Wu, Z. Zhuang, D. Wang, Q. Peng, C. Chen and Y. Li, Adv. Mater., 2019, 31, e1800426 CrossRef.
- B. Li and H. C. Zeng, Adv. Mater., 2019, 31, e1801104 CrossRef PubMed.
- W. M. Sharman, C. M. Allen and J. E. van Lier, Methods Enzymol., 2000, 319, 376–400 Search PubMed.
- W. Wu, L. Yu, Y. Pu, H. Yao, Y. Chen and J. Shi, Adv. Mater., 2020, 32, e2000542 CrossRef.
- Y. Xiong, Z. Wang, Q. Wang, Q. Deng, J. Chen, J. Wei, X. Yang, X. Yang and Z. Li, Theranostics, 2022, 12, 944–962 CrossRef.
- Q. Sun, Z. Zhou, N. Qiu and Y. Shen, Adv. Mater., 2017, 29, 1606628 CrossRef.
- Z. Zhang, Q. Deng, C. Xiao, Z. Li and X. Yang, Acc. Chem. Res., 2022, 55, 526–536 CrossRef.
- T. Yong, D. Wang, X. Li, Y. Yan, J. Hu, L. Gan and X. Yang, J. Controlled Release, 2020, 322, 555–565 CrossRef.
- P. Tan, H. Cai, Q. Wei, X. Tang, Q. Zhang, M. Kopytynski, J. Yang, Y. Yi, H. Zhang, Q. Gong, Z. Gu, R. Chen and K. Luo, Biomaterials, 2021, 277, 121061 CrossRef.
- H. T. Nia, L. L. Munn and R. K. Jain, Science, 2020, 370, eaaz0868 CrossRef.
- X. Dong, H. J. Liu, H. Y. Feng, S. C. Yang, X. L. Liu, X. Lai, Q. Lu, J. F. Lovell, H. Z. Chen and C. Fang, Nano Lett., 2019, 19, 997–1008 CrossRef PubMed.
- B. Wang, Y. Ding, X. Zhao, X. Han, N. Yang, Y. Zhang, Y. Zhao, X. Zhao, M. Taleb, Q. R. Miao and G. Nie, Biomaterials, 2018, 175, 110–122 CrossRef.
- R. K. Jain, Cancer Cell, 2014, 26, 605–622 CrossRef.
|
This journal is © The Royal Society of Chemistry 2023 |