DOI:
10.1039/D2BM01055K
(Paper)
Biomater. Sci., 2023,
11, 128-139
Bioinspired antifouling and antibacterial polymer coating with intrinsic self-healing property†
Received
6th July 2022
, Accepted 29th October 2022
First published on 3rd November 2022
Abstract
Infections caused by biofouling have become a serious concern in the health care sector. Multifunctional coatings with antifouling and antibacterial properties are widely used to combat these biofouling related infections. However, in practice macro or micro scratches or damages can happen to the coating, which can act as an active site for microbial deposition and destroy the antifouling or antibacterial functionality of the coating. Considering this fact, we have developed an excellent biocompatible and multifunctional coating with antifouling, antibacterial and self-healing properties. In this study, prebiotic chemistry inspired self-polymerization of aminomalononitrile (AMN) was used as a primary coating layer, which acted as a primer to graft vitamin B5 analogous methacrylamide polymer poly(B5AMA) and zwitterionic compound 2-methacryloyloxyethyl phosphorylcholine (MPC) containing polymer poly (MPC-st-B5AMA) by forming strong hydrogen bonds. B5AMA having multiple polar groups in the structure acted as an intrinsic self-healing material and showed an excellent antifouling property against protein and bacteria, maintaining a good hydration layer similar to the MPC containing polymer. To impart the antibacterial property to the coating, silver nanoparticles have also been incorporated, which showed more than 90% killing efficiency against both Gram-positive Staphylococcus aureus (S. aureus) and Gram-negative Escherichia coli (E. coli) bacteria with significant reduction of their adhesion on the surface. Incorporation of self-healing property into the fouling repelling and antibacterial coating can significantly extend the durability of the multifunctional coating, making it promising for biomedical applications.
1. Introduction
Surface deposited microorganisms are the critical sources of biofilm formation, which has become a serious global health concern due to their resistance to antibiotics and resulting chronic infections.1,2 Preventing biofilm formation with a recyclable antifouling3–5 and antimicrobial coating6,7 is a promising approach to control biofilm related infections.8 One of the most common strategies adopted by researchers is to functionalize the surface with a hydrophilic polymer9,10 (such as poly(ethylene glycol),11 zwitterionic polymers12 and glycopolymers13) coating to create a strong hydration layer on top of the surface, which usually helps to reduce the adsorption of foulants such as protein and bacteria. However, this passive strategy is not effective for long term applications and is ineffective toward microbials that attached to the surface.14 Researchers have developed many strategies15–18 to generate dual functional surfaces combining both biocidal and non-adhesive functionalities. Non-adhesive functionality helps to reduce the initial microbial adhesion, while the biocidal property helps to simultaneously kill bacterial cells in contact with the surfaces. To impart the biocidal property to surfaces, researchers either incorporate releasable bacteria killing agents such as nanoparticles,19 antibiotics20 and enzymes21 or modify the surface with antimicrobial functionalities such as quaternary ammonium salts,17 polycations,22 and chitosan23 for contact killing. Although various concepts to protect surfaces from foulants were introduced, there are still many challenges to be overcome for practical applications. In practice, it was found that during storage, transport, and clinical use, accidental mechanical damage such as scratching and abrasion can happen, which significantly affects and alters coating properties, exposing the underlying substrate.10 Since bacteria or other microorganisms could invade through the cracks,24,25 a mechanical damage or defect generated during operations might be the origin for surface fouling and accelerate function failure of the coating. One of the promising solutions to overcome this challenge is to introduce self-healing property into the antifouling and antibacterial coating. Several research studies on self-healing coating development have been reported, including multi-layer polymer brushes,24,25 hydrogels,26,27 microspheres28,29 and nanocomposites.30 Self-healing mechanisms can be categorized into two approaches such as “extrinsic self-healing” and “intrinsic self-healing”.31 In the extrinsic self-healing approach, a large number of microspheres or microcapsules containing a “healing agent” are usually pre-embedded into the material. The crack of the material will cause the release of the healing agent that can reconnect the fractured parts, resulting in the formation of a new healed material. The recyclability of extrinsic self-healing is very limited because it allows the material to heal only a limited number of times depending on the amount of healing agent. In contrast, intrinsic self-healing enables the materials to heal infinitely, which is more suitable for materials under requirements of long operation lifespans. In the intrinsic self-healing mechanism, healing of the mechanical damage of materials happens by the formation of reversible dynamic covalent bonds,32 non-covalent interactions such as hydrogen bonds,33 electrostatic attractions,34 and so on. Most of these intrinsic self-healing activities are stimulated by heat, UV or by tuning the pH,35,36 which in a broad range of physiological conditions is not sometimes applicable. So, there is a strong interest in developing an intrinsic self-healing coating with multiple healing sites at room temperature. In this project, we have developed a simple layer by layer coating system with the intrinsic self-healing property at room temperature in the presence of moisture/water with excellent biocompatibility and antibacterial properties.
To functionalize the surface, mussel-inspired dopamine chemistry has been widely used due to its simplicity, versatility and strong reactivity for secondary functionalization.37,38 Recently, similar to polydopamine coatings, prebiotic chemistry inspired another simple, biocompatible and substrate independent functional coating has been developed by spontaneous polymerization of amine-rich aminomalononitrile (AMN).39,40 The adhesive AMN coating has been utilized as a facile and versatile platform for developing multifunctional coatings, immobilizing antifouling41 and antibacterial polymers in a two-step method.42 In this study, we exploited this unique surface modification technique to develop a rapidly self-healing, anti-fouling and antibacterial surface. The recently reported vitamin B5 analogous hygroscopic methacrylamide (B5AMA)43 compound was used to impart the self-healing property to the coating by forming amide-amide and amide-hydroxyl multiple hydrogen bonds. To better understand the self-healing mechanism of the B5AMA, a homopolymer poly(B5AMA) was synthesized and grafted onto the AMN coated surface by hydrogen bonding interactions between poly(B5AMA) and AMN coating. With a zwitterionic compound 2-methacryloyloxyethyl phosphorylcholine (MPC) and B5AMA a statistical copolymer poly (MPC-st-B5AMA) was also synthesized to graft onto the AMN coating for developing a biocompatible, antifouling and self-healing coating. As the AMN coating can strongly bind silver nanoparticles (AgNPs) from silver nitrate solution,44 AgNPs were also incorporated into the AMN coating to impart the antibacterial property to the coating. The developed multifunctional antifouling, antibacterial and self-healing coating is expected to be a promising material for biomedical application.
2. Experimental section
2.1. Materials
MPC was obtained from Prof. Ishihara's lab (University of Tokyo, Japan). The initiator, 4,4′-azobis (4-cyanovaleric acid) (ACVA), thiazolyl blue tetrazolium bromide (MTT), bovine serum albumin (BSA), aminomalononitrile p-toluenesulfonate 98% and D-(−)-pantolactone were purchased from Sigma-Aldrich and used without further purification. The Live/Dead BacLight Bacterial Viability Kit L-7012 and Micro BCA Protein Assay Kit were obtained from Thermo Fisher Scientific. Escherichia coli (E. coli) (ATCC 25922) and Staphylococcus aureus (S. aureus) (ATCC 25923) (ATCC, USA) were used as model bacteria in all bacterial tests. Luria–Bertani broth (LB) and tryptic soy broth (TSB) (Fisher Scientific, USA) were used as liquid media for E. coli and S. aureus, respectively. Normal human lung fibroblast cells MRC-5. CCL-171™ were used for MTT assay.
2.2. Synthesis of polymers
2-Aminoethyl methacrylamide (AEMA) was synthesized according to the previously established procedure of our group.45 B5AMA monomer was synthesized by simple ring opening chemistry between D-(−)-pantolactone and synthesized AEMA, as previously reported.43 The purity of the synthesized monomers was confirmed by 1H NMR. The homo polymer of B5AMA poly (B5AMA) was synthesized via free-radical polymerization. To synthesize poly (B5AMA), B5AMA (1.55 g, 6 mmol), and initiator ACVA (16.8 mg, 60 μmol) were placed in a 50 mL polymerization tube and dissolved in a mixture of methanol (4.5 mL) and DMF (1.5 mL). After degassing the solution mixture with nitrogen for 30 min, the polymerization reaction was carried out at 70 °C for 18 h. The reaction was terminated by rapid cooling in liquid nitrogen and the resultant copolymer was purified by dialysis against DI water for 48 h. The purified polymer was lyophilized to obtain poly(B5AMA) as a powder. A zwitterionic polymer with a B5AMA pendent poly (MPC-st-B5AMA) was also synthesized via free-radical polymerization. To synthesize poly (MPC-st-B5AMA), B5AMA (774 mg, 3 mmol), MPC (885.8 mg, 3 mmol) and initiator ACVA (16.8 mg, 60 μmol) were placed in a 50 mL polymerization tube and dissolved in a mixture of methanol (4.5 mL) and DMF (1.5 mL). After the same polymerization and purification steps were carried out as mentioned above, the resulting purified polymers were characterized by 1H NMR and Viscotek conventional gel permeation chromatography (GPC) to determine the composition and molecular weight, respectively. 1H NMR spectra of the monomers and polymers were recorded on a Varian 500 MHz spectrometer. The number (Mn) and weight (Mw) average molecular weights of the synthesized polymers were determined using a GPC system equipped with one G5000PWXL TSK-GEL column using 0.5 M sodium acetate/0.5 M acetic acid buffer as the eluent at a flow rate of 1.0 mL min−1. The GPC was calibrated by monodisperse pullulan standards (Mw = 5900–404
000 g mol−1).
2.3. Surface coating
Glass substrates were ultrasonically cleaned with acetone, ethanol and DI water for 30 min, respectively, and dried with air. To modify the cleaned glass surfaces, first the glass surface was immersed in 5 mg ml−1 AMN solution in PBS at pH 8.5 and shaken with a mechanical shaker at 200 rpm for 24 hours to allow for autopolymerisation of AMN, forming a homogeneous AMN coating on the glass substrate. After 24 hours of shaking, the coated glass substrate was gently rinsed with DI water to remove loosely bound AMN aggregates from the surface and air dried. Then the polymer solution (10 mg of polymer in 100 μl PBS buffer) of either poly(B5AMA) or poly (MPC-st-B5AMA) (10 mg of polymer dissolved in 100 μl PBS solution) was added dropwise onto the AMN coated surface and placed at room temperature for 12 h. After 12 hours, the resulting coated surfaces were gently rinsed with PBS buffer to remove unreacted polymers and dried with air. The corresponding coated surfaces were denoted as AMN (substrate coated with AMN only), AMN/B5 (AMN coated substrate with poly(B5AMA)) and AMN/B5/MPC (AMN coated substrate with poly(B5AMA)). To incorporate AgNPs into the coating all the modified surfaces were immersed in 50 mM AgNO3 solution for the in situ deposition of the AgNPs onto the surface.
2.4. Surface characterization
Surface morphology and roughness of all the modified surfaces were observed by atomic force microscopy (AFM) using an Anton Paar Tosca 400 unit in tapping mode under dry conditions. Surface roughness was expressed as root-mean-square (RMS) value. The chemical composition of the polymer modified substrate was determined by X-ray photoelectron spectroscopy (XPS) using either an AXIS Nova or an AXIS Ultra-DLD spectrometer (Kratos Analytical Inc., Manchester, U.K.) with a monochromatic Al Kα source at a power of 180 W (15 kV × 12 mA) and a hemispherical analyzer operating in the fixed analyzer transmission mode. To investigate the surface relative hydrophilicity and wetting properties, static water contact angles of all the modified surfaces were measured using a model 590 goniometer. All the contact angles were measured with a 4 μL water droplet onto the surface at room temperature. Three contact angles were measured for each substrate at three different locations, and their average was recorded as a result. The surface morphology of AgNP loaded surfaces was observed by field emission scanning electron microscopy (FESEM, Zeiss Sigma 300/VP).
2.5. Bacterial adhesion and antibacterial assay
Gram-negative Escherichia coli (E. coli) and Gram-positive Staphylococcus aureus (S. aureus) were used as the model bacteria to evaluate both the bacterial adhesion and antibacterial property of the bare glass and modified glass substrates. A single bacteria colony of E. coli and S. aureus was collected from the cultured Luria–Bertani (LB) and tryptic soy (TS) agar plate, respectively, and cultured in 25 mL of liquid LB broth and TS broth, respectively, at 37 °C for 16 hours. Then the grown bacterial cells were washed with sterile PBS solution through centrifugation at 4000 rpm for 5 min and resuspension in PBS twice. The harvested bacterial cells were resuspended in 1 ml of PBS solution to get the concentrated bacteria and later diluted to an optical density reading at 670 nm (OD 670) of 0.15, corresponding to ∼8 × 109 cells per mL. For bacterial attachment, all the sample surfaces were incubated in E. coli and S. aureus suspensions (8 × 109 cells per mL in PBS, pH 7.4) for 24 h at 37 °C, followed by 3 times gently rinsing with sterile PBS to remove loosely bound bacterial cells. The bacterial cells attached on the surfaces were stained with 50 μL of LIVE/DEAD stain in the dark for 15 min and then washed with PBS gently. After that, each slide was examined using a fluorescence microscope with a magnification of 400×. The live bacteria emitted green light and the dead bacteria emitted red light. The number of bacterial cells was obtained through counting the bacterial cells in five different areas (four corners plus the center) of the sample surfaces and then averaging. The counted number of the attached bacteria was analyzed using the ImageJ software package and represented as cells per cm2. Comparing the number of dead cells to the total number of bacterial cells attached fraction of dead cells was calculated, which represented the antibacterial ability of the AgNP loaded surfaces.
2.6. Kinetic measurement of bacterial growth
To observe the bacterial growth in the presence of the AgNP incorporated coated surface, 10 μl of S. aureus bacterial suspension was added into the 3 ml TSB medium with different coated glass surfaces in a culture tube. The culture tubes with the bacterial suspension in TSB media and coated samples were gently shaken at 100 rpm at 37 °C for 24 hours. At different time intervals the bacterial culture media were withdrawn to measure the optical density (OD) at 600 nm using a spectrophotometer.
2.7. Protein adsorption assay
The sample surfaces (each of 1 × 1 cm2 area) were subjected to the protein adsorption test using Bovine Serum Albumin (BSA) as a model protein and the quantification of the adsorbed protein was performed using a bicinchoninic acid (BCA) protein assay kit according to the manufacturer's instructions. First, the samples were immersed in 2 mg mL−1 BSA solution in PBS and incubated at 37 °C for 3 h and then rinsed with PBS buffer solution to remove loosely bound protein from the surface. Afterward, samples were placed in a 24-well plate, and 1 mL of 2.0 wt% sodium dodecyl sulfate solution was added, shaken, and sonicated for 1 h to separate the absorbed protein from the sample surfaces. After the addition of equal volumes of protein solution and BCA reagent, the 96-well plate was incubated at 37 °C for 2 h. The absorbance of the absorbed protein at 570 nm was measured using a Tecan Genios pro microplate reader. The protein absorbance concentration of samples was calculated using the standard curve. The calculated number of absorbed proteins was expressed as μg cm−2.
2.8. Characterization of the Self-healing process
The Nano Scratch Test (NST) was performed using a sphero-conical diamond indenter (tip radius 200 μm) mounted on a scratch test machine (Anton Paar, Micro Combi Tester, MCT3). With this sphero-conical stylus a 2 mm length scratch was performed on the sample surfaces at a constant load of 5 N at a constant speed. Self-healing properties were characterized by measuring the penetration depth before and after the healing process through performing the cross-scan analysis. After the 2 mm long main scratch, three scanning scratches were done perpendicular to the main scratch with a scanning load of 0.05 N in order to scan the profile of the track at several positions. The Pre-scan usually provides a surface profile of the tested surface before scratch. Any non-uniformity in the flatness of the samples is taken into consideration during pre-scanning. The penetration depth corresponds to the difference between the depth of scratch and the surface profile obtained in the pre-scan step. Comparing the penetration depth of scratch to the penetration depth after healing, the percentage of recovery of the coating can be calculated. After the scratching and healing processes reached completion, the scratch-recovered sample was analyzed using an optical microscope. Along with the nano scratch healing observation, macro scratch healing has also been observed with an optical microscope. The modified surface was scratched with a sharp knife followed by 5 μl of water addition onto the surface for fast healing and the resulting surface was observed under the microscope.
2.9. Cytotoxicity
The cytotoxicity of the sample coating was investigated by performing the MTT assay with normal human lung fibroblast cells MRC-5. CCL-171™. MRC-5 cells were seeded into coated (AMN, AMN/B5 and AMN/B5/MPC coating; following the same procedure as mentioned under the Surface Coating section) 96-well plates in a density of 6000 cells per well with 100 μL of DMEM culture medium (including 10% fetal bovine serum (FBS), 1% penicillin/streptomycin and 1% sodium pyruvate) and incubated at 37 °C for 48 h. After 48 h, the cells were allowed to be further incubated for 3–4 h after the addition of MTT solution (20 μL, 5 mg mL−1 in sterilized PBS). The media were carefully removed, followed by the addition of 100 μL of dimethyl sulfoxide/isopropanol (1
:
1 v/v) solution to dissolve the formazan crystals. After that the absorbance at 570 nm was obtained via a TECAN Genios pro microplate reader and percent cell viability was calculated by comparing the OD values of cells treated with/without the coating. In addition to the observation of cell viability on the coated surface, cell viability of every coating extract has also been investigated following a similar protocol as reported by Asha et al.46
3. Results and discussion
A zwitterionic copolymer poly (MPC-st-B5AMA) and a homo polymer of B5AMA-poly(B5AMA) were synthesized by free radical polymerization with a targeted degree of polymerization (DP) of 100. Prior to that B5AMA monomer was synthesized according to the previous report.43 The purity of the B5AMA and chemical composition of poly (MPC-st-B5AMA) were characterized by 1H NMR (Fig. S1–S3†). The mole content of MPC and B5AMA in poly (MPC-st-B5AMA) was calculated from 1H NMR and was found to be 61% and 39%, respectively, by comparing the integral of the characteristic peak for B5AMA (δ 3.97 ppm) with the characteristic peak integral for MPC (δ 3.67 ppm) (Fig. S3†). The molecular weights of the synthesized polymers are summarized in Table 1.
Table 1 Chemical composition and molecular weights of the synthesized polymers
Polymer |
Composition of copolymer (mol%) |
Molecular weightsb |
In feed |
In polymera |
M
n
|
M
w
|
MPC |
B5AMA |
MPC |
B5AMA |
kDa |
|
Calculated from 1H NMR signal integration.
Obtained from aqueous GPC.
|
Poly(MPC-st-B5AMA) |
50 |
50 |
61 |
39 |
30.2 |
46.5 |
Poly(B5AMA) |
— |
100 |
— |
100 |
17.5 |
23.1 |
The amine rich-AMN coating can strongly graft B5AMA by forming a possible hydrogen bond between the functional hydroxyl group (–OH) of the B5AMA structure and C
O functional group of the proposed AMN structure.40 To evaluate the effect of B5AMA on the AMN coated surface, first a cleaned glass substrate was immersed in AMN solution (5 mg ml−1 in PBS buffer solution, pH 8.5) for 24 h to obtain a homogeneous AMN coating and then poly(B5AMA) and poly (MPC-st-B5AMA) polymer solutions (10 mg of polymer in 100 μl PBS solution) were added dropwise onto the AMN coated surface to achieve a homogeneous layer of antifouling coating (Fig. 1). The resultant surfaces are named AMN/B5 and AMN/B5/MPC, respectively.
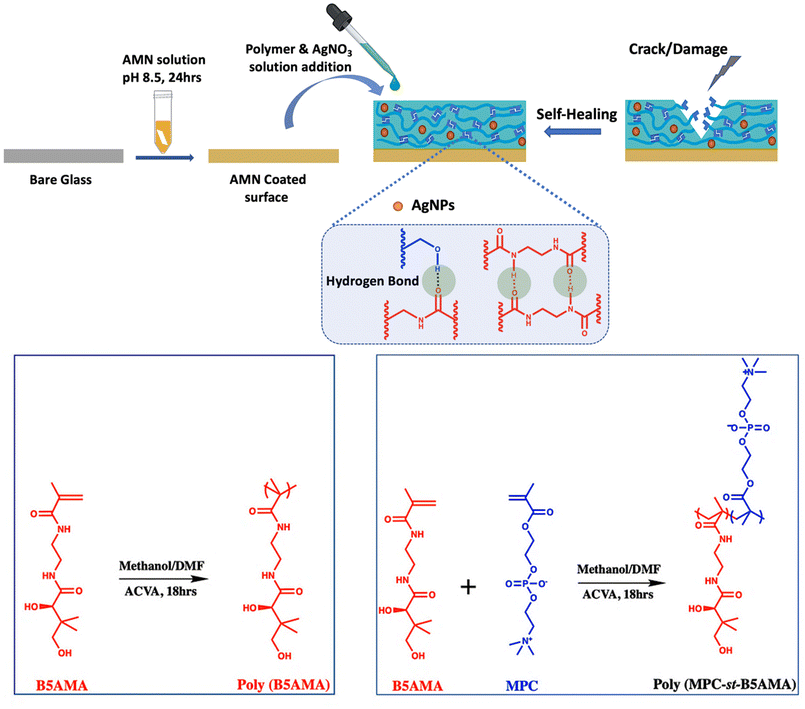 |
| Fig. 1 Schematic illustration of the self-healing coating with a possible hydrogen bond responsible for self-healing. Polymerization mechanism of poly(B5AMA) and poly (MPC-st-B5AMA) polymers with chemical structures. | |
3.1. Characterization of modified surfaces: morphology, composition, and wettability
The surface morphology of all the coatings on glass substrates was characterized by AFM (Fig. 2(a–d)). The AMN surface alone (Fig. 2b) was found to contain surface crystallites spherical in shape, roughening the surface morphology. The roughly spherical features observed by AFM represent the AMN-polymer aggregates expected to result from spontaneous polymerization of AMN. However, they are structurally complex polymers where the exact polymerization mechanism and structure are still under investigation.40 After introducing poly(B5AMA) and poly (MPC-st-B5AMA) polymers onto the AMN coated surfaces, all the surface roughness changed significantly (Fig. 2c and d), showing lower root mean square surface roughness values of 0.6 nm and 1.93 nm, respectively, which also demonstrated that the polymers were successfully grafted onto the AMN coated surfaces by hydrogen bonding interactions.
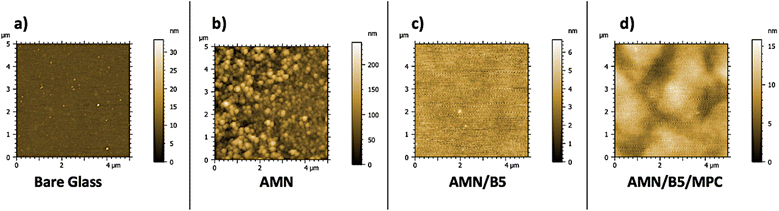 |
| Fig. 2 AFM images of surface morphology of (a) bare glass, (b) AMN, (c) AMN/B5 and (d) AMN/B5/MPC with surface roughness scale bar. | |
The elemental compositions of AMN and composite AMN/B5 and AMN/B5/MPC coatings were determined by XPS, with representative spectra presented in Fig. 3. High resolution spectra of C 1s and N 1s were evaluated qualitatively to confirm the successful grafting of both polymers on the AMN coated glass surface.
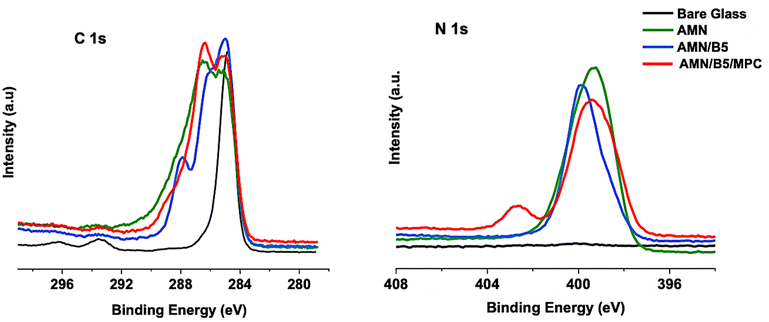 |
| Fig. 3 XPS high-resolution C 1s, and N 1s narrow-scan spectra of the modified glass substrates. | |
Successful AMN coating to the glass surface was indicated by the existence of a strong signal of N 1s peak at 399.2 eV, which corresponds to the nitrile and secondary amine groups present in AMN, whereas in the control bare glass no such strong peak was observed. After introducing the zwitterionic copolymer poly (MPC-st-B5AMA) another strong signal of N 1s at 402.4 eV has appeared. This characteristic peak was attributed to N+(CH3)3 of MPC47 from the polymer brushes of poly(MPC-st-B5AMA), which concludes that the zwitterionic copolymer has been successfully grafted to the AMN coated glass surface. In addition, it displayed a broad signal centered at 399.7–399.2 eV BE, assigned to the expected amide (–HN–(C
O)–) peak from the B5AMA chemical structure and nitrile peak from the primary AMN coating. For the AMN/B5 surface this peak was narrowed down a little and mostly appeared at 399.8 eV, attributed to the amide peak from B5AMA. The nitrile peak for the AMN/B5 surface was not much stronger, which confirms a good coverage of the AMN surface with poly(B5AMA). As grafting of synthesized polymers on the AMN coated surface was mostly by forming a hydrogen bond between B5AMA and AMN, the presence of less amount of B5AMA content in poly (MPC-st-B5AMA) led to less coverage on the AMN surface. Moreover, by analyzing the high-resolution spectra of C 1s, successful attachment of both poly (MPC-st-B5AMA) and poly(B5AMA) polymers on the AMN coated surface was confirmed by the appearance of a characteristic peak of C 1s at 289.1 eV (ester groups) and a strong increase in the peak at 286.4 eV (attributed to C–O and C–N). Appearance of the amide peak at 287.8 eV in C 1s also confirms the presence of B5AMA content in the polymer. As previously reported,39 only the AMN coating is supposed to be highly nitrogenous. We also found a similar result for the AMN coating with a higher N/C atomic ratio compared to other two polymer samples (Table 2). Moreover, N/C and O/C ratios obtained by XPS for the AMN coating were found to be consistent with the proposed chemical structure of AMN.40 After anchoring the synthesized polymers this N/C ratio decreased with an increase of oxygen content. The N/C ratio for the AMN coating was 0.595, which decreased to 0.285 for AMN/B5 and to 0.323 for the AMN/B5/MPC surface. Additionally compared to the control, AMN coating and AMN/B5 surfaces the P/C ratio of the AMN/B5/MPC surface increased from 0 to 0.023, which reflects the native structure of the MPC on the AMN coated surface.
Table 2 Surface compositions determined by XPS. Concentrations are expressed as atomic ratios X/C, i.e., atomic concentration of element X relative to that of C
Surfaces |
Composition (X/C) |
C |
Si |
P |
N |
N+ |
O |
Bare glass |
1.000 |
0.181 |
— |
0.008 |
— |
0.459 |
AMN |
1.000 |
0.032 |
— |
0.595 |
— |
0.229 |
AMN/B5 |
1.000 |
0.024 |
— |
0.285 |
0.006 |
0.299 |
AMN/B5/MPC |
1.000 |
0.043 |
0.023 |
0.323 |
0.038 |
0.379 |
The wetting behavior of AMN and AMN-polymer modified glass surfaces was studied in air with a 4 μL water droplet onto the surface at room temperature. As shown in Fig. 4, the water contact angle (WCA) of the pristine glass substrate was 47.3 ± 2.0°. It was observed that after introducing the AMN coating on the glass substrate the WCA increased to 55.6 ± 1.1°, which is consistent with our previous study48 and can be explained by the higher surface roughness observed by AFM. Both of the polymers grafted on AMN modified glass substrates showed a WCA < 15° and improved hydrophilicity compared to the pristine glass and AMN coating possibly due to the strong interactions between water molecules and hydrophilic polymer chains of B5AMA and zwitterionic MPC. Among them, the AMN/B5 modified glass substrate showed the lowest WCA, 11.5 ± 1.1°, indicating the presence of a stronger surface hydration. The presence of multiple polar groups (hydroxyl and amide groups) in the B5AMA structure enables the stronger interaction with water and the development of a super hydrophilic surface layer.
 |
| Fig. 4 Water contact angle of bare glass, AMN, AMN/B5 and AMN/B5/MPC modified glass substrates. | |
3.2. Antibacterial adhesion and antifouling property of the coating
We evaluated the antibacterial adhesion characteristics of the coatings using Gram-positive Staphylococcus aureus (S. aureus) and Gram-negative Escherichia coli (E. coli) bacteria. The initial attachment of bacterial cells to a surface is considered as the key preliminary step in the biofilm formation processes49 and plays a vital role for evaluating the antifouling properties of the surface. In this study, to evaluate the antibacterial adhesion property of the modified substrates quantitatively, all the coated samples were incubated with the bacterial solution containing 8 × 109 cells per mL for 24 h. After 24 h of incubation, all the sample surfaces were rinsed with PBS to remove loosely bound bacteria and stained using LIVE/DEAD stain for microscopic examination under fluorescence microscopy. As shown in Fig. 5(a) and S4(a),† many green spots (live bacteria) with only a few red spots (dead bacteria) were observed for the AMN coated glass substrate for both E. coli and S. aureus adhesion, indicating the insignificant anti-adhesion efficiency of the AMN coated glass surface due to the higher surface roughness of the AMN surface. After introducing poly(MPC-st-B5AMA) and poly(B5AMA) polymers on the surface the amount of bacterial adhesion was significantly reduced for AMN/B5 and AMN/B5/MPC coatings, indicating high antiadhesion efficiency. The enhancement of this antiadhesion or antifouling property is due to the presence of a strong hydration layer, which might be induced by the hygroscopic nature of poly(B5AMA) and zwitterionic poly (MPC-st-B5AMA) polymers. Compared to S. aureus adhesion, E. coli showed higher adhesion on AMN, AMN/B5 and AMN/B5/MPC surfaces (Fig. 6). Because of the presence of many functional amine groups on the AMN modified surfaces, more negatively charged E. coli might be adhered in greater number on the surfaces. But no antibacterial activity or no dead cells were found in the cases of both AMN/B5 and AMN/B5/MPC coatings.
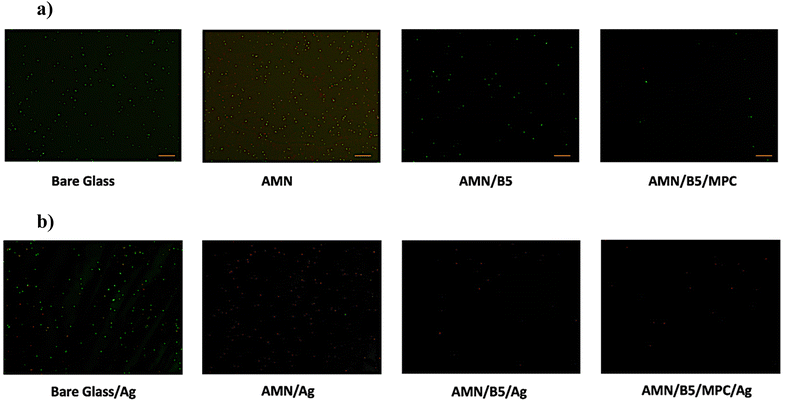 |
| Fig. 5 Fluorescence images of S. aureus adhesion on (a) modified surfaces (bare glass, AMN, AMN/B5, and AMN/B5/MPC). And (b) AgNP deposited modified surfaces (bare glass/Ag, AMN/Ag, AMN/B5/Ag, and AMN/B5/MPC/Ag). Scale bar 20μm. | |
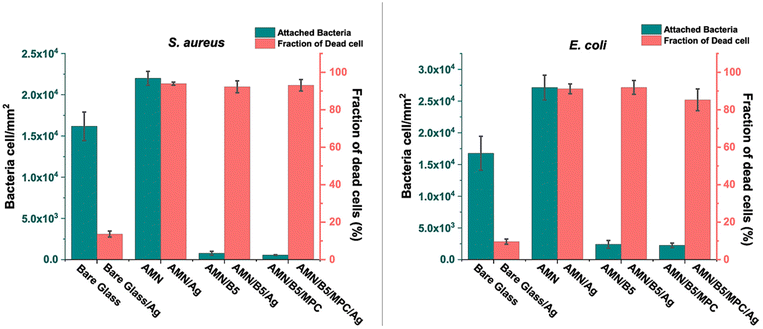 |
| Fig. 6 Attached number of S. aureus (left) and E. coli (right) bacteria on the bare glass and modified surfaces (AMN, AMN/B5, and AMN/B5/MPC); fraction of dead bacterial cells for AgNP deposited surfaces (bare glass/Ag, AMN/Ag, AMN/B5/Ag, and AMN/B5/MPC/Ag). | |
As surfaces with solely the antifouling property cannot achieve long term effectiveness against biofilm formation,42 we incorporated silver nanoparticles (AgNPs) into the coating to impart the antibacterial property by simply immersing the modified surfaces in the AgNO3 solution. As AMN has several electron donors such as amines and nitriles for metal coordination,44 it can help to reduce metallic silver and deposit on the surface. After introducing AgNPs into the coating more than 90% bacteria killing efficiency was obtained for both E. coli and S. aureus due to the release of the Ag+ bactericide agent. The most evident killing mechanism of the Ag+ bactericide agent is the cell membrane perforation and consequent microbial killing, after its accumulation on negatively charged parts of the cellular membrane. From the fluorescence images (Fig. 5(b) and S4(b)†) evidently most of the attached bacteria were found dead for all the modified surfaces except bare glass with AgNPs. For bare glass with AgNPs only 9.625% and 13.65% fraction of dead cells for E. coli and S. aureus, respectively, were found (Fig. 6). Compared to other AgNP loaded surfaces AMN/B5/MPC surface showed relatively low killing efficiency (85.3%) towards E. coli bacteria. Due to the presence of the outer membrane barrier of Gram-negative E. coli, it tends to be more resistant50 to the killing agent and the low concentration of killing agent such as AgNPs51 can also attribute to its lower fraction of dead cells compared to Gram-positive S. aureus bacteria.
To confirm the AgNP deposition and distribution on the modified surfaces, SEM analysis was conducted (Fig. 7). From Fig. 7, it was observed that AMN/Ag surfaces showed a cluster of AgNPs aggregates (white phase) onto the surface with a very rough surface morphology, which supports the high roughness of the AMN coating as well. On the other hand, onto the AMN/B5 surface AgNPs were very homogeneously distributed. Possibly the hydroxyl group of B5AMA can take part in the in situ deposition of AgNPs along with the functional reducing group of AMN. But compared to the AMN/B5 surface the AMN/B5/MPC surface showed less amount of AgNPs onto the surface with some aggregates, which could explain the low killing efficiency towards the E. coli bacteria.
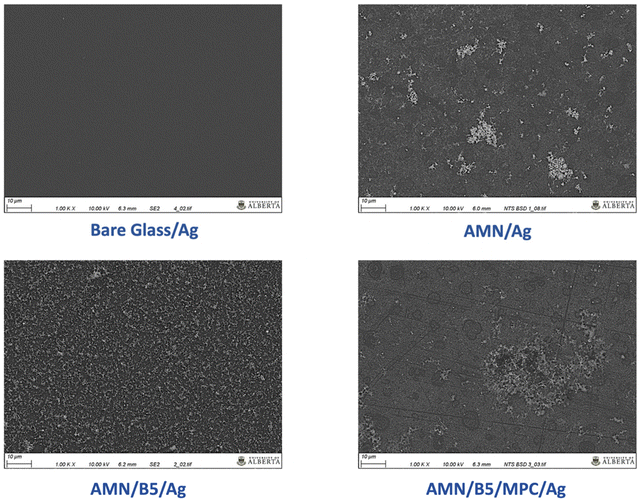 |
| Fig. 7 SEM images and surface morphology of AgNP deposited surfaces – bare glass/Ag, AMN/Ag, AMN/B5/Ag, and AMN/B5/MPC/Ag. Scale bar 10 μm. | |
To understand more about the antifouling property of the coating, every coated surface was challenged against 2 mg mL−1 bovine serum albumin (BSA) as a model protein solution. After 3 h of incubation of the samples with BSA solution at 37 °C, the amount of BSA absorbed on the surface was examined using a BCA assay kit. As shown in Fig. 8, compared to bare glass, the AMN coating showed higher BSA adsorption. High surface roughness and cationic nature of the AMN surface can be attributed to this greater adsorption, which also aligns with our previous study.48 After introducing poly (MPC-st-B5AMA) and poly(B5AMA) polymers on the AMN surface, protein adsorption decreased significantly, suggesting that both B5AMA and MPC moiety into the polymer contributed almost equally to resist protein adsorption on the surface by maintaining a strong hydration layer.
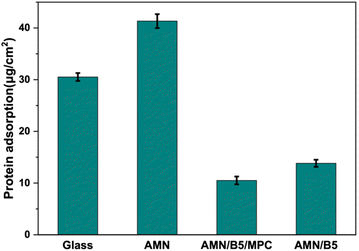 |
| Fig. 8 BSA protein adsorption on glass, AMN, AMN/B5, and AMN/B5/MPC surfaces using BCA protein assay. | |
3.3. Bacterial growth curve
To observe the effect of AgNP release from the surface to bacterial solution, bacterial growth rate has been studied. S. aureus bacterial solution was chosen to observe its growth over time in the presence of AgNP loaded samples. Optical density of the bacterial suspension in media was measured at different time intervals for 24 h. As shown in Fig. 9, with increasing incubation time the bare glass showed rapid bacterial growth after 4 h with increasing OD value. All the AgNP incorporated surfaces showed a slight decrease in growth at the beginning but after 4 h a slight increase in OD value was observed. Compared to the bare glass, the OD values of all the AgNP loaded surfaces were very small, which represents very slow growth of bacteria due to the slow release of the AgNPs from the coating. From Fig. S5,† it is also clearly visible that the turbidity of the bacterial suspension incubation media in contact with different AgNP loaded surfaces was very less compared to the control bare glass. Higher turbidity of the solution represents more bacterial growth.
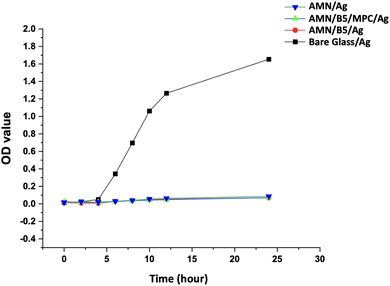 |
| Fig. 9
S. aureus bacterial growth curve with different AgNP containing bare glass/Ag, AMN/Ag, AMN/B5/Ag, and AMN/B5/MPC/Ag surfaces with 24 h incubation. | |
3.4. Self-healing property of the coating
For long-term practical biomedical application of the surface coating, the damage healing property combined with antifouling and antibacterial properties is highly desirable. In practice, anti-bacterial coatings usually failed as they experienced inevitable damage or scratches, leading to the rapid loss or burst release of loaded functional biocides.52 In our developed coating, it is anticipated that B5AMA mostly takes part in the self-healing property by forming amide–amide and amide–hydroxyl multiple hydrogen bonds as demonstrated in Fig. 1. To characterize the self-healing property of the coating, at constant 5 N load, a scratch with a sphero-conical indenter was performed on each sample and the penetration depth was measured using the Micro Combi Tester (MCT3). Both penetration depth of the as scratched and after healing/elastic recovery of every sample was recorded. It is basically a comparative test where the percentage of recovery of the coating has been determined by comparing the penetration depth of the scratched surface to the healed surface. As shown in Fig. 10(b), the bare glass and AMN coating showed negligible percentage of recovery, whereas the AMN/B5 surface showed more than 90% recovery, which proves its outstanding self-healing property. On the other hand, compared to the AMN/B5 surface AMN/B5/MPC showed less percentage of self-healing property, which can be due to the lower concentration of hydrogen bonding sites. As B5AMA mostly provided active sites for hydrogen bond formation for self-healing, higher concentration of hydrogen bonding sites on the AMN/B5 surface might lead to higher possibility of reformation of hydrogen bonds during self-healing.53 For rapid recovery, 5 μl of water droplet was added onto the macro scratch (with a sharp knife random scratch was done on the surface) of the coating, which induced the mobility of the polymer chains and resulted in the reconstruction of the damaged coating within a minute. To detect the coating damage and healing, the most reliable microscopic observation was performed. As shown in Fig. 10(c) and (d) for the AMN/B5 surface, a complete healing of both the nano scratch (done by MCT3) and macro scratch was observed, which also aligns with the surface profile of the nano scratch test (Fig. 10(a)).
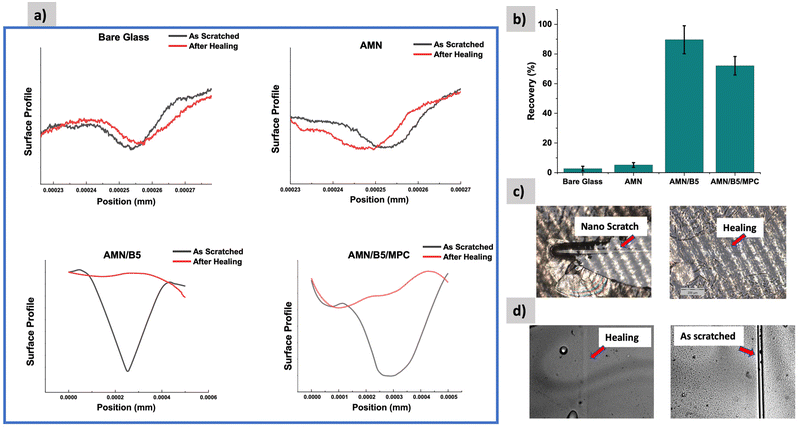 |
| Fig. 10 (a) Penetration depth of the scratch track of the as scratched and after healing in function of the position of Glass, AMN, AMN/B5, and AMN/B5/MPC surfaces. (b) Percentage of recovery of the coating of every surface. (c) Optical microscopic images of the AMN/B5 surface after nano scratch with the Micro Combi Tester and after healing (d) optical microscopic image of the macro scratch on the AMN/B5 surface with a sharp knife and healing within a minute after adding 5 μl water. | |
3.4. Biocompatibility
As an in vitro biocompatibility test, MTT assay was performed on the human fibroblast MRC-5 cells for 48 h to reveal the biocompatibility of the developed surface coatings. As shown in Fig. 11(a), all the developed surfaces showed excellent cell viability against normal fibroblast MRC-5 cells. All the AMN coated surfaces showed more than 100% cell viability. It might be explained by the cell proliferating nature of the AMN coating,44 which resulted in higher percentage of cell viability. Though we hypothesised that incorporation of MPC residues into the coating might enhance the biocompatibility, from the MTT analysis no such significant change has been found for MPC moiety incorporation. The homopolymer poly (B5AMA) grafted surface – AMN/B5 showed excellent biocompatibility similar to the poly (MPC-st-B5AMA) grafted AMN/B5/MPC surface. Thus, MTT analysis clearly demonstrated the non-toxic effect and cell adhesive nature of the coating on the human fibroblast cells, qualifying the developed coating for a broad range of biomedical applications. To better understand the cell toxicity of the AgNP loaded surfaces, MRC-5 cells were incubated for 48 h with AMN/Ag, AMN/B5/Ag, and AMN/B5/MPC/Ag coating extracts. All the coating extracts (Fig. 11b) showed more than 80% cell viability, representing well defined biocompatibility of the AgNP loaded surfaces.
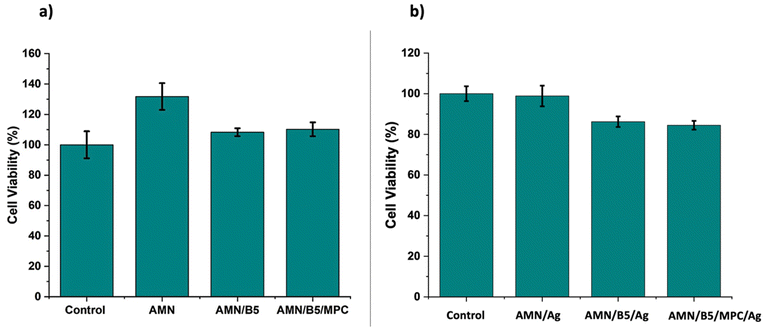 |
| Fig. 11 Cytotoxicity of coated surfaces (a) and AgNP containing coating extracts (b) after 48 h of incubation with MRC-5 cells. | |
4. Conclusion
In summary, utilizing the multiple hydrogen bond, we have reported a new class of self-healing coatings with antifouling and antibacterial properties. In this multifunctional coating, the primary AMN coating layer inspired by prebiotic chemistry not only offered the coating to be universal for a wide range of substrates to strongly graft another functional polymer layer onto the surface but also showed excellent biocompatibility with the cell adhesive property. As the biocompatibility of the B5AMA based coating has not been reported before, to understand and to improve the biocompatibility of the coating, we have combined the B5AMA with the zwitterionic MPC moiety. But compared to the AMN/B5 surface, the AMN/B5/MPC surface did not show any significantly enhanced biocompatibility. The presence of the zwitterionic moiety did not serve any additional advantage. Moreover, the AMN/B5 surface showed an almost similar outstanding antifouling property to AMN/B5/MPC. So, we can conclude that only the poly (B5AMA) coated surface, being hygroscopic in nature, can act as the best antifouling surface with excellent biocompatibility similar to the zwitterionic polymer coated surface. In addition to that, because of the presence of multiple polar functional groups in the B5AMA monomer structure, it serves as a promising self-healing material for biomedical application. In our study, we showed the first report of this self-healing behaviour of the poly (B5AMA) grafted coating. Incorporation of this intrinsic healing material into the antibacterial coating makes this coating system robust for long operation lifespan of a wide range of biomedical applications.
Conflicts of interest
There are no conflicts to declare.
Acknowledgements
This work was financially supported by Natural Sciences and Engineering Research Council of Canada (NSERC). Prof. Michael Serpe is thanked for the help with the use of the goniometer. Dr Thomas Gengenbach at The Commonwealth Scientific and Industrial Research Organization (CSIRO) Manufacturing, Bayview Avenue, Clayton, VIC 3168, is thanked for performing XPS analysis as well as processing XPS data.
References
- M. J. Elder, F. Stapleton, E. Evans and J. K. G. Dart, Eye, 1995, 9, 102–109 CrossRef PubMed
.
- G. Donelli, Adv. Exp. Med. Biol., 2015, 831, 137–146 CrossRef
.
- G. Dai, X. Ai, L. Mei, C. Ma and G. Zhang, ACS Appl. Mater. Interfaces, 2021, 13, 13735–13743 CrossRef CAS PubMed
.
- Z. X. Voo, M. Khan, Q. Xu, K. Narayanan, B. W. J. Ng, R. Bte Ahmad, J. L. Hedrick and Y. Y. Yang, Polym. Chem., 2016, 7, 656–668 RSC
.
- X. Ding, C. Yang, T. P. Lim, L. Y. Hsu, A. C. Engler, J. L. Hedrick and Y. Y. Yang, Biomaterials, 2012, 33, 6593–6603 CrossRef CAS
.
- A. Vaishampayan, A. de Jong, D. J. Wight, J. Kok and E. Grohmann, Front. Microbiol., 2018, 9, 1–14 CrossRef PubMed
.
- Y. Jiao, F. R. Tay, L. na Niu and J. hua Chen, Int. J. Oral Sci., 2019, 11, 1–11 CrossRef CAS PubMed
.
- I. Francolini, C. Vuotto, A. Piozzi and G. Donelli, APMIS, 2017, 125, 392–417 CrossRef PubMed
.
- S. Chen, L. Li, C. Zhao and J. Zheng, Polymer, 2010, 51, 5283–5293 CrossRef CAS
.
- A. M. C. Maan, A. H. Hofman, W. M. de Vos and M. Kamperman, Adv. Funct. Mater., 2020, 30, 2000936 CrossRef CAS
.
- Z. Zhi, Y. Su, Y. Xi, L. Tian, M. Xu, Q. Wang, S. Padidan, P. Li and W. Huang, ACS Appl. Mater. Interfaces, 2017, 9, 10383–10397 CrossRef CAS
.
- A. B. Asha, Y. Chen, H. Zhang, S. Ghaemi, K. Ishihara, Y. Liu and R. Narain, Langmuir, 2019, 35, 1621–1630 CrossRef CAS PubMed
.
- J. S. Gu, H. Y. Yu, L. Huang, Z. Q. Tang, W. Li, J. Zhou, M. G. Yan and X. W. Wei, J. Membr. Sci., 2009, 326, 145–152 CrossRef CAS
.
- J. Liang, J. She, H. He, Z. Fan, S. Chen, J. Li and B. Liu, Appl. Surf. Sci., 2019, 478, 770–778 CrossRef CAS
.
- G. Xu, P. Liu, D. Pranantyo, L. Xu, K. G. Neoh and E. T. Kang, Ind. Eng. Chem. Res., 2017, 56, 14479–14488 CrossRef CAS
.
- G. Cheng, H. Xue, Z. Zhang, S. Chen and S. Jiang, Angew. Chem., Int. Ed., 2008, 47, 8831–8834 CrossRef CAS PubMed
.
- T. Wei, W. Zhan, Q. Yu and H. Chen, ACS Appl. Mater. Interfaces, 2017, 9, 25767–25774 CrossRef CAS PubMed
.
- L. Mi and S. Jiang, Angew. Chem., Int. Ed., 2014, 53, 1746–1754 CrossRef CAS PubMed
.
- R. Tankhiwale and S. K. Bajpai, Colloids Surf., B, 2012, 90, 16–20 CrossRef CAS PubMed
.
- N. Aumsuwan, M. S. McConnell and M. W. Urban, Biomacromolecules, 2009, 10, 623–629 CrossRef CAS
.
- A. Caro, V. Humblot, C. Méthivier, M. Minier, M. Salmain and C. M. Pradier, J. Phys. Chem. B, 2009, 113, 2101–2109 CrossRef CAS PubMed
.
- T. P. Schaer, S. Stewart, B. B. Hsu and A. M. Klibanov, Biomaterials, 2012, 33, 1245–1254 CrossRef CAS PubMed
.
- G. Cado, R. Aslam, L. Séon, T. Garnier, R. Fabre, A. Parat, A. Chassepot, J. C. Voegel, B. Senger, F. Schneider, Y. Frère, L. Jierry, P. Schaaf, H. Kerdjoudj, M. H. Metz-Boutigue and F. Boulmedais, Adv. Funct. Mater., 2013, 23, 4801–4809 CAS
.
- D. Chen, M. Wu, B. Li, K. Ren, Z. Cheng, J. Ji, Y. Li and J. Sun, Adv. Mater., 2015, 27, 5882–5888 CrossRef CAS
.
- Z. Wang, G. Fei, H. Xia and H. Zuilhof, J. Mater. Chem. B, 2018, 6, 6930–6935 RSC
.
- L. Li, B. Yan, J. Yang, L. Chen and H. Zeng, Adv. Mater., 2015, 27, 1294–1299 CrossRef CAS PubMed
.
- Z. Wang, B. Yi, M. Wu, D. Lv, M. L. He, M. Liu and X. Yao, Adv. Funct. Mater., 2021, 31, 1–10 Search PubMed
.
- D. Sun, Y. B. Chong, K. Chen and J. Yang, Chem. Eng. J., 2018, 346, 289–297 CrossRef CAS
.
- G. Wu, J. An, X. Z. Tang, Y. Xiang and J. Yang, Adv. Funct. Mater., 2014, 24, 6751–6761 CrossRef CAS
.
- C. I. Idumah, C. M. Obele, E. O. Emmanuel and A. Hassan, Surf. Interfaces, 2020, 21, 100734 CrossRef CAS PubMed
.
- Y. Yanagisawa, Y. Nan, K. Okuro and T. Aida, Science, 2018, 359, 72–76 CrossRef CAS
.
- M. M. Perera and N. Ayres, Polym. Chem., 2020, 11, 1410–1423 RSC
.
- A. M. Kushner, J. D. Vossler, G. A. Williams and Z. Guan, J. Am. Chem. Soc., 2009, 131, 8766–8768 CrossRef CAS
.
- J. Y. Sun, X. Zhao, W. R. K. Illeperuma, O. Chaudhuri, K. H. Oh, D. J. Mooney, J. J. Vlassak and Z. Suo, Nature, 2012, 489, 133–136 CrossRef CAS PubMed
.
- N. Pathan and P. Shende, Mater. Sci. Eng., C, 2021, 125, 112099 CrossRef CAS PubMed
.
- J. Chen, Y. Huang, X. Ma and Y. Lei, Adv. Compos. Hybrid Mater., 2018, 1, 94–113 CrossRef CAS
.
- W. Z. Qiu, H. C. Yang and Z. K. Xu, Adv. Colloid Interface Sci., 2018, 256, 111–125 CrossRef CAS PubMed
.
- A. B. Asha, Y. Chen and R. Narain, Chem. Soc. Rev., 2021, 50, 11668–11683 RSC
.
- D. J. Menzies, A. Ang, H. Thissen and R. A. Evans, ACS Biomater. Sci. Eng., 2017, 3, 793–806 CrossRef CAS PubMed
.
- R. J. Toh, R. Evans, H. Thissen, N. H. Voelcker, M. D′ischia and V. Ball, Langmuir, 2019, 35, 9896–9903 CrossRef CAS PubMed
.
- W. H. Chen, T. Y. Liao, H. Thissen and W. B. Tsai, ACS Biomater. Sci. Eng., 2019, 5, 6454–6462 CrossRef CAS PubMed
.
- T. Y. Liao, C. D. Easton, H. Thissen and W. B. Tsai, ACS Biomater. Sci. Eng., 2020, 6, 3349–3360 CrossRef CAS PubMed
.
- A. Kabir, M. J. Dunlop, B. Acharya, R. Bissessur and M. Ahmed, RSC Adv., 2018, 8, 38100–38107 RSC
.
- H. Thissen, A. Koegler, M. Salwiczek, C. D. Easton, Y. Qu, T. Lithgow and R. A. Evans, NPG Asia Mater., 2015, 7, 1–9 CrossRef
.
- Z. Deng, H. Bouchékif, K. Babooram, A. Housni, N. Choytun and R. Narain, J. Polym. Sci., Part A: Polym. Chem., 2008, 46, 4984–4996 CrossRef CAS
.
- A. B. Asha, Y.-Y. Peng, Q. Cheng, K. Ishihara, Y. Liu and R. Narain, ACS Appl. Mater. Interfaces, 2022, 14, 9557–9569 CrossRef PubMed
.
- W. Ma, P. Yang, J. Li, S. Li, P. Li, Y. Zhao and N. Huang, Appl. Surf. Sci., 2015, 349, 445–451 CrossRef CAS
.
- Q. Cheng, A. B. Asha, Y. Liu, Y. Y. Peng, D. Diaz-Dussan, Z. Shi, Z. Cui and R. Narain, ACS Appl. Mater. Interfaces, 2021, 13, 9006–9014 CrossRef CAS PubMed
.
- G. Hwang, S. Kang, M. G. El-Din and Y. Liu, Biofouling, 2012, 28, 525–538 CrossRef CAS PubMed
.
- J. M. A. Blair, G. E. Richmond and L. J. V. Piddock, Future Microbiol., 2014, 9, 1165–1177 CrossRef CAS PubMed
.
- J. L. Graves, M. Tajkarimi, Q. Cunningham, A. Campbell, H. Nonga, S. H. Harrison and J. E. Barrick, Front. Genet., 2015, 5, 42 Search PubMed
.
- S. Liu and W. Guo, Adv. Funct. Mater., 2018, 28, 1800596 CrossRef
.
- Y. Zhuo, S. Xiao, V. Håkonsen, T. Li, F. Wang, J. He and Z. Zhang, Appl. Mater. Today, 2020, 19, 100542 CrossRef
.
Footnote |
† Electronic supplementary information (ESI) available: 1H NMR spectra of B5AMA and other synthesized polymers. Fluorescence images of E. coli bacterial cell attachment to different surfaces and AgNP deposited modified surfaces. See DOI: https://doi.org/10.1039/d2bm01055k |
|
This journal is © The Royal Society of Chemistry 2023 |
Click here to see how this site uses Cookies. View our privacy policy here.