Enhanced fatigue resistance and lattice dynamics induced by the strong local strain in Fe-doped KTa1−xNbxO3 single crystals†
Received
18th September 2021
, Accepted 22nd November 2021
First published on 23rd November 2021
Abstract
Improving the durability of lead-free-doped piezoelectric materials is very important for their practical application. However, the promotional mechanism of anti-fatigue properties and the impact on local structures of ion dopants should be further revealed. Here, we investigate the improvement of fatigue resistance in Fe-doped KTa1−xNbxO3 single crystals and reveal the origin of enhanced anti-fatigue properties. Fe-doping gives rise to the promotion of strain (by more than two times) and large enhanced anti-fatigue properties (only an 11.5% drop after 105 electric cycles). Furthermore, the dynamic evolution of doped lattices during the fatigue process is experimentally characterized. The lattice contraction and the stability of doped lattice vibration modes are demonstrated, leading to the stabilization of Eib and the enhanced recoverability of domain structures. Moreover, HRTEM reveals that the restriction of oxygen vacancies and the stable lattice vibrations are improved via the strong local strain fields induced by lattice distortion, which is fundamentally responsible for the enhancement of the fatigue resistance. The study revealing the promotional origin of anti-fatigue provides an effective strategy to design the local strain fields by doping modification to optimize the properties of ferroelectrics, which is greatly significant in the practical application of lead-free ferroelectrics.
Introduction
Improvement of durability of lead-free piezoelectric materials (i.e. transduces, actuators, etc.) is of great significance in their practical application.1,2 Both theoretical and experimental results confirm that ion doping is able to obviously modify the properties of ferroelectrics.3–7 With the awareness of environmental protection, the exploration of lead-free piezoelectrics has attracted more attention from researchers, such as BaTiO3 (BT),8–10 (K1−xNax)NbO3 (KNN),11–13 and (Ba,Ca)(Ti,Zr)O3 (BCZT).14 Numerous investigations focus on the doping-induced changes of aging,15,18 strain,16,17 and thermal features19 of lead-free materials. Mn doping largely improves the electric-field-induced strain of BT crystals to 0.75%.18 Fe doping enhances the electrocaloric effect of BCZT by design composition.14 Fe achieves a visible-light photovoltaic effect in BiFeO3 crystals.19 On the basis of the above-mentioned research studies, the properties of lead-free materials can be improved after appropriate Fe doping. However, the dynamic evolution of doped lattices in the fatigue process needs further research. The promotional mechanism of the anti-fatigue properties and the impact on the local structures of ion dopants should be further revealed.
Potassium tantalum niobate (KTa1−xNbxO3, KTN) single crystals exhibit excellent optical,20–25 ferroelectric,26–29 and piezoelectric30–32 properties. Doping directly affects the performance of KTN crystals. However, the anti-fatigue properties of KTN single crystals are still scarcely investigated, which affects the practical applications of KTN. Meanwhile, the effects of ion doping on fatigue resistance in KTN single crystals have not been adequately revealed to date.46 Hence, it is of importance to clarify the mechanism of how doping of ions contributes to the anti-fatigue properties to achieve the practical applications and the commercial markets of KTN.
This work achieves the improvement of fatigue resistance in Fe-doped KTN (Fe-KTN) single crystals and reveals the promotional mechanism of the anti-fatigue properties. Fe-doping gives rise to the promotion of electric-field-induced strain (by more than two times) and anti-fatigue properties (only an 11.5% drop after 105 cycles) compared with pristine KTN (a 69.3% drop). Meanwhile, the dynamic evolution of doped lattices in the fatigue process has been experimentally demonstrated, yielding the stabilization of the internal bias fields (Eib) and the recovery of the domain structures. Moreover, HRTEM shows that the restriction of oxygen vacancies and the recoverability of the lattice vibration are enhanced via the strong local strain fields inside the Fe-KTN, which is fundamentally responsible for the improvement of the anti-fatigue properties in Fe-KTN single crystals. Furthermore, revealing the origin of anti-fatigue is beneficial for designing the local distributions of strain fields by doping ions to improve the properties of lead-free materials, as well the reference for doping modification studies in other ferroelectric material systems.
Experimental details
The pristine and Fe-doped KTN single crystals were grown using a modified top-seeded solution growth method.33,34 The samples were cut into a rectangular prism with dimensions 2.00[100]c × 2.00[010]c × 1.00[001]c mm3 along the crystallographic directions, and gold electrodes were sputtered on both (001)c facets. All the single crystals were heated to 100 °C at a heating rate of 1 °C min−1 and were kept at 100 °C for two hours. Then the temperature of samples was cooled to room temperature at a rate of 1 °C min−1. The samples were aged for two weeks before our experiments.
Temperature dependence of dielectric permittivity (εr) and dielectric losses (tan
δ) were measured by using an inductance–capacitance–resistance (LCR) meter (E4980A, Agilent Technologies) at 100 kHz, applying a 1 V probe voltage to both gilded surfaces at the heating rate of 1 °C min−1. Polarization–electric field hysteresis loops (P–E) and strain–electric field loops (S–E) were measured using a modified ferroelectric test system (Precision Premier II, Radiant Technology, Inc., Albuquerque, NM). The P–E loops of Fe-KTN and pristine KTN were measured at 50 Hz under different electric fields (from 2.0 kV cm−1 to 15.0 kV cm−1). A cyclic electric field (12 kV cm−1 at 100 Hz, number of cycles increased from 102 to 105) was applied to characterize the fatigue properties. All the electrical experiments were carried out at room temperature (26 °C). All the samples were not poled before test.
The evolution of the domain structure before and after the electric field cycles was observed on a polarizing microscope (Axioskop40, Zeiss). The polarization microscopy images were measured at room temperature (26 °C) along the [001] direction. The Raman spectra (632.8 nm) were measured on a Renishaw inVia confocal micro-Raman spectroscopy system. The reflection mode of room-temperature X-ray diffraction (XRD-6000, Shimadzu, Kyoto, Japan) was applied to the bulk samples. A bright-field high-resolution transmission electron microscope (HRTEM, FEI Talos F200X) was used to obtain the microscopic atomic arrangement of Fe-KTN and KTN along the direction of (010)c. Please see the Supplemental Material for details of experimental conditions.
Results and discussion
Improvement of fatigue resistance
As shown in Fig. 1(a), the peak of dielectric permittivity exceeds 8
000 and the dielectric loss is less than 0.03 at Tr, which proves the high quality of the as-grown 0.5 mol% Fe-doped crystal and pure KTN. The element atomic percentages of these single crystals are shown in Table S1 and Fig. S1 (ESI†) by the energy dispersive X-ray analysis. The Fe atomic percentage (0.49 mol% for test) is consistent with the growth components (0.5 mol%) we designed. The Curie temperature (Tc) of these similar-component single crystals is around 65 °C, and all the samples are in the tetragonal phase at room temperature (Tr). Furthermore, the dielectric loss is greatly reduced (< 0.01 at Tr) with a slight decrease in the dielectric properties after Fe doping, which is the characteristic of acceptor doping. Additionally, the εr and the tan
δ displayed a dramatic drop during the ferroelectric phase transitions (at TO–T) after Fe doping. These changes are also characteristics of acceptor doping.
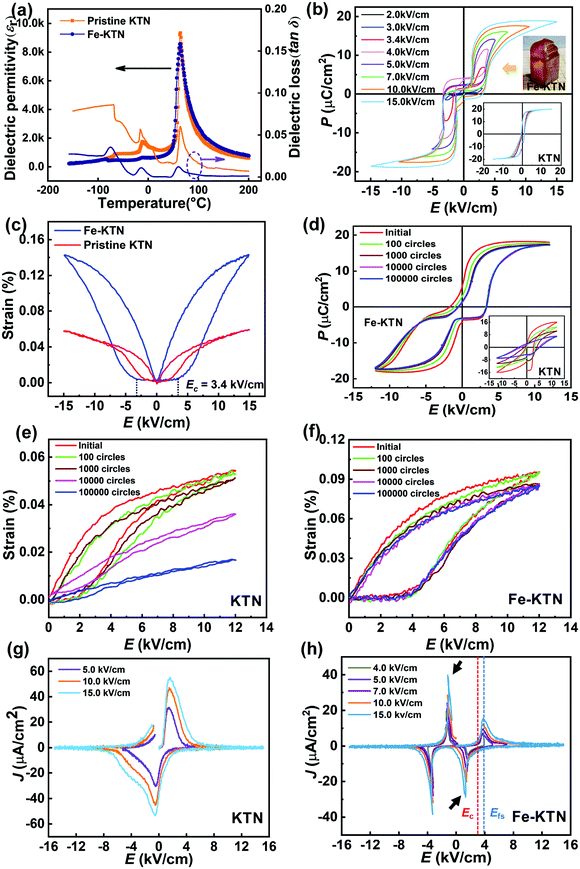 |
| Fig. 1 (a) Temperature dependence of dielectric permittivity (εr) and dielectric losses (tan δ) of Fe-KTN and pristine KTN single crystals. (b) The P–E loops of Fe-KTN. The insets show the picture of the as-grown Fe-KTN single crystals and the P–E loops of pristine KTN. (c) The S–E of pristine KTN and Fe-doped KTN. (d) The P–E loops of Fe-KTN and KTN (the inset) after electric cycles. The unilateral S–E of the (e) pristine KTN and the (f) Fe-KTN after electric cycles. The J–E curves of the (g) Fe-KTN and the (h) pristine KTN. | |
The polarization–electric field hysteresis loops (P–E) of these single crystals under external electric fields are shown in Fig. 1(b). The amplitude of electric fields (E) ranges from 2 to 15 kV cm−1. Different from KTN single crystals, the P–E loops of Fe-KTN exhibit the characteristics of double hysteresis loops, and the coercive field Ec (∼3.4 kV cm−1, Fig. S2, ESI†) significantly increases (Ec ∼ 1.2 kV cm−1 in pristine KTN). Generally, the double loops are closely related to the defects in materials. According to the symmetry-conforming principle of point defects,18 the defect dipoles are induced via doping Fe ions and the generated oxygen vacancies in Fe-doped KTN,30 which is the reason for the double P–E loop. When applying an appropriate E to reorient the spontaneous dipoles, the arranged spontaneous dipoles determine the macroscopic polarization response. However, after removing E, the local defects provide a restoring force to drive the spontaneous dipoles. The restoring force is macroscopically reflected in the internal bias fields (Eib), which turns the domain structures back to their initial states. Simultaneously, the macroscopic polarization also returns to zero.
The Eib helps the ferroelectric to return to the initial state, which is effective for improving the anti-fatigue properties. The stability of strain is direct evidence of an anti-fatigue performance. The strain–electric field loops (S–E) of pristine KTN and Fe-KTN were measured as shown in Fig. 1(c). The Fe doping (∼0.14%) increases the electric-field-induced strain of KTN (∼0.06%) by more than two times at 15 kV cm−1. Furthermore, the fatigue characteristics were characterized as shown in Fig. 1(d–f). Generally, acceptor doped ferroelectrics often display larger fatigue behaviors.36 However, as shown in Fig. 1(d), the maximum polarization Pmax of Fe-KTN does not decrease greatly after 105 cycles of electric field and the hysteresis maintains a good double loop with almost constant Eib. In contrast to the pristine KTN, the Pmax reduces considerably and the hysteresis loop gradually deforms with Eib approaching zero. What's more, the unilateral electric-field-induced strain of Fe-KTN is significantly improved from a 69.3% drop to an 11.5% drop after 105 cycles compared with that of undoped KTN as shown in Fig. 1(e and f). The fatigue resistance of single crystals is significantly improved after Fe doping.
The change of the Eib after doping plays an important role in the recovery of the domain during electric cycles. The current density J can characterize the connection between domain reversal and Eib.35 The current density (J–E) curves corresponding to the P–E are shown in Fig. 1(g and h). It is worth noting that Efs (∼4.0 kV cm−1) at the maximum switching current is larger than Ec. This indicates that Fe doping introduces an internal bias field of ∼0.6 kV cm−1, which is much larger than Eib of KTN (∼0.2 kV cm−1). The larger Eib of Fe-KTN may be related to the synergistic influence of the limitation to oxygen vacancies and the stronger pinning effect. Compared with pristine KTN, the Fe-KTN has a dramatic domain switching process below Ec as indicated by the black arrows in Fig. 1(h). It suggests that Eib in the Fe-KTN single crystals has a recoverable effect on the polarization state of domains, leading to an improvement of anti-fatigue.
The recoverability of macro-domain structures
The improvement of the anti-fatigue properties of Fe-KTN single crystals is shown in Fig. 2(a), and the strain of Fe-KTN almost stays constant. Macroscopically, the fatigue of KTN is directly impacted by the behaviors of domains during the electric field cycles. The state of the domain can be adjudicated by the scaling relation of the energy dissipation which relates the hysteresis area (〈A〉) to the amplitude (E) and frequency (f) of the applied electric fields.37 The relation is expressed as 〈A〉 ∝ fαEβ, where α and β are controlled by the domain states and the polarization switching mechanism. α refers to the response time of domain under a given electric field, and its value is dominated by the defects and oxygen vacancies.38,39 The larger the absolute value of α, the longer the response time to the electric field. The variable-frequency polarization (Fig. S3, ESI†) response of the single crystal was measured to fit α. Here, we calculate the logarithm of the scaling relation to obtain α, the results of which are shown in Fig. 2(b).
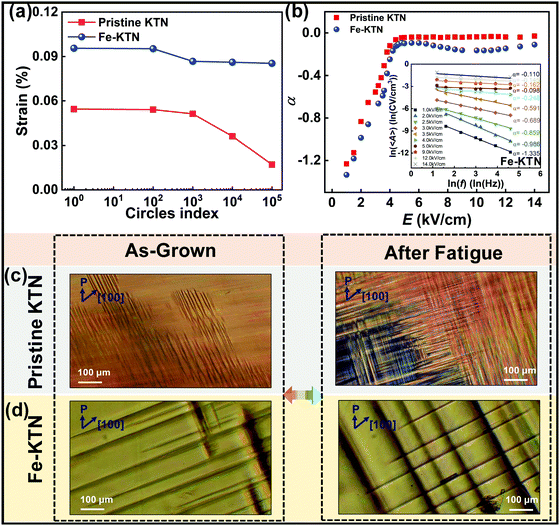 |
| Fig. 2 (a) The decreases of sample strains after the same cycles of electric field. (b) The dependence of the exponent α on E. The inset shows the calculation of the logarithm of the scaling relation to obtain α by fitting. The polarization microscopy images of the domain structure of Fe-KTN (d) and pristine KTN (c) before and after the electric field cycles. | |
Obviously, the absolute value of α of pristine KTN is less than that of Fe-KTN, which means that Fe-KTN needs a much longer response time than on KTN under the same external electric field. Fig. 2(b) further clearly implies that doping Fe affects the macroscopic domains, making the size of domains in the doped single crystal larger than that in the undoped.
To directly observe the influence of doping on domains before and after the electric field cycles, the changes of domain structures of Fe-KTN and pristine KTN are shown in Fig. 2(c and d) using a polarizing microscope. Before fatigue, the two single crystal samples had similar 90° domain structures. The size of the Fe-KTN domain structure was larger than that of KTN, and the domain walls of Fe-KTN were more prominent and straight, which corresponds to the above-mentioned domains in Fig. 2(b). Interestingly, the curved domain walls were observed in the undoped sample, which may be related to the high disorder of the domain inside KTN. After fatigue, the KTN exhibited tiny-periodic domain structures without poling and some cracks appeared inside the sample (the black domain area in Fig. 2(c)). However, because of the high Eib in Fe-KTN samples, the domain structures almost remain in their initial state, and the prominent-straight domain walls do not change drastically. The recoverable domain of Fe-KTN makes the strain stable.
The source of Eib needs to be further clarified, which may be associated with the local structure
induced by Fe doping.17 The relationship between the mechanism of fatigue and the domain-switching dynamics is revealed as shown in Fig. 3, which can be regarded as the improved domain-pinning models.19 The migration of oxygen vacancies
plays a decisive role in the fatigue properties of ferroelectrics.40,41 Initially,
are randomly distributed in the domain structures. In pristine KTN, the unstable
, which were introduced by volatilization of K+ in growth (high temperature environment above 1000 °C), gradually migrate and accumulate at the domain boundaries by electric cycles. Due to the pinning effect of
, the switching of domain structures is suppressed when the electric field is applied, leading to more domain walls appearing near the
under the cyclic fatigue. Finally, the strain of KTN drops sharply with the Eib reducing to zero, and the tiny-periodic domain structures with the above-mentioned cracking are produced.
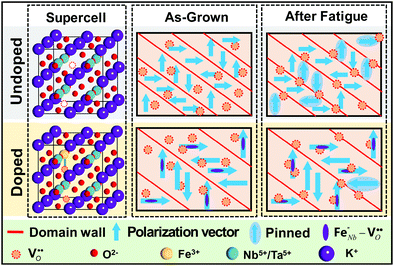 |
| Fig. 3 The improved domain-pinning models are proposed to signal the enhanced restriction of induced by the local structure . The supercells of (un)doped KTN are listed in the first column. The schematic diagrams of the tetragonal domain structures of as-grown (Fe-)KTN are listed in the second column. The schematic diagrams of the domain structure of fatigued (Fe-)KTN are listed in the third column. | |
After Fe doping, Fe occupies the site of Nb5+/Ta5+ thereby combining with the surrounding
to form the stable local structure
(as the supercell shown in Fig. 3), which was also reported in KNN ceramics doped with Fe.17 For the Fe-KTN single crystals, the local structure
limits the migration of
under the electric cycles and maintains the Eib to provide a recoverable ability of domain structures. As a result, the macro-domain structures of Fe-KTN are kept stable and the fatigue resistance is enhanced. In addition, the restriction of
caused by
promotes the full response of the domain under the electric field, which leads to the strain increase after appropriate doping.
The lattice contraction and the recoverability of the lattice vibration modes
The lattice vibrational modes of single crystals are changed because of the generation of the stable local structure
and the limitation on
by
, which are revealed via Raman spectra. The normalized Raman spectra of the as-grown samples are shown in Fig. 4(a), and there are three important vibration peaks about the KTN single crystal. The A1(2TO) mode (∼199 cm−1) is related to the reverse movement between the B-site ion and the oxygen octahedron of KTN.42 The B1 + E(3TO) mode (∼278 cm−1) is connected with the wing-flapping vibration in the oxygen octahedron. The A1(3TO) mode (∼557 cm−1) is associated with the distortion of the oxygen octahedron.43,44 The schematic diagrams of the vibration modes are shown in Fig. 4(c). The distortion of lattices and the disorder of domain structures can be characterized by these vibrational modes.42 It is obvious that the domain structure of Fe-KTN are more ordered than that of KTN because of the larger intensities of the B1 + E(3TO) and A1(3TO) modes (Fig. S4, ESI†). That is why the initial domain walls of Fe-KTN are more prominent and straight compared to those of pristine KTN via observation using a polarizing microscope. What's more, a blue shift (∼5 cm−1) happens in the Raman spectrum as indicated by the arrow in Fig. 4(a), which means that the bond length inside Fe-KTN is shorter than that in KTN after Fe doping. The shorter bond length implies the lattice contraction caused by the generation of the stable local structure
.
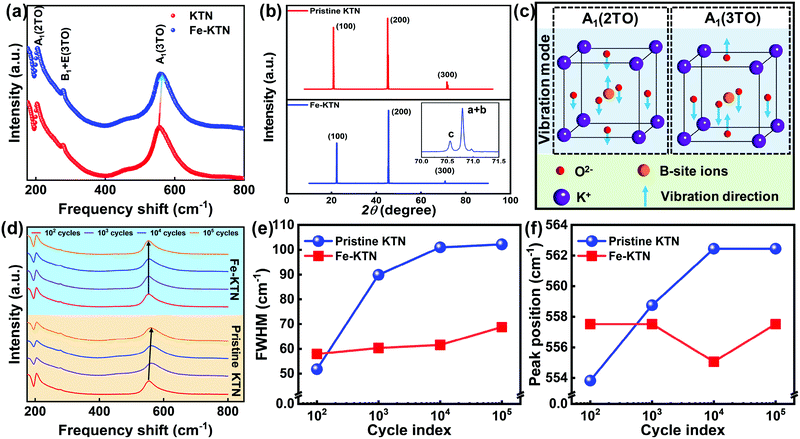 |
| Fig. 4 (a) The normalized Raman spectra from 175 cm−1 to 800 cm−1 of as-grown Fe-KTN and KTN. (b) The room-temperature X-ray diffraction for the Fe-KTN and KTN single crystals. (c) The schematic diagrams of the vibration modes A1(2TO) and A1(3TO). (d) The Raman spectra of pristine KTN and Fe-KTN single crystals during the electric cycles. (e) The FWHM of the A1(3TO) mode for pristine KTN and Fe-KTN single crystals. (f) The peak position of the A1(3TO) mode for pristine KTN and Fe-KTN single crystals. | |
The lattice contraction inside Fe-KTN is characterized by XRD, as shown in Fig. 4(b). The angles of diffraction peaks (θ) in Fe-KTN are larger than those of KTN (Fig. S7, ESI†). The accurate lattice parameters of Fe-KTN and pristine KTN are also calculated in Table S2 (ESI†). Experimentally, the results of XRD show that the contraction of lattice induced by the shorter bond length after the generation of
happens, which demonstrate that the lattice parameters decrease after Fe doping. Meanwhile, the precise scanning of the (300) peak is also measured in Fig. 4(b). The intensity ratio of the lattice parameter c to (a + b) in Fe-KTN is less than 1/2 in the ideal case, indicating that the spontaneous polarization orientates towards the a or b direction, which is related to the increase of Eib inside Fe-KTN.
To prove that the lattice distortion after Fe doping is related to the improvement of the anti-fatigue performance, the Raman spectra during the electric cycles were also measured as shown in Fig. 4(d). Firstly, the weakening of the A1(2TO) peak of pristine KTN indicates the weakening of the reverse movement between the B-site ion and the oxygen octahedron, which leads to the huge reduction of polarization vectors. Moreover, the full width at half maximum (FWHM) and the peak position of the A1(3TO) mode are shown in Fig. 4(e and f). The FWHM of pristine KTN gradually widens from 50 cm−1 to 100 cm−1, and the A1(3TO) peak position shifts notably. This suggests that the initial tilting of oxygen octahedron can’t limit the migration of oxygen vacancies during the electric cycles, leading to the sharp shrinkage of the lattices inside KTN. As the
accumulates at the domain walls and pins the domain structures, the obvious disordered domain structures such as the tiny-periodic domains and cracks gradually appear inside the fatigued KTN. Finally, the pinning fatigued domain structures lead to a drastic drop in strain.
However, in Fe-KTN single crystals, the A1(2TO) vibration modes of Fe-KTN is almost unchanged because of the local structure
, yielding the stabilization of the polarization vectors (Fig. 1(d)). The FWHM of Fe-KTN has no significant changes, and the A1(3TO) peak position displays no obvious blue shift after cycles. The initial tilting of oxygen octahedron is maintained during the electric cycles because of the enhanced restriction of
by the local structure
. Therefore, the Fe-KTN single crystals exhibit a stable Eib value and the recoverability of macro-domain structures after the repeated cycles. At the same time, the strain is maintained due to the full response of the unpinned domain structures.
The strong local distributions of strain fields induced by the lattice distortion
The specific manifestation of limitation on
induced by
needs to be further revealed in the shrinking lattices. High-resolution transmission electron microscopy (HRTEM) is an effective method to study the internal effects of the contractile lattices. The microscopic atomic arrangement of Fe-KTN along the (010)c direction is shown in Fig. 5(a and c). The images of pristine KTN are given in Fig. S5 (ESI†). The diffraction patterns (Fig. S6, ESI†) suggest that both the Fe-KTN and the pristine KTN are in the tetragonal phase. Inside the Fe-KTN, the slant displacement of the B-site ions at the atomic scale is clearly observed, which is biased toward K+ along the red arrow in Fig. 5(c). However, for the pristine KTN, the B-site ions are almost located at the center of the four adjacent K+ ions without obvious displacements as shown in Fig. S5(c) (ESI†). In addition, the angle of three non-adjacent K+ ions inside Fe-KTN (∼88.052°) is less than that of KTN (∼88.836°). The results of atomic distribution directly demonstrate the contraction of the lattice after Fe3+ occupies the site of Nb5+/Ta5+.
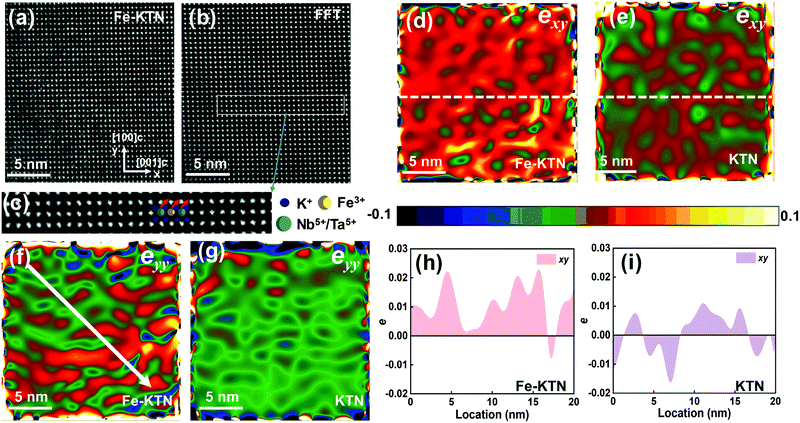 |
| Fig. 5 The bright-field HRTEM image of Fe-KTN (a) and the corresponding image of atomic distribution after FFT (b). (c) Part of the atomic distribution after zooming. (d–g) The local distribution of strain fields via geometric phase analysis (GPA), exy (d and e), eyy (f and g). (h and i) The accurate values of strain exy in (d) and (e) along the white centerline. | |
Moreover, the local distributions of strain fields were obtained based on geometric phase analysis (GPA) to accurately characterize the origin of
restriction in lattice distortion,45 as shown in Fig. 5(d–i). The strain tensor can be expressed as a symmetric matrix
| 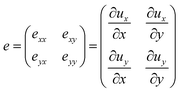 | (1) |
where
μx and
μy are the average of deformation along the
x and
y directions. The local distribution
exy of Fe-KTN is much larger than that of KTN as shown in
Fig. 5(d and e), which suggests that the Fe-KTN single crystal exhibits a larger shear strain induced by lattice contraction, thereby causing the strong restriction of

. Moreover, the stability of lattice vibration modes is also improved because of the enhanced limitation on

by the local structure

, yielding no significant changes of the FWHM and the peak position in the Raman spectra. The accurate values of strain
exy along the white centerline are shown in
Fig. 5(h and i). Obviously, the strain of Fe-KTN is more ordered than that of KTN. The unstable strain of KTN further illustrates the disorder of the domain structures of KTN. The strain
eyy of pristine KTN along the
y axis is relatively uniform as shown in
Fig. 5(g). However, the strain
eyy of Fe-KTN shows an increase along the diagonal direction as shown in
Fig. 5(f), again demonstrating the lattice distortion along the red arrow in
Fig. 5(c). The results of the HRTEM show that the restriction of

and the stability of the lattice vibration are enhanced
via the internal strain induced by the lattice distortion, which is fundamentally responsible for the improvement of anti-fatigue properties in Fe-KTN single crystals.
Conclusions
In conclusion, this work investigates the improvement of fatigue resistance in Fe-doped KTN (Fe-KTN) single crystals. Fe-doping gives rise to the promotion of electric-field-induced strain (by more than two times) and anti-fatigue properties (only an 11.5% drop after 105 cycles) compared with pristine KTN (a 69.3% drop). Meanwhile, an enhanced recoverability of Fe-doped domain structures in the process of fatigue was observed. Based on the improved domain-pinning models, the lattice distortion caused by the local structure
and the stability of the doped lattice vibration modes were also demonstrated. The enhanced restriction of
induced by the lattice contraction led to the stabilization of Eib and the recovery of the domain structures. Furthermore, the results of HRTEM directly revealed that the restriction of
and the recoverable lattice vibration were improved via the strong local strain fields induced by the lattice distortion, which was fundamentally responsible for the enhancement of fatigue resistance in Fe-KTN single crystals. The study revealing the origin of fatigue resistance provides an effective strategy to enhance the lattice distortion by ion doping to optimize the properties of ferroelectrics, which is also greatly significant in the practical application of doped lead-free ferroelectrics.
Conflicts of interest
There are no conflicts to declare.
Acknowledgements
This work was supported by the National Natural Science Foundation of China (Grants No. 12074092 and 12004085) and the Fundamental Research Funds for the Central Universities (XNGBQQ5750000319). P. T. acknowledges support through a fellowship of the China National Postdoctoral Program for Innovative Talents (Grant No. BX20200111), the Heilongjiang Postdoctoral Fund (Grant No. LBH-Z20065), and the Post-Doctoral Research Project at Harbin Institute of Technology.
References
- X. Liu and X. Tan, Adv. Mater., 2016, 28, 574–578 CrossRef CAS PubMed.
- B. Chen, T. Li, Q. Dong, E. Mosconi, J. Song, Z. Chen, Y. Deng, Y. Liu, S. Ducharme, A. Gruverman, F. De Angelis and J. Huang, Nat. Mater., 2018, 17, 1020–1026 CrossRef CAS PubMed.
- R. Yimnirun, R. Wongmaneerung, S. Wongsaenmai, A. Ngamjarurojana, S. Ananta and Y. Laosiritaworn, Appl. Phys. Lett., 2007, 90, 112908 CrossRef.
- M. I. Morozov and D. Damjanovic, J. Appl. Phys., 2010, 107, 034106 CrossRef.
- S. Zhang, X. Ru and T. R. Shrout, J. Electroceram., 2007, 19, 251–257 CrossRef CAS.
- F. Li, D. Lin, Z. Chen, Z. Cheng, J. Wang, C. Li, Z. Xu, Q. Huang, X. Liao, L.-Q. Chen, T. R. Shrout and S. Zhang, Nat. Mater., 2018, 17, 349–354 CrossRef CAS PubMed.
- F. Li, M. J. Cabral, B. Xu, Z. Cheng, E. C. Dickey, J. M. LeBeau, J. Wang, J. Luo, S. Taylor, W. Hackenberger, L. Bellaiche, Z. Xu, L.-Q. Chen, T. R. Shrout and S. Zhang, Science, 2019, 364, 264–268 CrossRef CAS PubMed.
- W. Liu, W. Chen, L. Yang, L. Zhang, Y. Wang, C. Zhou, S. Li and X. Ren, Appl. Phys. Lett., 2006, 89, 172908 CrossRef.
- S. Wada, K. Yako, H. Kakemoto, T. Tsurumi and T. Kiguchi, J. Appl. Phys., 2005, 98, 014109 CrossRef.
- M. Acosta, N. Novak, V. Rojas, S. Patel, R. Vaish, J. Koruza, G. A. Rossetti and J. Rodel, Appl. Phys. Rev., 2014, 4, 041305 Search PubMed.
- L.-Q. Cheng, K. Wang, J.-F. Li, Y. Liu and J. Li, J. Mater. Chem. C, 2014, 2, 9091–9098 RSC.
- P. Li, J. Zhai, B. Shen, S. Zhang, X. Li, F. Zhu and X. Zhang, Adv. Mater., 2018, 30, 1705171 CrossRef.
- C. Hu, X. Meng, M.-H. Zhang, H. Tian, J. E. Daniels, P. Tan, F. Huang, L. Li, K. Wang, J.-F. Li, Q. Lu, W. Cao and Z. Zhou, Sci. Adv., 2020, 6, 5979 CrossRef PubMed.
- S. Patel, A. Chauhan and R. Vaish, Appl. Mater. Today, 2015, 1, 37–44 CrossRef.
- S. M. Ke, H. T. Huang, H. Q. Fan, H. K. Lee, L. M. Zhou and Y.-W. Mai, Appl. Phys. Lett., 2012, 101, 082901 CrossRef.
- W. Liu, W. Chen, L. Yang, L. Zhang, Y. Wang, C. Zhou, S. Li and X. Ren, Appl. Phys. Lett., 2006, 89, 172908 CrossRef.
- T. Wang, D. Wang, Y. Liao, Q. Zheng, H. Sun, K. W. Kwok, N. Jiang, W. Jie, C. Xu and D. Lin, J. Eur. Ceram. Soc., 2018, 38, 4915–4921 CrossRef CAS.
- X. Ren, Nat. Mater., 2004, 3, 91–94 CrossRef CAS PubMed.
- T. Choi, S. Lee, Y. J. Choi, V. Kiryukhin and S.-W. Cheong, Science, 2009, 324, 63–66 CrossRef CAS PubMed.
- F. Di Mei, L. Falsi, M. Flammini, D. Pierangeli, P. Di Porto, A. J. Agranat and E. Delre, Nat. Photonics, 2018, 12, 734–738 CrossRef CAS.
- E. Delre, F. Di Mei, J. Parravicini, G. Parravicini, A. J. Agranat and C. Conti, Nat. Photonics, 2015, 9, 228–232 CrossRef CAS.
- P. Tan, H. Tian, F. Huang, X. Meng, Y. Wang, C. Hu, X. Cao, L. Li and Z. Zhou, Phys. Rev. Appl., 2019, 11, 024037 CrossRef CAS.
- Y. Wang, C. Hu, H. Tian, F. Huang, P. Tan, Y. Wu, C. Wang and Z. Zhou, J. Mater. Chem. C, 2020, 8, 3235–3239 RSC.
- Y. Wang, P. Tan, Z. Zhou, X. Huang, C. Hu, X. Meng, F. Huang, J. Wang and H. Tian, New J. Phys., 2021, 23, 013014 CrossRef CAS.
- P. Wu, Z. Zhao, X. Zhang and H. Liu, Opt. Express, 2018, 26, 8171–8178 CrossRef CAS PubMed.
- Y. Wang, P. Tan, X. Meng, Z. Zhou, X. Huang, C. Hu, F. Huang, J. Wang and H. Tian, IUCrJ, 2021, 8, 319–326 CrossRef CAS PubMed.
- F. Huang, C. Hu, H. Tian, X. Meng, P. Tan and Z. Zhou, Cryst. Growth Des., 2019, 19, 5362–5368 CrossRef CAS.
- F. Huang, C. Hu, H. Tian, X. Meng, P. Tan and Z. Zhou, CrystEngComm, 2019, 21, 7002–7010 RSC.
- F. Huang, C. Hu, Z. Zhou, X. Meng, P. Tan, Y. Wang, C. Wang and H. Tian, Acta Mater., 2020, 200, 24–34 CrossRef CAS.
- X. Cao, H. Tian, C. Hu, Y. Wang, X. Li, L. Li, X. Sun and Z. Zhou, J. Am. Ceram. Soc., 2018, 101, 3755–3760 CrossRef CAS.
- X. Cao, H. Tian, C. Hu, F. Huang, Y. Wang, X. Sun and Z. Zhou, J. Am. Ceram. Soc., 2019, 102, 3117–3122 CrossRef CAS.
- H. Tian, F. Huang, X. Meng, Y. Wang, Z. Xian, C. Hu and Z. Zhou, Appl. Phys. Lett., 2019, 115, 172901 CrossRef.
- X. Wang, B. Liu, Y. Yang, Y. Zhang and J. Wang, Mater. Res. Innovations, 2014, 18, 334–339 CrossRef CAS.
- H. Tian, P. Tan, X. Meng, C. Hu, B. Yao, G. Shi and Z. Zhou, J. Mater. Chem. C, 2015, 3, 10968–10973 RSC.
- J. Fu and R. Zuo, J. Appl. Phys., 2012, 112, 104114 CrossRef.
- B. Guiffard, E. Boucher, L. Eyraud, L. Lebrun and D. Guyomar, J. Eur. Ceram. Soc., 2005, 25, 2487–2490 CrossRef CAS.
- Y. Kim and J. Kim, Phys. Rev. B: Condens. Matter Mater. Phys., 1997, 55, 11933–11936 CrossRef.
- N. Wongdamnern, A. Ngamjarurojana, Y. Laosiritaworn, S. Ananta and R. Yimnirun, J. Appl. Phys., 2009, 105, 044109 CrossRef.
- N. Wongdamnern, N. Triamnak, M. Unruan, K. Kanchiang, A. Ngamjarurojana, S. Ananta, Y. Laosiritaworn and R. Yimnirun, Phys. Lett. A, 2010, 374, 391–395 CrossRef CAS.
- L. Zhang and X. Ren, Phys. Rev. B: Condens. Matter Mater. Phys., 2005, 71, 174108 CrossRef.
- Z. Cen, X. Wang, Y. Huan, Y. Zhen, W. Feng and L. Li, J. Am. Ceram. Soc., 2018, 101, 3032–3043 CrossRef CAS.
- D. Wang, J. Hlinka, A. A. Bokov, Z.-G. Ye, P. Ondrejkovic, J. Petzelt and L. Bellaiche, Nat. Commun., 2014, 5, 5100 CrossRef CAS PubMed.
- A. Bartasyte, J. Kreisel, W. Peng and M. Guilloux-Viry, Appl. Phys. Lett., 2010, 96, 262903 CrossRef.
- E. Bouziane, M. D. Fontana and M. Ayadi, J. Raman Spectrosc., 2005, 36, 872–878 CrossRef CAS.
- M. J. Hytch, E. Snoeck and R. Kilaas, Ultramicroscopy, 1998, 74, 131–146 CrossRef CAS.
- P. Li, X. Chen, F. Wang, B. Shen, J. Zhai, S. Zhang and Z. Zhou, ACS Appl. Mater. Interfaces, 2018, 10, 28772–28779 CrossRef CAS PubMed.
Footnote |
† Electronic supplementary information (ESI) available. See DOI: 10.1039/d1tc04480j |
|
This journal is © The Royal Society of Chemistry 2022 |
Click here to see how this site uses Cookies. View our privacy policy here.