Near-infrared photodetectors based on CH3NH3PbI3 perovskite single crystals for bioimaging applications†
Received
15th October 2021
, Accepted 19th November 2021
First published on 8th December 2021
Abstract
Near-infrared (NIR) photodetector based on CH3NH3PbI3 perovskite single crystal has potential application in NIR bioimaging in the first biological window (700–900 nm). To demonstrate NIR bioimaging with the CH3NH3PbI3 single crystal photodetector, the high-performance NIR photodetector based on the CH3NH3PbI3 single crystal with the excellent crystalline quality was fabricated, the microscopic imaging system was constructed, and the in vitro NIR bioimaging of the mouse organs was performed in this work. The NIR images of the mouse kidney, mouse spleen, and mouse ovary were consistent with the corresponding real objects, indicating the feasibility of the photodetector for NIR bioimaging. The results reported in this work show that the NIR photodetector based on the CH3NH3PbI3 single crystal is expected to be widely used in bioimaging.
1. Introduction
Bioimaging has important applications in the life sciences and medicine because it can provide detailed visualization of various biological samples. In contrast to conventional bioimaging in the visible region (400–700 nm), near-infrared (NIR) bioimaging in the first biological window (700–900 nm) offers numerous merits, including deeper penetration depth and lower photodamage.1–7 NIR photodetectors play a key role in NIR bioimaging. Thus, high performance NIR photodetectors in the range of 700–900 nm are highly desired for NIR bioimaging. At present, commercial NIR photodetectors for NIR bioimaging are based on inorganic semiconductor materials (e.g., Si, Ge, InGaAs). Nevertheless, the current commercial photodetectors have the disadvantages of high cost and complex production processes, which hamper their further applications in NIR bioimaging.8–11 Recently, NIR photodetectors based on colloidal quantum dots (CQD), 2D layered materials, and organic semiconductors have been widely studied,8,9,11–14 which have potential applications for NIR bioimaging. For example, Ren et al. has developed PbS–QD based on NIR photodetectors, which exhibit a responsivity (R) of 100–200 mA W−1 and specific detectivity (D*) of ∼1.0 × 1012 Jones in the 700–900 nm wavelength at 10 V.12 Li et al. has investigated NIR photodetectors based on Bi2O2Se 2D layered material.14 The R and D* can approach 1 A W−1 and ∼1.0 × 1011 Jones at 1 V and room temperature under 808 nm laser illumination. Huang et al. reported that NIR organic photodetectors based on CO1–4Cl achieved a high maximum R of ∼0.6 A W−1, an external quantum efficiency (EQE) of ∼50%, and D* of ∼1.0 × 1013 Jones in the 700–900 nm wavelength at 2 V.11 Despite the enormous progress achieved in NIR photodetectors based on the above materials, the performance and stability of these photodetectors need to be further improved to meet the practical requirement.8,14,15
Metal halide perovskites have sparked wide interest for optoelectronic devices due to their facile synthesis, excellent optical and optoelectronic properties.16–28 Among them, organic–inorganic perovskite CH3NH3PbI3 single crystals exhibit excellent stability in air, low trap-state density, high carrier mobility, large light absorption coefficient, long carrier diffusion length, and direct bandgap (∼1.48 eV), which are one of the most promising materials for NIR light detection in the first biological window.21–28 So NIR photodetector based on perovskite CH3NH3PbI3 single crystal has potential application in NIR bioimaging. However, to our knowledge, its application in bioimaging has not been reported so far. Based on this, this work will assess whether it is suitable for NIR bioimaging.
2. Experimental
2.1 Preparation of CH3NH3PbI3 single crystals
The CH3NH3PbI3 single crystals were prepared by inverse temperature crystallization.29 Briefly, an equimolar mixture of CH3NH3I and PbI2 was dissolved in γ-butyrolactone (GBL) at 60 °C to get a homogenous solution. The solution was filtered using a PTFE filter with 0.45 μm pore size. The filtrate was placed in a crystallizing dish and then was kept in an oil bath heated from 60 °C to 100 °C at the rate of 0.3 °C h−1. Black CH3NH3PbI3 single crystals with sizes of ∼1.5 cm were attained.
2.2 Preparation of the photodetector
To fabricate the metal–semiconductor–metal (MSM) structured photodetector based on CH3NH3PbI3 single crystal, interdigital Au electrodes were deposited upon the CH3NH3PbI3 single crystal via evaporation through a shadow mask, with the electrode gap of 200 μm, electrode length of 4.45 mm, and electrode width of 100 μm.
2.3 Biological sample acquisition
The female ICR mice were sacrificed for the experiments in 7–8 weeks. 75% alcohol was used to sterilize the mouse abdomen. The abdominal cavity was opened to remove the spleen that is located behind the stomach, kidneys at the lower abdomen, and the adipose wrapped ovaries. The spleen and kidneys were cleaned with normal saline and then fixed in 4% paraformaldehyde. The ovaries were also transferred into normal saline. Then, the adipose tissues enveloping the ovaries were isolated with a stereomicroscope. After washing, the ovaries were fixed in 4% paraformaldehyde. The fixed spleen, kidney, and ovary were used for imaging by microscope with our homemade photodetector.
2.4 Fabrication of the imaging system
The illumination and detection system of the commercial optical microscope was modified to make it suitable for NIR imaging. The lighting system was changed to an 800 nm NIR light source with the intensity of 0.1 mW cm−2 and equipped with a chopper. The detection system was changed to the CH3NH3PbI3 single crystal photodetector, and the photocurrent from the photodetector was collected by a lock-in amplifier and a computer. In this work, the samples to be tested were placed on the X–Y bidirectional scanning stage. The transmitted NIR light through samples was collected by a 10 times objective lens and then detected by the photodetector and converted into the photocurrent signal. The photocurrent values at different coordinate positions collected by the imaging system were presented in the form of an M × N matrix. The numerical matrix was converted into M × N gray image by the MATLAB program. Note that the voltage applied to the photodetector is 5 V during the following imaging process.
2.5 Measurements
The crystal structure of the CH3NH3PbI3 single crystal and its powder was characterized by a Japan Rigaku-D-Max Ra X-ray diffractometer (XRD). The absorption spectra were measured with a UV-3101PC spectrophotometer. The current–voltage (I–V) curves were measured using a computerized Keithley 2400 SourceMeter. The spectral responses and photocurrent–time (I–t) curves were measured using a lock-in amplifier, with a 150 W Xe lamp equipped with a monochromator as the light source. The response time of the photodetector was measured under the excitation of a 532 nm pulsed laser, and the transient signals were recorded by an oscilloscope.
3. Results and discussion
Fig. 1a shows the X-ray diffraction (XRD) patterns of CH3NH3PbI3 single crystal and its powder. For the CH3NH3PbI3 powder, all the XRD peaks could be indexed to the CH3NH3PbI3 crystal with a tetragonal structure. No additional phases from the starting material CH3NH3I, PbI2, or other impurities were found.23–26 To obtain the detailed crystal structure information, the Rietveld refinement was performed on the CH3NH3PbI3 powder XRD data, which is shown in Fig. S1 (ESI†). The unit cell parameters are determined a = b = 8.8857, c = 12.6791. From the CH3NH3PbI3 single crystal XRD patterns, it can be noted that there are only two sharp peaks corresponding to (200) and (400) crystal planes, which indicates that the CH3NH3PbI3 single crystal is preferably orientated along the (100) direction.21,25 The photograph of the CH3NH3PbI3 single crystal is shown in the inset of Fig. 1a. The single crystal exhibits a hexagonal shape, with the dimension of 15 mm × 14 mm × 4 mm. Its surface is very flat and uniform, which is suitable to be used as the photosensitive surface of photodetector. The absorption spectrum of the CH3NH3PbI3 single crystal is shown in Fig. 1b. The single crystal shows strong absorption in the wavelength region of 300–840 nm, and its absorption edge is located at 840 nm. It is obvious that CH3NH3PbI3 single crystal is suitable for NIR light detection in the first biological window. The steep absorption edge of the single crystal reveals excellent crystalline quality. The optical band gap of the single crystal was determined to be 1.48 eV from the Tauc plot shown in the inset of Fig. 1b, which is in good agreement with previous reports by other groups.21,30 To study the trap density (ntrap) in the CH3NH3PbI3 single crystal, a hole-only device was constructed by depositing Au electrodes on the upper and lower surfaces of the single crystal. The current–voltage (I–V) characteristic of the device in the dark was investigated by the space charge limited current method,21,23,25,26,31 which is shown in Fig. 1c. It can be noted the I–V curve exhibits the linear ohmic relationship when the voltage is lower than 2.2 V. When the voltage is higher than 2.2 V and lower than 18.3 V, the current increases sharply with the voltage, indicating that all trap states in the CH3NH3PbI3 single crystal are occupied by the injected charge carriers. So, in our case, the trap-filled limit voltage (VTFL) is about 2.2 V. Based on the value of VTFL, the ntrap can be calculated with the following formula:21,23,25,26,31 | 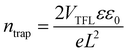 | (1) |
where ε0 is the vacuum permittivity, ε is the relative dielectric constant of the CH3NH3PbI3 single crystal (ε = 32),32 L is the thickness of the single crystal, and e is the electron charge. The ntrap can be deduced to be as low as 8.7 × 108 cm−3. The value of ntrap can be comparable to that of the reported high quality CH3NH3PbI3 single crystal. For example, Shi et al. reported the ntrap of 3.3 × 1010 cm−3,23 Zhang et al. reported the ntrap of 2.1 × 109 cm−3,25 Ye et al. reported the ntrap of 1.14 × 109 cm−3,26 Lian et al. reported the ntrap of (1.80 ± 1.07) × 109 cm−3,21 and Liu et al. reported the ntrap of 6.0 × 108 cm−3.31 So, it indicates that the CH3NH3PbI3 single crystal in our case exhibits excellent crystalline quality with very low density of trap states, which is very important for the realization of high-performance NIR photodetectors.
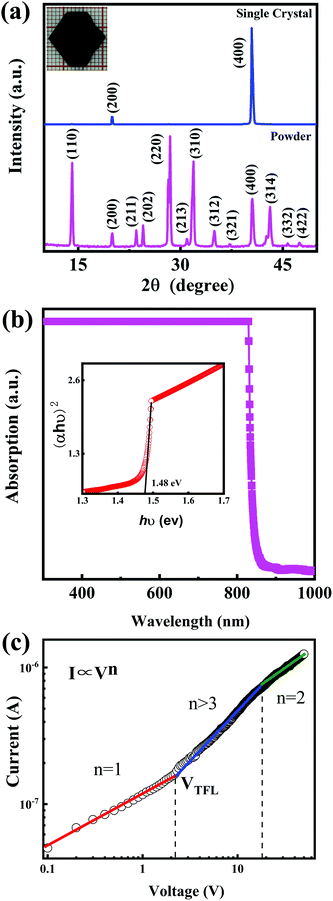 |
| Fig. 1 (a) X-ray diffraction patterns of CH3NH3PbI3 single crystal and its powder. Inset: photograph of the CH3NH3PbI3 single crystal. (b) Absorption spectrum of CH3NH3PbI3 single crystal, with the inset showing the corresponding Tauc plot. (c) Current–voltage (I–V) characteristic of CH3NH3PbI3 single crystal in the dark investigated by the space charge limited current method. | |
To explore the photoelectric properties of the CH3NH3PbI3 single crystal, the planar structured photodetector was fabricated by depositing the interdigitated Au electrodes on the CH3NH3PbI3 single crystal, as schematically illustrated in the inset of Fig. 2a. The Au fingers of the interdigital electrode are 4.45 mm in length, 100 μm in width, and the inter-electrode spacing is 200 μm. The I–V curve was measured under the dark, as shown in Fig. 2a. It can be noted that the dark current is as low as 9.4 × 10−8 A at 5 V. The low dark current is due to the excellent crystalline quality of the single crystal, which is beneficial to realize high detectivity of the photodetector. The photoresponse spectrum of the photodetector based on the CH3NH3PbI3 single crystal at 5 V is shown in Fig. 2b. It can be noted that the device exhibits the maximum responsivity of 1.33 A W−1 in the NIR wavelength of 780 nm. The cutoff wavelength of the photoresponse is located at around 840 nm, which is consistent with the absorption spectrum of the CH3NH3PbI3 single crystal. So, the photodetector based on the CH3NH3PbI3 single crystal in our case is very suitable for NIR light detection in the first biological window. The external quantum efficiency (EQE) of the device can be determined by the following expression:32,33
| 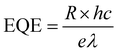 | (2) |
where
R is the responsivity of the device,
h is the Planck's constant,
c is the velocity of light,
e is the elementary charge of the electron, and
λ is the wavelength of the incident light. At 5 V, the maximum EQE of the device is 211.29% at 780 nm, as shown in
Fig. 2c. The detectivity (
D*) of the device can be expressed by the following formula:
34,35 | 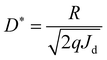 | (3) |
where
q is the elemental charge, and
Jd is the dark current density. At 5 V, the maximum
D* of the device is 2.18 × 10
12 Jones at 780 nm, which is shown in
Fig. 2d. The stability of the photodetector is very important for the accuracy and clarity of bioimaging. To study the stability of the device, the photocurrent of the device as a function of time has been measured with the illumination of 800 nm light on and off repeatedly for 10 cycles at 5 V, which is shown in
Fig. 2e. The device exhibited excellent repetition of photocurrent under the light on/off conditions, and almost no decay of the photocurrent during the measurement. It indicates the good short-term stability of the device. However, CH
3NH
3PbI
3 is easy to break down in the presence of moisture and oxygen.
16 Therefore, to achieve long-term stability of the CH
3NH
3PbI
3 single crystal photodetector, the photodetector should be vacuum sealed to isolate moisture and oxygen. To characterize the response speed of the device, the transient photocurrent response at 5 V was measured. The light source was a 532 nm pulsed laser. The rise and decay time, defined as the time for photocurrent changing from 10% to 90% of its maximum and
vice versa, were evaluated to be 125 μs and 1.93 ms, respectively, as shown in
Fig. 2f. To evaluate the performance of the device in our case, we compared the critical parameters of the device with the reported high performance NIR photodetector in the NIR wavelength of 800 nm, as shown in
Table 1. Obviously, the device is a high performance NIR photodetector in the first biological window, indicating its potential application for bioimaging. Note that the performance of the device is limited by the large electrode spacing (200 μm). If the electrode spacing can be reduced, the performance of the device will be further improved.
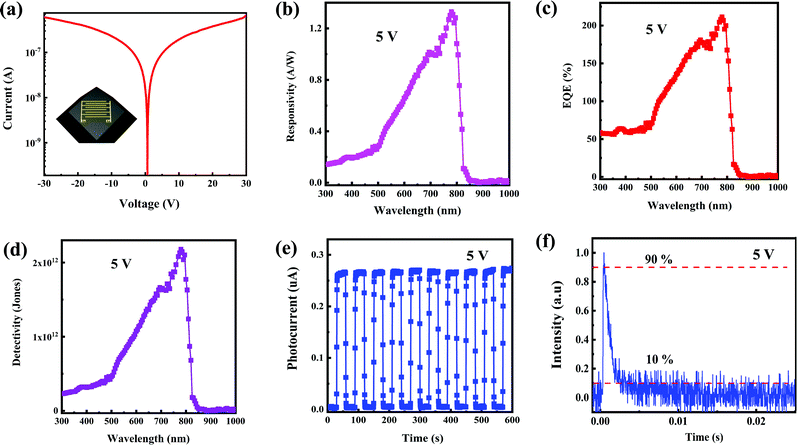 |
| Fig. 2 (a) I–V curve of the photodetector based on the CH3NH3PbI3 single crystal measured under the dark, with the inset showing a schematic diagram of the device structure. (b) Photoresponse spectrum of the photodetector based on the CH3NH3PbI3 single crystal at 5 V. (c) External quantum efficiency of the device at 5 V. (d) Detectivity of the device at 5 V. (e) Photocurrent of the device as a function of time measured with the illumination of 800 nm light on and off repeatedly for 10 cycles at 5 V. (f) The response time of the device at 5 V. The light source was a 532 nm pulsed laser. | |
Table 1 Comparison of the critical parameters for the NIR photodetectors in the 800 nm wavelength
Photoactive material |
R (A W−1) |
EQE (%) |
D* (Jones) |
Rise/decay time |
Voltage (V) |
Ref. |
PbS QDs |
∼0.15 |
NA |
∼1.0 × 1012 |
∼3.63 ms/∼29.56 ms |
10 |
12
|
Bi2O2Se 2D layered material |
∼1 |
NA |
∼1.0 × 1011 |
∼3 ms/∼4.5 ms |
1 |
14
|
CO1–4Cl organics |
∼0.6 |
∼50 |
∼1.0 × 1013 |
NA/NA |
−2 |
11
|
(FASnI3)0.6(MAPbI3)0.4 film |
∼0.4 |
∼65 |
∼1.0 × 1012 |
∼6.9 μs/∼9.1 μs |
−0.2 |
35
|
CH3NH3PbI3 film |
∼0.06 |
∼40 |
1.27 × 1012 |
12.7 μs/6.9 μs |
0 |
7
|
CH3NH3PbI3 single crystals |
∼0.24 |
∼36.16 |
NA |
∼71 μs/∼112 μs |
0 |
27
|
CH3NH3PbI3 single crystals |
1.08 |
167.64 |
1.77 × 1012 |
∼125 μs/∼1.93 ms |
5 |
This work |
To apply the NIR photodetector based the CH3NH3PbI3 perovskite single crystal to in vitro bioimaging, an appropriate imaging system should be constructed. An optical microscope is an important means of in vitro bioimaging, so the imaging system in our case was constructed based on the optical microscope. The schematic diagram of the microscopic imaging system is shown in Fig. 3a, and the light path diagram is shown in Fig. 3b.
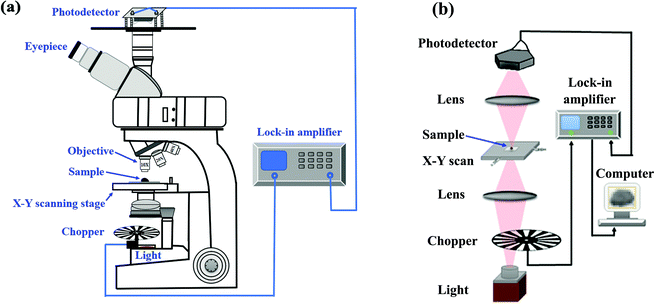 |
| Fig. 3 (a) Schematic diagram of the microscopic imaging system. (b) Light path diagram of the microscopic imaging system. | |
To investigate the imaging capability of the CH3NH3PbI3 single crystal photodetector, the letter T model with a regular boundary made by a 3D printer was firstly imaged by the microscopic imaging system. For the model shown in Fig. 4a, the T-shaped area is hollowed, and the background area is opaque. The NIR image of the T model is shown in Fig. 4b, and the curve of gray values in X and Y directions changing with the position in the T model image is shown in Fig. 4c. It can be seen that the transverse length of the T model image is about 23 mm, and the longitudinal length is about 3 mm, which is consistent with the real object. It demonstrated the feasibility of the photodetector for NIR imaging. Next, the in vitro NIR bioimaging of the mouse organs was performed. The physical pictures of the mouse kidney and mouse spleen taken by the SLR camera are shown in Fig. 4d and g, respectively. It can be observed that the mouse kidney is a reddish brown organ shaped like a broad bean, which is about 11 mm in length and 7 mm in width, and the mouse spleen is a reddish brown, oval, flat and curved organ, which is about 15 mm in length and 5 mm in width. Fig. 4e and h are the NIR images of the mouse kidney and spleen with a pixel size of 18 × 28 and 14 × 29 acquired by the microscopic imaging system, and the corresponding curves of gray values in X and Y directions changing with the position in NIR images are shown in Fig. 4f and i, respectively. Comparing the physical pictures and the NIR images of the kidney and spleen, it can be noted that the shape of the two images is the same as that of the corresponding objects, indicating the feasibility of the photodetector for in vitro NIR bioimaging. It can also be observed that the edge gray of the sample images is slightly stronger than the internal gray. This is because the NIR light can pass through the thin edge of the kidney and spleen and reach the photodetector, causing an increase in the photocurrent of the photodetector. The photocurrent of the photodetector is directly proportional to the gray value of the images in our case. So, the gray value at the edges of the images is increased. It indicates that the photodetector has high sensitivity. After bioimaging of the large organs of the mouse (spleen and kidney), the bioimaging of a small organ of the mouse was carried out. Fig. 4j shows the physical picture of the mouse ovary taken by CCD under a stereomicroscope. It can be seen that the mouse ovary shows a small size of ∼2 mm. Fig. 4k shows the NIR image of the mouse ovary with a pixel size of 12 × 20 obtained by the photodetector, and the curve of gray values in X and Y directions changing with the position in the NIR image is shown in Fig. 4l. It can be noted that the image can accurately show the shape characteristics of the ovary. For example, the concave position of the lower edge in the physical picture of the ovary can be clearly observed in the corresponding NIR image. The above results show that the CH3NH3PbI3 single crystal photodetector in our case can realize high quality NIR bioimaging. The imaging speed and clarity of the present imaging system need to be further improved. Multi-pixel sensor array, that is, multiple optical sensors built on a single crystal, can improve the clarity and speed of the imaging system. The information at different positions of the sample will be collected by different optical sensors at the same time without X–Y scanning, which will improve the imaging speed and imaging clarity. The above work will be realized in our future work.
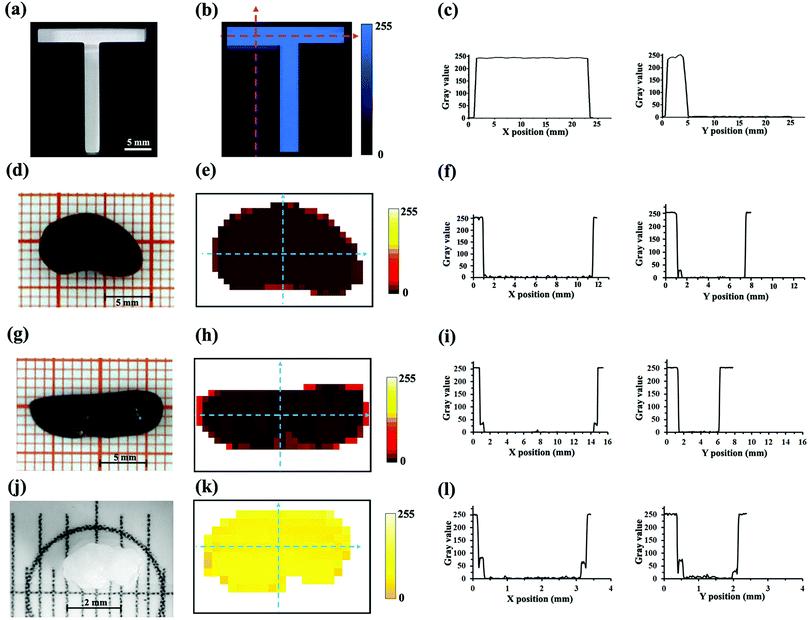 |
| Fig. 4 (a) Picture of the letter T taken by SLR camera. (b) NIR image of the T model. (c) Curve of gray values in X and Y directions changing with position in the T model image. Physical pictures of the mouse kidney (d) and mouse spleen (g) taken by SLR camera. (j) Physical picture of the mouse ovary taken by CCD under a stereomicroscope. NIR images of the mouse kidney (e), spleen (h), and ovary (k) acquired by the microscopic imaging system. Curves of gray values in X and Y directions changing with position in NIR images of the mouse kidney (f), spleen (i), and ovary (l). | |
4. Conclusions
In conclusion, CH3NH3PbI3 single crystals were prepared by inverse temperature crystallization and showed strong absorption in the wavelength region of 300–840 nm with the absorption edge of 840 nm. The single crystal exhibited excellent crystalline quality with a very low density of trap states of 8.7 × 108 cm−3. The planar structured photodetector based on the single crystal was fabricated. At 5 V, the device exhibited the best performance in the NIR wavelength of 780 nm, with the responsivity of 1.33 A W−1, external quantum efficiency of 211.29%, detectivity of 2.18 × 1012 Jones, and excellent stability. In vitro NIR bioimaging of the mouse organs was performed by the microscopic imaging system equipped with the CH3NH3PbI3 single crystal photodetector. The NIR images of the mouse kidney, mouse spleen, and mouse ovary were well consistent with the corresponding real objects, indicating the feasibility of the photodetector for NIR bioimaging. The results reported in this work are expected to promote the application of CH3NH3PbI3 perovskite single crystal NIR photodetector for bioimaging.
Author contributions
Ji Yu: investigation, data curation, writing – original draft. Jie Zheng: investigation, data curation. Hongyu Chen: formal Analysis, writing – reviewing and editing. Ning Tian: investigation, writing – reviewing and editing. Lin Li: validation, writing – reviewing and editing. Yanmei Qu: investigation, data curation. Yongtao Huang: investigation, data curation. Yinxian Luo: investigation, data curation. Wenzhu Tan: investigation, data curation.
Conflicts of interest
There are no conflicts to declare.
Acknowledgements
This work is supported by the National Natural Science Foundation of China (Grant No. 12074095, 11804235, 11704264), the Support Plan for Young and Middle-Aged Scientific and Technological Innovation Talents of Shenyang City (Grant No.RC200213), the Natural Science Foundation of Heilongjiang Province (Grant No. LH2019A016) and the 2020 Central Government's plan to support the Talent Training Project of the Reform and Development Fund of Local Universities (Grant No. 2020YQ02).
References
- R. Weissleder, Nat. Biotechnol., 2001, 19(4), 316–317 CrossRef CAS PubMed.
- C. Li, H. Wang, F. Wang, T. Li, M. Xu, H. Wang, Z. Wang, X. Zhan, W. Hu and L. Shen, Light: Sci. Appl., 2020, 9(1), 31 CrossRef CAS PubMed.
- J. Huang and K. Pu, Chem. Sci., 2021, 12(10), 3379–3392 RSC.
- C. Shen, Q. Lou, K. Liu, L. Dong and C. Shan, Nano Today, 2020, 35, 100954 CrossRef CAS.
- H. Sun, J. Yang, M. Fuji, Y. Sakka, Y. Zhu, T. Asahara, N. Shirahata, Z. Bai, J. Li and H. Gao, Small, 2011, 7(2), 199–203 CrossRef CAS PubMed.
- H. Chen, H. Liu, Z. Zhang, K. Hu and X. Fang, Adv. Mater., 2016, 28(3), 403–433 CrossRef CAS PubMed.
- J. Wang, S. Xiao, W. Qian, K. Zhang, J. Yu, X. Xu, G. Wang, S. Zheng and S. Yang, Adv. Mater., 2021, 33(3), 2005557 CrossRef CAS PubMed.
- X. Liu, Y. Lin, Y. Liao, J. Wu and Y. Zheng, J. Mater. Chem. C, 2018, 6(14), 3499–3513 RSC.
- K. Xu, X. Xiao, W. Zhou, X. Jiang, Q. Wei, H. Chen, Z. Deng, H. Huang, B. Chen and Z. Ning, ACS Appl. Mater. Interfaces, 2020, 12(13), 15414–15421 CrossRef CAS PubMed.
- Z. Wu, W. Yao, A. London, J. Azoulay and T. Ng, Adv. Funct. Mater., 2018, 28(18), 1800391 CrossRef.
- J. Huang, J. Lee, J. Vollbrecht, V. Brus, A. Dixon, D. Cao, Z. Zhu, Z. Du, H. Wang, K. Cho, G. Bazan and T. Nguyen, Adv. Mater., 2020, 32(1), 1906027 CrossRef CAS PubMed.
- Z. Ren, J. Sun, H. Li, P. Mao, Y. Wei, X. Zhong, J. Hu, S. Yang and J. Wang, Adv. Mater., 2017, 29(33), 1702055 CrossRef PubMed.
- D. Wu, C. Jia, F. Shi, L. Zeng, P. Lin, L. Dong, Z. Shi, Y. Tian, X. Li and J. Jie, J. Mater. Chem. A, 2020, 8(7), 3632–3642 RSC.
- J. Li, Z. Wang, Y. Wen, J. Chu, L. Yin, R. Cheng, L. Lei, P. He, C. Jiang, L. Feng and J. He, Adv. Funct. Mater., 2018, 28(10), 1706437 CrossRef.
- C. Bi, S. Kershaw, A. Rogach and J. Tian, Adv. Funct. Mater., 2019, 29(29), 1902446 CrossRef.
- H. Wang, S. Li, X. Liu, Z. Shi, X. Fang and J. He, Adv. Mater., 2020, 33(7), 2003309 CrossRef PubMed.
- Z. Li, Z. Li, Z. Shi and X. Fang, Adv. Funct.
Mater., 2020, 30(28), 2002634 CrossRef CAS.
- Z. Li, X. Liu, C. Zuo, W. Yang and X. Fang, Adv. Mater., 2021, 33(41), 2103010 CrossRef CAS PubMed.
- Y. Li, Z. Shi, X. Li and C. Shan, Chin. Phys. B, 2019, 28(1), 017803 CrossRef CAS.
- F. Wang, X. Zou, M. Xu, H. Wang, H. Wang, H. Guo, J. Guo, P. Wang, M. Peng, Z. Wang, Y. Wang, J. Miao, F. Chen, J. Wang, X. Chen, A. Pan, C. Shan, L. Liao and W. Hu, Adv. Sci., 2021, 8(14), 2100569 CrossRef CAS PubMed.
- Z. Lian, Q. Yan, Q. Lv, Y. Wang, L. Liu, L. Zhang, S. Pan, Q. Li, L. Wang and J. Sun, Sci. Rep., 2015, 5, 16563 CrossRef PubMed.
- Z. Lian, Q. Yan, T. Gao, J. Ding, Q. Lv, C. Ning, Q. Li and J. Sun, J. Am. Chem. Soc., 2016, 138(30), 9409–9412 CrossRef CAS PubMed.
- D. Shi, V. Adinolfi, R. Comin, M. Yuan, E. Alarousu, A. Buin, Y. Chen, S. Hoogland, A. Rothenberger, K. Katsiev, Y. Losovyj, X. Zhang, P. Dowben, O. Mohammed, E. Sargent and O. Bakr, Science, 2015, 347(6221), 519–522 CrossRef CAS PubMed.
- Y. Zhang, Y. Liu, Z. Yang, S. Liu and S. Liu, J. Energy Chem., 2018, 27(3), 722–727 CrossRef.
- X. Zhang, X. Dong, S. Wang, H. Liu, W. Hu and X. Li, Chem. Eng. J., 2021, 404, 125957 CrossRef CAS.
- F. Ye, H. Lin, H. Wu, L. Zhu, Z. Huang, D. Ouyang, G. Niu and W. Choy, Adv. Funct. Mater., 2019, 29(6), 1806984 CrossRef.
- J. Ding, H. Fang, Z. Lian, J. Li, Q. Lv, L. Wang, J. Sun and Q. Yan, CrystEngComm, 2016, 18(23), 4405–4411 RSC.
- Q. Lin, A. Armin, P. Burn and P. Meredith, Laser Photonics Rev., 2016, 10(6), 1047–1053 CrossRef CAS.
- M. Saidaminov, A. Abdelhady, B. Murali, E. Alarousu, V. Burlakov, W. Peng, I. Dursun, L. Wang, Y. He, G. Maculan, A. Goriely, T. Wu, O. Mohammed and O. Bakr, Nat. Commun., 2015, 6, 7586 CrossRef PubMed.
- Y. Dang, Y. Liu, Y. Sun, D. Yuan, X. Liu, W. Lu, G. Liu, H. Xia and X. Tao, CrystEngComm, 2015, 17(3), 665–670 RSC.
- Y. Liu, Y. Zhang, Z. Yang, D. Yang, X. Ren, L. Pang and S. Liu, Adv. Mater., 2016, 28(41), 9204–9209 CrossRef CAS PubMed.
- Z. Cheng, K. Liu, J. Yang, X. Chen, X. Xie, B. Li, Z. Zhang, L. Liu, C. Shan and D. Shen, ACS Appl. Mater. Interfaces, 2019, 11(37), 34144–34150 CrossRef CAS PubMed.
- L. Li, H. Chen, Z. Fang, X. Meng, C. Zuo, M. Lv, Y. Tian, Y. Fang, Z. Xiao, C. Shan, Z. Xiao, Z. Jin, G. Shen, L. Shen and L. Ding, Adv. Mater., 2020, 32(24), 1907257 CrossRef CAS PubMed.
- J. Yu and N. Tian, Phys. Chem. Chem. Phys., 2016, 18(34), 24129–24133 RSC.
- W. Wang, D. Zhao, F. Zhang, L. Li, M. Du, C. Wang, Y. Yu, Q. Huang, M. Zhang, L. Li, J. Miao, Z. Lou, G. Shen, Y. Fang and Y. Yan, Adv. Funct. Mater., 2017, 27(42), 1703953 CrossRef.
Footnote |
† Electronic supplementary information (ESI) available: Fig. S1. XRD Rietveld refinement result of the CH3NH3PbI3 powder. See DOI: 10.1039/d1tc04961e |
|
This journal is © The Royal Society of Chemistry 2022 |