Highly polarized [GeOTe3] motif-driven structural order promotion and an enhanced second harmonic generation response in the new nonlinear optical oxytelluride Ba3Ge2O4Te3†
Received
27th October 2021
, Accepted 17th November 2021
First published on 18th November 2021
Abstract
The hybrid anion strategy has been illustrated to be an effective way to design and synthesize high-performance nonlinear optical (NLO) crystals. Herein, a new NLO-active oxytelluride with a highly polarized [GeOTe3] unit, Ba3Ge2O4Te3, has been successfully synthesized. Driven by the distorted [GeOTe3] motif, this compound exhibits interesting structural order promotion with its parent model Ba2ZnGe2O7 (BZGO). Moreover, on the basis of inheriting the polarization structure of BZGO, the Ba3Ge2O4Te crystal possesses an enhanced second harmonic generation (SHG) response benefiting from the partial replacement of O with the more polarized Te. Remarkably, the experimental results and theoretical calculations reveal that Ba3Ge2O4Te3 has the potential to be a promising infrared (IR) NLO material, encompassing a strong SHG response with good phase matchability, wide band gap, high laser damage threshold (LDT), and large birefringence of 0.14@2.09 μm (5.7× AGSe, 10× BZGO) which overcomes the drawback of the small birefringence of AGSe. This work demonstrates that a highly polarized [GeOTe3] motif can be used as a new basic building block (BBB) for IR NLO materials, providing a new idea for the exploration of new functional oxytellurides.
Introduction
Nonlinear optical (NLO) crystals, as a class of important functional materials for photoelectric information, are widely used to convert an existing laser frequency to other favorable wavelength ranges through a number of up- and down-conversion processes including second harmonic generation (SHG), difference frequency generation (DFG), and optical parametric oscillation (OPO).1–7 For application in visible and ultraviolet regions, many excellent NLO crystals have been discovered during the past few decades, including KH2PO4 (KDP),8 KTiOPO4 (KTP),9 β-BaB2O4 (BBO),10 LiB3O5 (LBO),11 and KBe2BO3F2 (KBBF),12 which have largely satisfied the application requirement.
Conversely, the development of IR NLO crystals is falling behind. IR NLO crystals are urgently needed to produce high-power 3–20 μm IR lasers, which have important applications in medical diagnosis, environmental monitoring and homeland security.13–16 However, commercially available IR NLO crystals are limited to a few chalcopyrite crystals such as AgGaS2 (AGS), AgGaSe2 (AGSe), and ZnGeP2 (ZGP), most of which were discovered in the 1970s.17–20 Moreover, these traditional IR NLO crystals have become more and more inadequate in satisfying the development of modern laser technology.21–23
The advantages of these traditional chalcogenide and pnictide IR NLO materials include large NLO coefficients and wide IR transparent ranges covering the atmospheric transparent windows of “3–5 μm” and “8–12 μm”.24–28 However, one of their major drawbacks is the relatively small band gap, which can lead to a low laser damage threshold (LDT) and two-photon absorption (TPA) of the conventional Nd:YAG 1 μm pumping source.29 In contrast, oxide materials usually possess a much larger band gap but much smaller NLO coefficients.30–35 Currently, the hybrid anion strategy provides a broad platform for the imagination of material designers.6,15,36,37 The reasonable combination of two or more anions may have a positive impact on the properties of the material.38–40
Based on these considerations, mixed anionic oxychalcogenides may emerge as a new class of IR NLO materials to achieve the critical balance of a strong NLO response, large band gap, and wide transparent range. Due to the difference in the ionic radius and electronegativity of O2− and Q2− (Q = S, Se, and Te) anions, the mixed-anion building units in oxychalcogenides may show a larger distortion of the coordination polyhedra with enhanced polarity and anisotropy, which can contribute to the strong NLO response and large birefringence.29,41 Besides, diverse combinations of chalcogen and the oxygen element may lead to more new basic building units, enlarging the family of IR NLO materials. A number of oxychalcogenide IR NLO materials have been discovered in recent years, including CaZnSO,42 SrZn2S2O,43 Sr5Ga8O3S14,44 Sr6Cd2Sb6O7S10,29 Sr4Pb1.5Sb5O5Se8,45 AEGeOQ2 (AE = Sr, Ba; Q = S, Se),41,46,47 Sr3Ge2O4Se3,48 and α-Na3PO3S.49 In particular, Sr6Cd2Sb6O7S10 displays a phase-matchable SHG response of more than 4× AGS. However, these materials are all oxysulfides or oxyselenides, and no oxytelluride IR NLO materials have been reported to the best of our knowledge. Theoretically, (1) the electronegativity of the Te atom (χp = 2.10) is lower than those of S (χp = 2.58) and Se (χp = 2.55) atoms, so the electron cloud around Te is easier to polarize, which may be beneficial to produce a NLO response; (2) the IR cut-off edge of the Te-containing compound is red-shifted compared with S/Se analogues, which is beneficial for broadening its transmission range.1,50 Therefore, oxytellurides have attracted our attention as mid- and far-IR NLO materials.
Therefore, in order to produce a highly asymmetric NLO-active mixed-anion basic building block (BBB) and achieve the balance between a strong NLO response and high LDT,51–53 multifunctional Ba2ZnGe2O7 (BZGO) with a noncentrosymmetric structure is used as the parent model, and then the O2− anions are partially substituted by the more polarized Te2− anions to further increase the optical anisotropy and electronic polarization. With efforts, a new NLO-active oxytelluride with a highly polarized [GeOTe3] unit, Ba3Ge2O4Te3, has been successfully discovered. Meanwhile, all Zn2+ cations in Ba2ZnGe2O7 are replaced by equivalent Ba2+ cations to reduce the band dispersion and increase the band gap. Experimental results demonstrate that Ba3Ge2O4Te3 exhibits a strong NLO response (∼0.6× AGSe), and a relatively large band gap (2.08 eV) and LDT (1.3× AGSe). In addition, it possesses a large birefringence of 0.14@2.09 μm (5.7× AGSe, 10× BaZGO), overcoming the drawback of the small birefringence of AGSe. Theoretical calculations demonstrate that the large NLO coefficient comes from the highly polarized [GeOTe3] tetrahedron. This work demonstrates that a highly polarized [GeOTe3] motif can be used as a new basic building block (BBB) for IR NLO materials, providing a new idea for the exploration of new functional oxytellurides.
Experimental section
Reagents
All starting materials, Ba (99.99%), Ge (99.99%), Te (99.999%), BaO (99.9%), and GeO2 (99.9%), were directly purchased from Aladdin Co., Ltd without further purification. The binary materials BaTe and GeTe are synthesised by heating a stoichiometric mixture of the elements in vacuum flame-sealed silica tubes. All manipulations are carried out in an Ar-filled glove box.
Single crystal synthesis
Ba3Ge2O4Te3 single crystals were grown via spontaneous crystallization. 0.207 g (1 mmol) of BaTe, 0.12 g (1 mmol) of BaO, 0.06 g (1 mmol) of Ge, and 0.2 g (2 mmol) of Te were thoroughly ground and loaded into a quartz tube. This tube was slowly heated to 1123 K within 20 h and held for 25 h, then heated to 1223 K within 17 h and maintained for 34 h, gradually cooled to 923 K at a rate of 4 K h−1, and finally the furnace was turned off. Dark red block-like crystals of Ba3Ge2O4Te3 can be obtained with a yield of 30% (based on Ge). These crystals are not sensitive to oxygen and water in the air. A Hitachi S-4800 scanning electron microscope (SEM) was used to perform elemental analysis of the single crystals of Ba3Ge2O4Te3. The results show that the Ba, Ge and Te elements in Ba3Ge2O4Te3 exist in a ratio close to 3
:
2
:
3. It is worth noting that due to the small atomic mass and the oxygen-containing substrate, the O content is not credible.
Structural determination
A Ba3Ge2O4Te3 crystal without cracks was selected for single-crystal X-ray diffraction on an XtaLAB Synergy four-circle diffractometer equipped with a graphite-monochromated Mo-Kα (λ = 0.71073 Å) radiation at 297(2) K. The CrysAlispro software was used for data reduction, and the multi-scan method was used for absorption correction. The structure solution and refinement of Ba3Ge2O4Te3 were solved by direct methods and full-matrix least squares on F2 using the SHELXL-2014 package. The crystallographic data and structure refinement details of Ba3Ge2O4Te3 are presented in Table 1. The atomic coordinates, equivalent isotropic displacement parameters, and bond-valence sum (BVSs) are listed in Table S1 (ESI†). The selected bond lengths and angles are summarized in Table S2 (ESI†).
Table 1 Crystallographic data and structure refinement for Ba3Ge2O4Te3
Empirical formula |
Ba3Ge2O4Te3 |
Formula weight |
1003.98 |
Crystal system |
Trigonal |
Space group |
R3m |
T/K |
297(2) |
a (Å) |
10.9518(4) |
b (Å) |
10.9518(4) |
c (Å) |
8.9740(3) |
α (°) |
90 |
β (°) |
90 |
γ (°) |
120 |
V (Å3) |
932.15(7) |
Z
|
3 |
ρ
calc (g cm−3) |
5.366 |
μ (mm−1) |
21.022 |
Radiation |
Mo Kα (λ = 0.71073) |
2θ range for data |
6.25 to 52.656 |
Index ranges |
−13 ≤ h ≤13, −13 ≤ k ≤ 13, −10 ≤ l ≤ 11 |
Reflections collected |
3989 |
Independent reflections |
[Rint = 0.0391, RSigma = 0.0204] |
GoF on F2 |
1.102 |
Final R indexes [I ≥2σ(I)] |
R
1 = 0.0255, wR2 = 0.0640 |
|
R
1 = 0.0255, wR2 = 0.0640 |
Final R indexes [all data] |
R
1 = 0.0255, wR2 = 0.0640 |
Polycrystalline powder synthesis
The polycrystalline powder of Ba3Ge2O4Te3 was synthesized using a solid-state reaction. A mixture of 0.396 g of BaTe and 0.104 g of GeO2 was evenly ground and placed into quartz tubes, which were sealed under a high vacuum of 10−3 Pa. These samples were gradually heated to 1003 K in 22 h, maintained for 70 h, and finally cooled to room temperature (RT) at a rate of 4 K h−1. A Bruker D8 Focus diffractometer equipped with a Cu-Kα (λ = 1.5418 Å) radiation source was used to perform the powder X-ray diffraction of Ba3Ge2O4Te3. The diffraction pattern from 10° to 70° was collected with the parameters of a scanning step width of 0.02° and a counting time of 0.2 s per step. As shown in Fig. 1, the experimental diffraction pattern is in good agreement with the simulation one, indicating the purity of the polycrystalline sample.
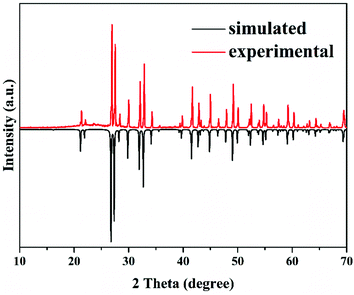 |
| Fig. 1 Simulated and experimental powder X-ray diffraction patterns of Ba3Ge2O4Te3. | |
Optical characterization
A Lab RAM Aramis spectrometer equipped with a 532 nm laser was used to collect the Raman spectrum of Ba3Ge2O4Te3 in the range of 100 to 900 cm−1 at RT. An Excalibur 3100 Fourier transform infrared spectrometer was used to record the IR spectrum in the range of 400–4000 cm−1. The samples for IR measurement were prepared by fully grinding Ba3Ge2O4Te3 crystals with KBr powder (1
:
100 mass ratio). A Cary 5000 UV-vis-NIR spectrophotometer was used to record the diffuse-reflectance spectrum of Ba3Ge2O4Te3 in the range of 2500–200 nm (BaSO4 as a reference).
Thermal properties
A Labsys TG-DTA16 (SETARAM) thermal analyzer was used to evaluate the thermal stability of Ba3Ge2O4Te3. An appropriate amount of Ba3Ge2O4Te3 polycrystalline powder was placed into a quartz tube with a size of 5 mm (o.d.) × 3 mm (i.d.) and eventually sealed the tube at 10−6 Pa. During the measurement process, the nitrogen flow was circulated at a flow rate of about 15 mL min−1, and the temperature of the tube was heated from RT to 1473 K at a rate of 15 K min−1, and then gradually lowered to RT at the same rate.
Second harmonic generation measurement
The SHG intensity of Ba3Ge2O4Te3 was estimated by the Kurtz–Perry technique using a 2090 nm Q-switch Ho:Tm:Cr:YAG laser.54 The powder of Ba3Ge2O4Te3 was sieved into five particle ranges, namely 20–40, 40–90, 90–125, 125–224, and 224–250 μm, and then these samples of different particle sizes were loaded into custom holders with a thickness of 1 mm. In addition, the microcrystals of the traditional material AGSe were sieved to the same particle ranges as mentioned above for reference.
Laser damage threshold measurement
The powder LDT values of Ba3Ge2O4Te3 and the benchmark material AGSe were evaluated by the single-pulse method.55 Glass coverslips were used to place the samples of the same particle size range (40–90 μm), and then fixed with rubber pads in a 1 mm thick aluminum holder. The molds were placed in the experimental light path, and the holders were irradiated using a 1064 nm laser with an τp = 8 ns pulse width. Then, the surfaces of these two samples were observed under an optical microscope with an increase in the laser power until the dazzling light spot emitted by the sample surface due to damage was observed. Finally, the laser power and the area of the damage point were recorded to calculate the LDT values of these two samples.
Theoretical calculations
The first-principles calculations were performed by the method of plane-wave pseudopotential in the CASTEP package according to density functional theory (DFT).56,57 The Perdew–Burke–Ernzerhof (PBE) function was used to describe the exchange-related energy in the generalized gradient approximation (GGA) with a 750 eV kinetic energy cutoff.58 The norm-conserving pseudopotential was chosen to simulate the interaction between the atom cores and the valence electrons with a small plane-wave basis.59 And the total valence electrons are distributed as Ba 5s25p66s2, Ge 4s24p2, Te 5s25p4, and O 2s22p4 electrons. A 3 × 3 × 3 dense Monkhorst–Pack κ-point mesh was selected for Ba3Ge2O4Te3 in the numerical integration of the Brillouin zone.60 The optical properties were calculated by the shift of the conduction band (CB) with the scissor operation to match the experimental value.61 The SHG-weight electron density of virtual electronic (VE) was described by the visualization program and the refractive index was calculated based on the Kramers–Kronics transform.
Results and discussion
Crystal structure
The single crystal diffraction result demonstrates that Ba3Ge2O4Te3 crystallizes in the noncentrosymmetric R3m (No. 160) space group with unit cell parameters of a = b = 10.9518(4) Å, c = 8.9740(3) Å, and Z = 3. The structure of Ba3Ge2O4Te3 is shown in Fig. 2. One crystallographically independent Ba atom, two independent Ge atoms, two independent O atoms (O2 atom has a 33.3% occupancy rate), and one independent Te atom constitute the asymmetric unit of Ba3Ge2O4Te3. Among them, Ba atom, O atoms, and Te atom occupy the Wyckoff 9b positions, and Ge atoms occupy the 3a positions. In addition, Ba, Ge, O, and Te atoms all show normal valence due to the absence of Te–Te or Ge–Ge bonds. Meanwhile, the bond valence theory was applied to calculate the bond valence sums (BVSs) of Ba3Ge2O4Te3, and the results revealed that the valences of Ba, Ge, O, and Te atoms are 2.087, 3.969, −1.973, and −1.954, which are consistent with the structural analysis (Table S1, ESI†).
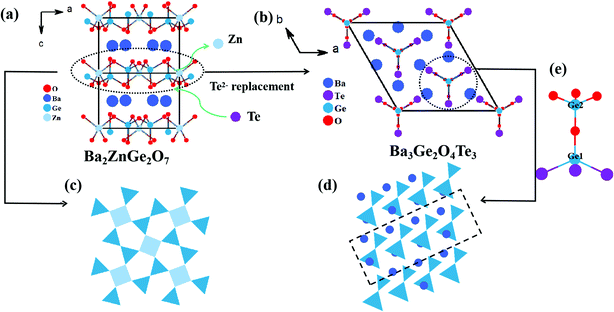 |
| Fig. 2 Structural evolution from Ba2ZnGe2O7 to Ba3Ge2O4Te3. The crystal structures of Ba2ZnGe2O7 (a) and Ba3Ge2O4Te3 (b); the2∞ [ZnGe2O7]4− layer constructed by a corner-sharing [Ge2O7] dimer and [ZnO4] tetrahedron (c), the crystal structure of Ba3Ge2O4Te3 along the [010] direction (d), and the [Ge2O4Te3]6− dimer (e). | |
In the structure of Ba3Ge2O4Te3, the central metal Ge1 atom is connected with three Te atoms and one O atom (O2) to form the [GeOTe3] tetrahedron with d(Ge–O) = 1.8372(447) Å and d(Ge–Te) = 2.5620(14) Å, while the Ge2 atom bonds with four O atoms (three O1 and one O2) to form the classic [GeO4] tetrahedron with Ge–O bond lengths ranging from 1.723 (12) to 1.769(4) Å (Table S2, ESI†). Moreover, the [Ge2O4Te3]6− dimer is composed of [GeOTe3] and [GeO4] tetrahedra by sharing the O2 atom (Fig. 2e). The lengths of these Ge–O/Te bonds are consistent with those of some known compounds, for example, d(Ge–O) = 1.7625 Å in Sr3Ge2O4Se348 and 1.788 Å in SrGeS2O;46d(Ge–Te) = 2.571 Å in Ba2GeTe562 and 2.567 Å in Ba5Ga2Ge3Te12,50 respectively. It can be considered that the zero-dimensional [Ge2O4Te3]6− clusters are arranged along the c axis with the Ba2+ cations located at the interstices built by these clusters (Fig. 2b). The Ba2+ cations are connected with five Te atoms and three O atoms to build up an irregular [BaO3Te5] polyhedron with d(Ba–Te) = 3.5877(15)–3.7967(8) Å and d(Ba–O) = 2.572(12)–2.732(7) (Fig. S2, ESI†), which are consistent with those in the known structures (BaIn2Te4,63 3.580 Å for Ba–Te; BaGeOSe2,41 2.608 Å for Ba–O).
Interestingly, the structure of Ba3Ge2O4Te3 can be viewed to evolve from Ba2ZnGe2O7. The equivalent Ba2+ cation is chosen to substitute the Zn2+ cation in Ba2ZnGe2O7, while three O2− anions are replaced by three Te2− anions, striving to achieve a balance between the wide band gap and strong SHG response. Subsequently, Ba2ZnGe2O7 with 2D2∞[ZnGe2O7]4− layers is transformed into Ba3Ge2O4Te3 with 0D [Ge2O4Te3]6− clusters (Fig. 2c and d). All of the 0D [Ge2O4Te3]6− clusters are aligned in a parallel manner, driving the promotion of structural order. It can be seen that the dimensionality of the structure is inversely proportional to the AE/M value. Similar phenomena can be observed in some systems. For example, SrZn2OS264 possesses 2D wurtzite-like slabs, and BaGeOQ2 (Q = S, Se) features 1D anionic [GeOQ2]2− chains.41,46 In addition, because the ionic radii of Te2− (2.21 Å) and Ba2+ (1.35 Å) were larger than those of O2− (1.40 Å) and Zn2+ (0.74 Å), respectively, the volume of Ba3Ge2O4Te3 (V = 932.15 Å3) is significantly larger than that of Ba2ZnGe2O7 (V = 410.31 Å3), due to the [GeO4], [ZnO4], and [BaO8] units in Ba2ZnGe2O7 being replaced by [GeOTe3] and [BaO3Te5] units, respectively (Fig. S1 and S2, ESI†).
Optical properties
The IR and Raman spectra of Ba3Ge2O4Te3 are shown in Fig. 3. The absorption peaks ranging from 714 to 903 cm−1 in the IR spectrum and the peaks at 669 and 754 cm−1 in the Raman spectrum can be designated as the stretching vibration of Ge–O.41,65 And the peaks in the range of 120–365 cm−1 in the Raman spectrum were attributed to the vibration modes of Ge–Te.50 In addition, the UV-vis-NIR diffuse reflectance spectrum of Ba3Ge2O4Te3 is also shown in Fig. 4. According to the Kubelka–Munk equation, the band gap of the title compound is deduced to be 2.08 eV, which matches well with the yellow-brown color of the Ba3Ge2O4Te3 powder. The bandgap of Ba3Ge2O4Te3 is larger than those of the traditional IR NLO material AGSe (1.83 eV) and many oxygen-free tellurides, such as β-BaGa2Te450 (Eg = 1.19 eV), Cu2CdSnTe466 (Eg = 0.8 eV), PbGa6Te1067 (Eg = 1.11 eV), CsCd4In5Te1268 (Eg = 1.42 eV), etc.
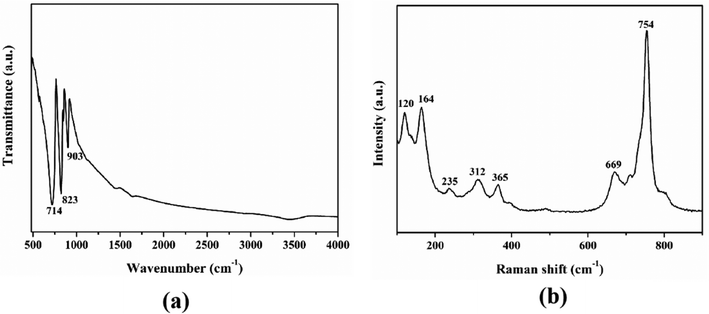 |
| Fig. 3 The IR spectrum (a) and Raman spectrum (b) of Ba3Ge2O4Te3. | |
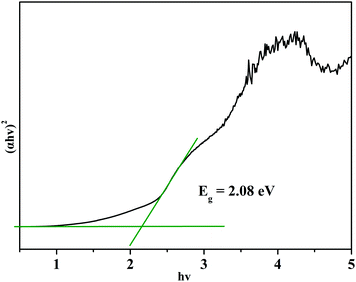 |
| Fig. 4 The UV-vis-NIR diffuse reflectance spectrum of Ba3Ge2O4Te3. | |
Thermal analysis
The thermal properties of Ba3Ge2O4Te3 are shown in Fig. 5. There is a sharp endothermic peak at 1018 °C and two tiny exothermic peaks located at 777 °C and 668 °C during the heating and cooling processes, respectively, implying that Ba3Ge2O4Te3 has a non-congruent melting behavior. Moreover, the melting points of oxychalcogenides are usually lower than those of oxides, which may be due to the longer ionic bond lengths and lower energy required to break the bonds in the former. A similar phenomenon can be observed in BaGeO3 (1270 °C) vs. BaGeOSe2 (920 °C).41,69
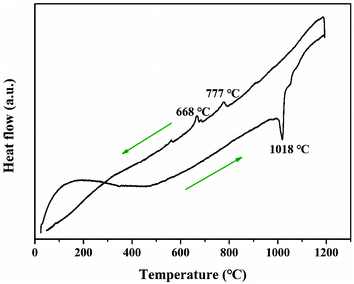 |
| Fig. 5 The DSC curve of Ba3Ge2O4Te3. | |
LDT and SHG properties
The LDT results of Ba3Ge2O4Te3 and AGSe were estimated using the single-pulse method with a 1064 nm, 8 ns Q-switched laser in the particle size range of 40–90 nm, and the spot diameter is 0.4 mm. The energy of the incident laser gradually increased from 0.1 mJ until the damaged spot could be observed with an optical microscope. The cutoff energies of Ba3Ge2O4Te3 and AGSe were determined to be 0.33 mJ and 0.25 mJ, respectively. In addition, the LDT of Ba3Ge2O4Te3 can be calculated as 32.88 MW cm−2, which is about 1.32 times that of AGSe (25 MW cm−2).
The measurement of powder SHG signals of Ba3Ge2O4Te3 and AGSe (as a reference) was estimated using a 2.09 μm laser as fundamental light (Q-switched Ho
:
Tm
:
Cr
:
YAG) based on the Kurtz–Perry method. Obviously, the SHG intensities show a rising tendency with an increase in the particle size and then approach saturation, which indicates that Ba3Ge2O4Te3 can achieve type-I phase-matching (PM) (Fig. 6a). Phase-matching behavior is beneficial to improve the energy conversion efficiency, so it is crucial for the realization of laser applications of IR NLO crystals. Moreover, as demonstrated in Fig. 6b, Ba3Ge2O4Te3 with a strong SHG intensity is about 0.6 times AGSe in the range of 90–125 nm, which is larger than most reported values in oxychalcogenide compounds (Table 2). Obviously, the SHG response of Ba3Ge2O4Te3 is proportional to the sample size, and eventually tends to be saturated, which means that Ba3Ge2O4Te3 can achieve phase matching (PM) (Fig. 6a).
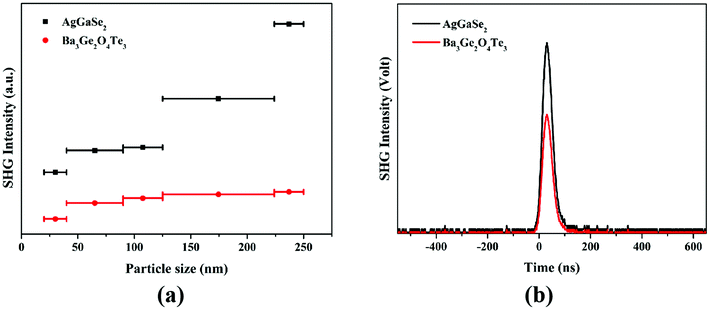 |
| Fig. 6 (a) The phase-matching curves of Ba3Ge2O4Te3 and AgGaSe2 (as a reference), (b) the SHG signals in the size range of 90–125 nm. | |
Table 2 Summary of the properties of known IR NLO oxychalcogenides
Compounds |
Dimension |
E
g
/eV |
d
ij
|
NPM/PMc |
Ref. |
Experimental value.
Powder sample.
NPM = non-phase matchability, PM = phase matchability.
|
Sr3Ge2O4Se3 |
0D |
2.96 |
0.8× AGS |
PM |
48
|
α-Na3PO3S |
0D |
— |
200× SiO2 |
NPM |
49
|
Ba
3
Ge
2
O
4
Te
3
|
0D
|
2.1
|
0.6× AGSe
|
PM
|
This work
|
BaGeOSe2 |
1D |
3.2 |
1.1× AGS |
PM |
41
|
SrGeOS2 |
1D |
3.9 |
0.4× AGS |
NPM |
46
|
BaGeOS2 |
1D |
4.1 |
0.5× AGS |
NPM |
46
|
SrGeOSe2 |
1D |
3.16 |
1.3× AGS |
PM |
47
|
CaZnOS |
2D |
3.71 |
100× SiO2 |
NPM |
42
|
SrZn2OS2 |
2D |
3.86 |
2× KDP |
PM |
43
|
Sr5Ga8O3S14 |
2D |
3.9 |
0.8× AGS |
NPM |
44
|
Sr6Cd2Sb6O7S10 |
2D |
1.89 |
4× AGS |
PM |
29
|
In addition, according to Chen's theory, the macroscopic NLO properties mainly depend on the microscopic anionic group.70 Therefore, the dipole moment (DM) analysis of the [Ge2O4Te3]6− dimer in Ba3Ge2O4Te3 was performed to figure out the origin of the strong NLO effect by using the bond-valence method.71 As mentioned in the structure section, the [Ge2O4Te3]6− dimer is constructed by one [GeO4] tetrahedron and one [GeOTe3] unit via corner-sharing. The DMs of Ge2–O1 and Ge2–O2 are 20.97 D and 19.39 D, respectively. The net dipole moment (NDM) of the [GeO4] tetrahedron is 6.22 D, which is obtained by the vector superposition of the DMs of the four Ge–O bonds, as illustrated by the green arrow in Fig. 7. The NDM of the [GeOTe3] unit is 18.40 D, which is given by the vector sums of one Ge1–O2 with a DM of 19.49 D and three Ge1–Te1 with DMs of 36.07 D, as shown by a yellow arrow. It is worth noting that the directions of the DMs of [GeO4] and [GeOTe3] tetrahedra are opposite, and the value of [GeOTe3] is 3 times that of [GeO4], which means that [GeOTe3] tetrahedra make a huge contribution to the SHG response. Finally, the NDM of the [Ge2O4Te3]6− dimer is 12.18 D, shown by the yellow arrow, which is significantly larger than those of [Ge2O4Se3]6− in Sr3Ge2O4Se3 (3.8 D)48 and [GeO2S2]4− in SrGeOS2 (2.96 D).46
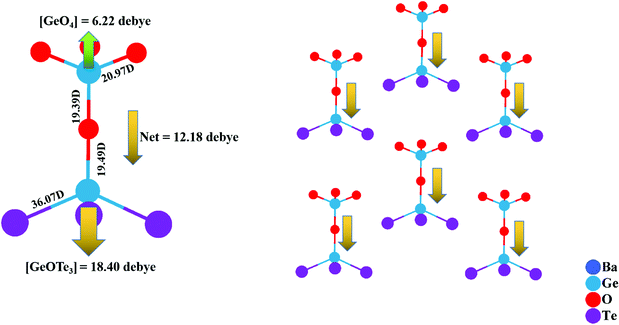 |
| Fig. 7 The dipole moment of the [GeO4] tetrahedron (green arrow) and [GeOTe3] tetrahedron (yellow arrow), and the net dipole moment of the [Ge2O4Se3]6− dimer (yellow arrow). | |
Theoretical calculations
First-principles calculations were performed on Ba3Ge2O4Te3 to help us deeply understand its property–structure relationship. The band structure of Ba3Ge2O4Te3 is shown in Fig. 8b, indicating that it is a direct bandgap semiconductor with a calculated value of 1.79 eV, which is slightly smaller than the experimental one of 2.08 eV. The underestimation of the calculated bandgap is a normal phenomenon, which is due to the discontinuity of the PBE function. Therefore, the scissor operation of 0.2865 eV was applied to shift the CB to ensure that it is consistent with the experimental value. Meanwhile, the calculated total and partial densities of states (DOS) of Ba3Ge2O4Te3 are shown in Fig. 8a. The range from −5 eV to 0 eV in the valence band (VB) are mainly contributed by Ge 4s, Te 5p, and O 2p orbitals. The bottom region of the CB is primarily composed of Ge 4s 4p and Te 5s 5p orbitals. This implies a strong covalent interaction between Ge, Te, and O atoms. The calculated birefringence value of Ba3Ge2O4Te3 is 0.14 at 2090 nm (Fig. 8c), which is approximately 10 times and 5.7 times those of Ba2ZnGe2O7 (0.014 at 2090 nm) and AGSe (0.0245 at 2090 nm), respectively. It is worth noting that such a birefringence value is conducive to achieving PM, which is consistent with the experimental results.
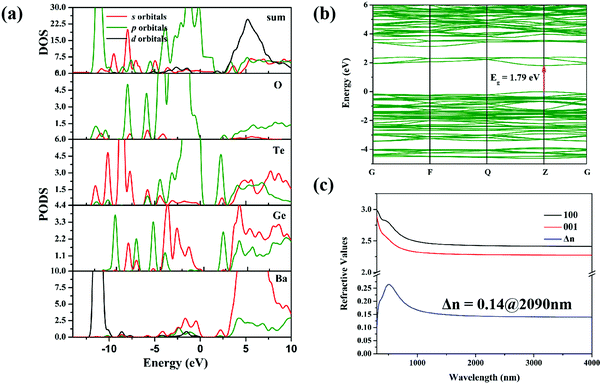 |
| Fig. 8 (a) Total and partial densities of states of Ba3Ge2O4Te3. (b) Calculated electronic band-structure of Ba3Ge2O4Te3. (c) Calculated refractive curves of Ba3Ge2O4Te3. | |
Since Ba3Ge2O4Te3 belongs to the m point group (due to the disorder of the O2 atom, the virtual unit cell approximation method was used in the theoretical calculations, which leads to the transformation of the space group from R3m to Cm), there are six independent second-order NLO tensors under the restriction of Kleinman's symmetry. The results are d11 = 8.8 pm V−1, d12 = −10.6 pm V−1, d13 = 3.7 pm V−1, d15 = 9.1 pm V−1, d24 = 7.7 pm V−1, and d33 = 10.9 pm V−1, respectively, which are significantly larger than that of BaGeOSe2 (2 pm V−1).41 Moreover, the real-space atom-cutting technique was performed to calculate the relative SHG response contribution of constituent units (Table 3). The effective NLO coefficients d33 of [GeO4] and [GeOTe3] units are −2.0 and 10.2 pm V−1, respectively. Obviously, the SHG response of Ba3Ge2O4Te3 mainly comes from the [GeOTe3] unit, and the negative coefficient of the [GeO4] tetrahedron is harmful to the SHG response, which is consistent with the calculated value of DMs. Besides, the SHG-density technique was used to visually describe the main contribution of atoms/groups to the SHG response. Only the virtual electronic (VE) processes were analyzed, because the contribution of virtual holes (VHs) to the SHG effect is negligible. As shown in Fig. 9, there is no SHG density distribution on Ba2+ cations. In contrast, [GeOTe3] and [GeO4]tetrahedra exhibit significant SHG electron density, which indicates that Ba2+ cations have no impact, while [GeOTe3] and [GeO4] tetrahedra have strong SHG activity.
Table 3 Effective NLO coefficients (pm V−1) from real-space atom-cutting analysis of Ba3Ge2O4Te3
|
d
11
|
d
12
|
d
13
|
d
15
|
d
24
|
d
33
|
Ba3Ge2O4Te3 |
8.8 |
−10.6 |
3.7 |
9.1 |
7.7 |
10.9 |
GeO4 |
0.5 |
−0.6 |
1.0 |
−0.7 |
−0.5 |
−2.0 |
GeOTe3 |
7.4 |
−8.3 |
2.5 |
11.4 |
9.4 |
10.2 |
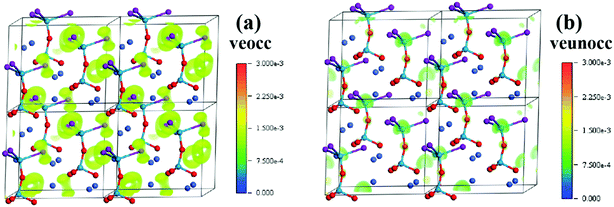 |
| Fig. 9 The SHG-density of virtual electronic (VE) occupied (a) and unoccupied (b) states. | |
Conclusion
In summary, a new SHG-active oxytelluride, Ba3Ge2O4Te3, has been successfully synthesized by taking the multifunctional BZGO as the parent model. It inherits the polar structure of BZGO and remarkably improves the SHG response benefiting from the highly polarized [GeOTe3] motif. Experimental measurements accompanied by theoretical calculations reveal that Ba3Ge2O4Te3 possesses potential as a promising IR NLO crystal, encompassing a strong SHG response (0.6× AGSe), large birefringence (0.14 at 2090 nm), good phase-matching behavior, high LDT (1.32× AGSe) and relatively large band gap (2.08 eV). These results illustrate that the highly polarized [GeOTe3] motif can be used as a new BBB for IR NLO materials. More importantly, the hybrid anion strategy is expected to provide a broader chemical space in the search for new IR NLO materials, and the additional degrees of freedom provided by the selection of multiple anions should allow the adjustment of material properties.
Author contributions
Mengran Sun: validation, characterization, investigation, data curation, writing – original draft, and writing – review & editing. Xingyu Zhang: investigation, theoretical calculations, and writing – original draft. Chunxiao Li: investigation and powder SHG measurement. Wenhao Liu: investigation. Zheshuai Lin: theoretical calculations. Jiyong Yao: resources, data curation, writing – review & editing, supervision, and funding acquisition.
Conflicts of interest
The authors declare no competing financial interest.
Acknowledgements
The authors thank the National Natural Science Foundation of China (no. 22175190) for support. They also thank Wenhao Xing from the Institute of Chemical Materials, China Academy of Engineering Physics, for assistance in the measurement of single crystal diffraction and thermal properties.
References
- X. Luo, Z. Li, Y. Guo, J. Yao and Y. Wu, J. Solid State Chem., 2019, 270, 674–687 CrossRef CAS.
- J. Zhang, D. Clark, J. Brant, K. Rosmus, P. Grima, J. Lekse, J. Jang and J. Aitken, Chem. Mater., 2020, 32(20), 8947–8955 CrossRef CAS.
- I. Chung and M. G. Kanatzidis, Chem. Mater., 2014, 26(1), 849–869 CrossRef CAS.
- T. K. Bera, J. Jang, J. Song, C. D. Malliakas, A. J. Freeman, J. B. Ketterson and M. G. Kanatzidis, J. Am. Chem. Soc., 2010, 132(10), 3484–3495 CrossRef CAS.
- J. Liao, G. M. Marking, K. F. Hsu, Y. Matsushita, M. D. Ewbank, R. Borwick, P. Cunningham, M. J. Rosker and M. G. Kanatzidis, J. Am. Chem. Soc., 2003, 125(31), 9484–9493 CrossRef CAS PubMed.
- Y. Pan, S. Guo, B. Liu, H. Xue and G. Guo, Coord. Chem. Rev., 2018, 374, 464–496 CrossRef CAS.
- V. Petrov, Opt. Mater., 2012, 34(3), 536–554 CrossRef CAS.
- D. Eimerl, Ferroelectrics, 1987, 72(1), 95–139 CrossRef CAS.
- F. C. Zumsteg, J. D. Bierlein and T. E. Gier, J. Appl. Phys., 1976, 47(11), 4980–4985 CrossRef CAS.
- C. Chen, W. U. Bochang, A. Jiang and G. You, Sci. China: Chem., 1985, 28(3), 235–243 Search PubMed.
- C. Chen, Y. Wu, A. Jiang, B. Wu, G. You, R. Li and S. Lin, J. Opt. Soc. Am. B, 1989, 6(4), 616–621 CrossRef CAS.
- B. Wu, D. Tang, N. Ye and C. Chen, Opt. Mater., 1996, 5(1), 105–109 CrossRef CAS.
- P. Gong, F. Liang, L. Kang, X. Chen, J. Qin, Y. Wu and Z. Lin, Coord. Chem. Rev., 2019, 380, 83–102 CrossRef CAS.
- Y. Chu, G. Li, X. Su, K. Wu and S. Pan, J. Solid State Chem., 2019, 271, 266–272 CrossRef CAS.
- Y. Li, W. Wang, H. Wang, H. Lin and L. Wu, Cryst. Growth Des., 2019, 19(7), 4172–4192 CrossRef CAS.
- H. Lin, W. Wei, H. Chen, X. Wu and Q. Zhu, Coord. Chem. Rev., 2020, 406, 213150 CrossRef CAS.
- D. S. Chemla, P. J. Kupecek, D. S. Robertson and R. C. Smith, Opt. Commun., 1971, 3(1), 29–31 CrossRef CAS.
- G. Boyd, H. Kasper, J. McFee and F. Storz, IEEE J. Quantum Electron., 1972, 8(12), 900–908 CrossRef CAS.
- G. D. Boyd, E. Buehler and F. G. Storz, Appl. Phys. Lett., 1971, 18(7), 301–304 CrossRef CAS.
- Z. Li, S. Zhang, Z. Huang, L. Zhao, E. Uykur, W. Xing, Z. Lin, J. Yao and Y. Wu, Chem. Mater., 2020, 32(7), 3288–3296 CrossRef CAS.
- Y. Guo, F. Liang, W. Yin, Z. Li, X. Luo, Z. Lin, J. Yao, A. Mar and Y. Wu, Chem. Mater., 2019, 31(8), 3034–3040 CrossRef CAS.
- M. Zhou, L. Kang, J. Yao, Z. Lin, Y. Wu and C. Chen, Inorg. Chem., 2016, 55(8), 3724–3726 CrossRef CAS.
- W. Xing, F. Liang, C. Tang, E. Uykur, Z. Lin, J. Yao, W. Yin and B. Kang, ACS Appl. Mater. Interfaces, 2021, 13(31), 37331–37338 CrossRef CAS.
- H. Lin, L. Chen, L. Zhou and L. Wu, J. Am. Chem. Soc., 2013, 135(34), 12914–12921 CrossRef CAS PubMed.
- S. Kuo, Y. Chang, I. Chung, J. Jang, B. H. Her, S. Yang, J. B. Ketterson, M. G. Kanatzidis and K. F. Hsu, Chem. Mater., 2013, 25(12), 2427–2433 CrossRef CAS.
- C. Li, W. Yin, P. Gong, X. Li, M. Zhou, A. Mar, Z. Lin, J. Yao, Y. Wu and C. Chen, J. Am. Chem. Soc., 2016, 138(19), 6135–6138 CrossRef CAS PubMed.
- K. Wu, Z. Yang and S. Pan, Chem. Mater., 2016, 28(8), 2795–2801 CrossRef CAS.
- X. Li, C. Li, M. Zhou, Y. Wu and J. Yao, Chem. – Asian J., 2017, 12(24), 3172–3177 CrossRef CAS.
- R. Wang, F. Liang, F. Wang, Y. Guo, X. Zhang, Y. Xiao, K. Bu, Z. Lin, J. Yao, T. Zhai and F. Huang, Angew. Chem., Int. Ed., 2019, 58(24), 8078–8081 CrossRef CAS PubMed.
- Q. Wu, C. Yang, J. Ma, F. Liang, C. Teng and Y. Du, Inorg. Chem., 2021, 60(19), 15041–15047 CrossRef CAS PubMed.
- X. Meng, F. Liang, M. Xia and Z. Lin, Inorg. Chem., 2018, 57(9), 5669–5676 CrossRef CAS PubMed.
- X. Meng, F. Liang, K. Kang, J. Tang, T. Zeng, Z. Lin and M. Xia, Inorg. Chem., 2019, 58(17), 11289–11293 CrossRef CAS PubMed.
- M. Mutailipu, Z. Xie, X. Su, M. Zhang, Y. Wang, Z. Yang, M. R. S. A. Janjua and S. Pan, J. Am. Chem. Soc., 2017, 139(50), 18397–18405 CrossRef CAS PubMed.
- Y. Yang, P. Gong, Q. Huang, G. Song, X. Liu and Z. Lin, Inorg. Chem., 2019, 58(14), 8918–8921 CrossRef CAS PubMed.
- B. Lei, Q. Jing, Z. Yang, B. Zhang and S. Pan, J. Mater. Chem. C, 2015, 3(7), 1557–1566 RSC.
- S. Guo, Y. Chi and G. Guo, Coord. Chem. Rev., 2017, 335, 44–57 CrossRef CAS.
- J. Xiao, S. Yang, F. Feng, H. Xue and S. Guo, Coord. Chem. Rev., 2017, 347, 23–47 CrossRef CAS.
- P. Yu, L. Zhou and L. Chen, J. Am. Chem. Soc., 2012, 134(4), 2227–2235 CrossRef CAS.
- W. Xing, C. Tang, N. Wang, C. Li, E. Uykur, J. Wu, Z. Lin, J. Yao, W. Yin and B. Kang, Adv. Opt. Mater., 2021, 9(15), 2100563 CrossRef CAS.
- B. Liu, X. Jiang, B. Li, H. Zeng and G. Guo, Angew. Chem., Int. Ed., 2020, 59(12), 4593 CrossRef CAS.
- B. Liu, X. Jiang, G. Wang, H. Zeng, M. Zhang, S. Li, W. Guo and G. Guo, Chem. Mater., 2015, 27(24), 8189–8192 CrossRef CAS.
- T. Sambrook, C. F. Smura, S. J. Clarke, K. M. Ok and P. S. Halasyamani, Inorg. Chem., 2007, 46(7), 2571–2574 CrossRef CAS.
- Y. Tsujimoto, C. A. Juillerat, W. Zhang, K. Fujii, M. Yashima, P. S. Halasyamani and H. C. Zur Loye, Chem. Mater., 2018, 30(18), 6486–6493 CrossRef CAS.
- R. Wang, Y. Guo, X. Zhang, Y. Xiao, J. Yao and F. Huang, Inorg. Chem., 2020, 59(14), 9944–9950 CrossRef CAS.
- Y. Wang, M. Luo, P. Zhao, X. Che, Y. Cao and F. Huang, CrystEngComm, 2020, 22(20), 3526–3530 RSC.
- X. Zhang, Y. Xiao, R. Wang, P. Fu, C. Zheng and F. Huang, Dalton Trans., 2019, 48(39), 14662–14668 RSC.
- M. Ran, Z. Ma, H. Chen, B. Li, X. Wu, H. Lin and Q. Zhu, Chem. Mater., 2020, 32(13), 5890–5896 CrossRef CAS.
- W. Xing, P. Fang, N. Wang, Z. Li, Z. Lin, J. Yao, W. Yin and B. Kang, Inorg. Chem., 2020, 59(22), 16716–16724 CrossRef CAS PubMed.
- N. J. Takas and J. A. Aitken, Inorg. Chem., 2006, 45(7), 2779–2781 CrossRef CAS PubMed.
- M. Sun, X. Zhang, W. Xing, Z. Li, W. Liu, Z. Lin, W. Yin and J. Yao, Inorg. Chem., 2021, 60(19), 14793–14802 CrossRef CAS PubMed.
- P. Becker, P. Held, J. Liebertz and L. Bohatý, Cryst. Res. Technol., 2009, 44(6), 603–612 CrossRef CAS.
- A. A. Kaminskii, L. Bohati, P. Becker, J. Liebertz, H. J. Eichler, H. Rhee and J. Hanuza, Laser Phys. Lett., 2010, 7(7), 528–543 CrossRef CAS.
- C. Li, C. Yin, J. Chen, H. Xiang, Y. Tang and L. Fang, J. Eur. Ceram. Soc., 2018, 38(15), 5246–5251 CrossRef CAS.
- S. K. Kurtz and T. T. Perry, J. Appl. Phys., 1968, 39(8), 3798–3813 CrossRef CAS.
- M. Zhang, X. Jiang, L. Zhou and G. Guo, J. Mater. Chem. C, 2013, 1(31), 4754–4760 RSC.
- M. D. Segall, P. J. D. Lindan, M. J. Probert, C. J. Pickard, P. J. Hasnip, S. J. Clark and M. C. Payne, J. Phys.: Condens. Matter, 2002, 14(11), 2717–2744 CrossRef CAS.
- S. J. Clark, M. D. Segall, C. J. Pickard, P. J. Hasnip, M. I. J. Probert, K. Refson and M. C. Payne, Z. Kristallogr. - Cryst. Mater., 2005, 220(5–6), 567–570 CrossRef CAS.
- J. P. Perdew and A. Zunger, Phys. Rev. B: Condens. Matter Mater. Phys., 1981, 23(10), 5048–5079 CrossRef CAS.
- A. M. Rappe, K. M. Rabe, E. Kaxiras and J. D. Joannopoulos, Phys. Rev. B: Condens. Matter Mater. Phys., 1990, 41(2), 1227–1230 CrossRef PubMed.
- H. J. Monkhorst and J. D. Pack, Phys. Rev. B: Solid State, 1976, 13(12), 5188–5192 CrossRef.
- R. W. Godby, M. Schlüter and L. J. Sham, Phys. Rev. B: Condens. Matter Mater. Phys., 1988, 37(17), 10159–10175 CrossRef PubMed.
- J. Prakash, A. Mesbah, S. Lebègue and J. A. Ibers, Solid State Sci., 2019, 97, 105974 CrossRef CAS.
- M. Ishtiyak, S. Jana, S. Narayanswamy, A. K. Nishad, G. Panigrahi, P. P. Bhattacharjee and J. Prakash, J. Alloys Compd., 2019, 802, 385–393 CrossRef CAS.
- Y. Shi, W. Wei, X. Wu, H. Lin and Q. Zhu, Dalton Trans., 2021, 50(12), 4112–4118 RSC.
- J. Hanuza, M. M
czka, M. Ptak, J. Lorenc, K. Hermanowicz, P. Becker, L. Bohatý and A. A. Kaminskii, J. Raman Spectrosc., 2011, 42(4), 782–789 CrossRef CAS.
- Y. Dong, A. R. Khabibullin, K. Wei, Z. Ge, J. Martin, J. R. Salvador, L. M. Woods and G. S. Nolas, Appl. Phys. Lett., 2014, 104(25), 252107 CrossRef.
- S. A. Avanesov, D. V. Badikov, V. V. Badikov, V. L. Panyutin, V. Petrov, G. S. Shevyrdyaeva, A. A. Martynov and K. V. Mitin, J. Alloys Compd., 2014, 612, 386–391 CrossRef CAS.
- F. Liang, L. Kang, Z. Lin and Y. Wu, Cryst. Growth Des., 2017, 17(4), 2254–2289 CrossRef CAS.
- O. Yamaguchi, M. Ki, T. Niimi and K. Shimizu, Polyhedron, 1983, 2(11), 1213–1216 CrossRef CAS.
- N. Ye, Q. Chen, B. Wu and C. Chen, J. Appl. Phys., 1998, 84(1), 555–558 CrossRef CAS.
- P. A. Maggard, T. S. Nault, C. L. Stern and K. R. Poeppelmeier, J. Solid State Chem., 2003, 175(1), 27–33 CrossRef CAS.
Footnote |
† Electronic supplementary information (ESI) available. CCDC 2112888. For ESI and crystallographic data in CIF or other electronic format see DOI: 10.1039/d1tc05177f |
|
This journal is © The Royal Society of Chemistry 2022 |