India's biomethane generation potential from wastes and the corresponding greenhouse gas emissions abatement possibilities under three end use scenarios: electricity generation, cooking, and road transport applications†
Received
25th July 2022
, Accepted 8th November 2022
First published on 8th November 2022
Abstract
This paper evaluates India's annual waste-to-energy potential through biomethane production, and the corresponding greenhouse gas abatement. Biodegradable wastes generated across various sectors (agriculture, horticulture, animal husbandry, municipalities, sericulture, fisheries, and industries) are examined, many of which have not been considered previously for India's bioenergy potential assessments. The degree of replaceability of present-day unclean fuels and the net avoided greenhouse gas emissions from the utilisation of this biomethane are evaluated for three separate end use scenarios: electricity generation, cooking, and road transportation. The total biomethane generation potential is 125 billion cubic metres, after considering a gas leakage rate of 3%. The corresponding total heat and electrical energy generation potentials are 4.49 EJ and 748.59 TWh respectively; the breakdown of this for all the states and union territories of India is also calculated. Biomethane from wastes could have provided for either 47% of India's gross generated electricity or 91% of India's road transport fuel demand in the financial year of 2018–19. Less than 43% of this biomethane could supply the entirety of the country's cooking fuel demand. The corresponding avoided GHG emissions from the displacement of fossil fuels and the prevention of crop residue field burning and municipal waste dumping are between 284 and 461 million tonnes of carbon dioxide-equivalent, excluding the contribution from black carbon. The avoided particulate emissions from crop residue burning prevention is around 2 million tonnes. Thus, this paper makes a strong case for biomethane generation from wastes in India to appropriately address climate change impact, pollution, and waste disposal problems, and aims to inform and influence energy policy in the country, with additional considerations of the gap between the potential and the state-of-the-art, and the technical and socio-economic challenges of waste-to-energy schemes. In addition to the quantitative evaluations, this paper contains a comprehensive compilation of data on waste and biomethane generation potentials from experiments and surveys scattered across the literature; it is hoped that this will be a valuable resource for future research, energy assessments, and policy considerations.
1 Introduction
India is the world's seventh largest and second most populous country, with a population of 1.38 billion (in 2020).1 The size and huge population give rise to several challenges. The first of these is that there is a high demand for energy: with one of the most rapid rates of development in the world,2 India consumed 1210 TWh of electricity in the financial year of 2018–19,3 and the demand for crude oil is so high that India imports nearly 80%4 of the quantity consumed. Secondly, there is an asymmetry in energy access: though the country is officially considered to have 100% electricity access after an excellent countrywide electrification drive by the government recently, almost 300 million people either still do not have electricity access or are unhappy with their electricity supply.5–8 This is because a village is considered electrified if all of its public places and 10% of its houses have access to electricity;5–8 also, some remote villages got left out of the electrification drive.5 Other prominent challenges are those of air pollution and waste management, chiefly agricultural residues and municipal solid wastes (MSW). With a countrywide MSW generation rate of nearly 163 million tonnes per day (in 2018–19),9 which increases at a mean rate of 5% per annum,10 urban waste management is considered a problem by many municipal authorities.11
These challenges in turn lead to significant environmental problems; India is not only a major contributor to global climate change and air pollution but is also drastically impacted by them. India is the world's third largest emitter of greenhouse gases (GHGs),12 but the per-capita emissions are low compared to the global average,13 denoting again, an asymmetry in energy use and access. Existing energy sources are carbon-intensive and polluting: around 72% electricity is generated from coal.14 Current waste management practices are poor: about 72–90% MSW is left in open dumps with little or no processing,11,15 causing air, soil, and water pollution, methane emissions, and a spreading of foul odour and pathogens. The MSW collection efficiency is not 100% (though there has been considerable improvement since 2010, as detailed in previous studies.11,15). Additionally, due to a lack of source segregation, biodegradables and plastics end up in the same garbage dumps almost throughout the country.16–18 Pollution from the field burning of crop residues (FBCR) causes largescale pollution, health, and visibility problems, disrupting the life and work of citizens, with considerable associated economic losses, especially in North India and around the National Capital Region.19–24 This FBCR also causes a loss of nutrients,23,25 kills beneficial soil microorganisms, and has adverse impacts on the soil nitrogen balance, leading to a gradual reduction in soil fertility.23 However, burning is an easy and traditional practice for quick crop residue and orchard pruning waste management. Some of these are also burnt as solid biomass fuels (SBFs) for cooking (and a small part, as insect repellent), causing GHG and particulate matter (PM) emissions.26
There is an opportunity to exploit municipal, agricultural, horticultural, and industrial wastes for energy generation, as noted in some previous studies.27–30 This could form part of the energy transition to low-carbon, non-polluting, and locally available energy sources to improve energy access, address climate change, and make India more self-reliant in energy supply. It would also address waste management difficulties. In order to enable such an energy transition, it is important to identify all available renewable and sustainable energy sources along with their geographical locations in the country. This will help in planning energy system expansions and the replacement of fossil fuels with cleaner energy sources.
In this paper, we assess the state-wise energy generation potential of organic wastes produced annually in India from all major sectors, i.e., agriculture, horticulture, animal husbandry, industries (including sericulture and fisheries), and municipalities, through anaerobic digestion (decomposition by microorganisms in the absence of oxygen) to produce biogas. Our assessment is based on experimental results on biomethane generation from the literature and also includes a much more comprehensive list of wastes than previous studies; so, this study should estimate India's waste-to-energy (WTE) potential more accurately than previous publications that are usually based on assumed biogas yields for most substances. We also evaluate the degree of replaceability of existing unclean fuels in India with biogas, under three end-use scenarios, i.e., electricity generation, cooking, and road transportation, and the corresponding annual GHG emissions abatement potential, which add to the novelty of this study. The emissions avoided from the prevention of FBCR and MSW open dumping are also estimated. As part of the assessment, a comprehensive compilation of experimental results on characteristics and biomethane generation of many types of wastes was prepared, from the academic literature. This is included here to simplify future bioenergy assessments in India and other countries. Being a spatial (state-wise) energy potential assessment, it is hoped that this paper will inform policy framing by encouraging India's central and state governments towards greater WTE conversion, and also inspire similar energy research and transitions in other developing countries.
Biogas was chosen as the preferred energy generation route from wastes because it is the most efficient path for WTE conversion (even when compared with bioethanol),31 and several life cycle assessment (LCA) studies conclude that biogas generation and composting are the most effective and environmentally sustainable strategies for organic waste management.32–34 Biogas (mostly methane) is produced by anaerobic digestion (AD) (alongside digestate fertiliser) and undergoes clean combustion to emit only carbon dioxide (CO2) and water vapour. This emitted CO2 is biogenic, i.e., it is part of the Earth's natural carbon cycle, and would be emitted during the usual aerobic (oxidative) decay of organic matter anyway, even on not producing and utilising biogas; thus, biogas burning does not add any additional carbon to the atmosphere. It is also worth noting that methane, which is a much more potent GHG, is only released during anaerobic decay of organic matter and not natural aerobic decay (some methane is released from large dumps due to anaerobic digestion in the deep layers because of a shortage of atmospheric oxygen supply; these would also be avoided on producing biogas). Biogas can thus displace fossil fuels and reduce the carbon impact of energy use. Energy extraction from wastes has lower environmental impact compared to other biomass energy sources, like growing dedicated energy crops35 because wastes are ubiquitous and are generated irrespective of their use, often causing management problems. The environmental impacts of biogas generation are negligible,36 even when the positive impacts of displacing fossil fuels and chemical fertilisers are not explicitly considered. Biogas is a renewable, sustainable, and ozone-layer-friendly energy source.37 Additionally, the digestate that remains is a rich organic fertiliser and performs as good as synthetic fertilisers.38 It can displace synthetic fertilisers in agricultural fields, potentially offsetting further, the environmental impacts of their manufacture.
2 Methods and data sources
2.1. Biomethane generation potential assessment
Biodegradable wastes generated from various sectors in India were identified as follows:
• Crop residues from agricultural and horticultural activities, including orchard pruning wastes.
• Wastes from the animal husbandry sector.
• Municipal solid wastes (MSW) from urban and semi-urban areas.
• Wastes from fisheries.
• Wastes from sericulture (silk production).
• Municipal wastewaters.
• Industrial wastewaters and solid (organic) wastes.
A wide array of sources in the literature relating to bioenergy in general, and these specific sectors in particular, were reviewed to compile data and methodologies for WTE potential calculations through anaerobic digestion.
Biogas is an energy-rich gaseous mixture consisting of methane (usually 50–75% by volume), carbon dioxide, and trace amounts of other gases like oxygen, nitrogen, hydrogen sulphide, and ammonia.39,40 Biogas is usually upgraded to the methane purity of natural gas; the trace gases, especially hydrogen sulphide and ammonia, are removed before use.41,42 Methane is the only combustible component in biogas; we refer to this as ‘biomethane’ henceforth, and obtain its volume directly, rather than that of biogas. This keeps calculations independent of the varying methane concentrations in biogas obtained from different substances.
In this paper, biomethane generation calculations are based on the volatile solids (VS) content of substances, defined as the amount of organic matter present in the material that biodegrades during anaerobic digestion to generate biogas.43,44 It may be mentioned as a percentage/fraction of the mass of the substance or its total solids (TS) concentration. The TS (total solids) or dry matter content of a sample is the net amount of solid material present in it; this remains after the moisture contained in the substance is completely removed, and is expressed as a percentage/fraction of the mass of the substance under consideration.43–45 If VSTS is the VS content of a material expressed as a fraction of its TS, then the net volatile solids content (VS) is given by eqn (1).43,46
The volume of biomethane (V) generated from a substance is calculated using one of the eqn (2a)–(2c), depending on whether methane generation was available per unit mass of VS, TS, or fresh matter respectively (after Langeveld et al.43):
Here,
W = mass of available fresh (wet) wastes undergoing anaerobic digestion; VS = volatile solids content as a fraction of the mass of wastes, either available directly, or calculated using
eqn (1) if available in terms of TS. TS = total solids content as a fraction of the mass of wastes.
Yb,VS = biochemical methane potential (BMP) per unit mass of VS,
i.e., the specific cumulative biomethane yield per unit mass of VS over the entire period allowed for anaerobic digestion (called the hydraulic retention time, HRT
47);
Yb,TS = corresponding BMP per unit mass of TS;
Yb,FM = corresponding BMP per unit mass of the wastes or fresh matter (FM).
In this paper, the values of TS and VS were obtained from the academic literature. The TS values reported with respect to fresh/wet wastes (and not dry wastes) were used since production quantities were evaluated for wastes that are directly available; the masses will reduce on drying these, depending upon the moisture lost; moisture losses are not directly relevant to our study. The Yb's (BMPs in one of the forms) required in the above equations were sourced from published experimental results on waste mono-digestion, to account for the different methane yields of different substances and obtain dependable predictive quantitative estimates. Mono-digestion was considered even though co-digestion can slightly improve yields for some waste streams (like crop and animal wastes43,48–50) because the simultaneous availability of wastes from different sources, and hence, the possibilities and extents of mixing, can vary spatially and temporally. The mixing ratios cannot be obtained for the whole country in this work and need to be determined for individual biogas plants depending on location, on a case-by-case basis. This makes our estimates slightly modest since multiple wastes are expected to be available together at many locations, but it also prevents overly optimistic results.
Furthermore, all the wastes generated from a particular source are not available for energy generation since some wastes have other competing uses. This is denoted by the waste or residue accessibility factor (or availability factor), which is the fraction or percentage of the total wastes available for a particular purpose (in this case, energy generation).29,51 The values of ‘W’ in eqn (2) were obtained after accounting for waste availabilities, as explained in the following sections. Accessibility factors for wastes were obtained from the literature relating to India (as far as possible); the corresponding sources are mentioned in the respective sections.
There are three standard temperature ranges for anaerobic digestion: psychrophilic (25 °C), mesophilic (35 °C to 42 °C) and thermophilic (50 °C to 55 °C).39,47 All the experimental results used in this study pertain to mesophilic conditions. This temperature range is best suited for India as appreciable additional heating of the digester chambers will not be required in most parts of the country during most of the year. The process is also economical and operates smoothly without much attention, giving good yields of biomethane.47,52
2.1.1. Agricultural and horticultural crop residues.
Agricultural and horticultural activities generate huge quantities of wastes in India, being major occupations almost throughout the country. The possibility of using the residues of major Indian crops for energy generation through both, direct combustion and biogas production, has been previously studied by some researchers.27–29,46,51,53–56 These studies, particularly those that consider anaerobic digestion (which constitute the minority),27,28,46 mostly only focus on some major crops like cereals, pulses, and sugarcane, and do not analyse the spatial state-wise distribution of these WTE resources, as presented in this paper. We consider wastes from all crops grown in India that are mentioned in the Agricultural and Horticultural Statistics.57–59 Furthermore, this study is different in using distinct experimentally determined BMPs from the academic literature for each crop residue type. This was deemed necessary for greater accuracy because the BMP varies widely across different crops, as may be observed from Table 8.
The residues produced from a particular crop are quantified by the residue-to-product ratio (RPR); it is the mass of residues produced per unit mass of the corresponding crop (or product),29,51,55 and is also called the residue-to-crop ratio (RCR). The multiplicative product of the annual crop production and the RPR, is called the total residue production (TRP).29,51 This TRP, when multiplied by the corresponding residue accessibility factor, gives the surplus residue production (SRP), which is the quantity of crop residues actually available for energy generation, with alternate uses accounted for.29,51 The SRP from each crop in a state was substituted for ‘W’ in eqn (2a) to obtain the corresponding annual biomethane generation; the sum over all crops gives the biomethane potential for each state. The RPRs, proximate characteristics (TS and VS), and BMPs of all crop residues included in this study are compiled in Table 8 in Appendix 1. The spatially different state-wise accessibility factors for all crop types were obtained from Hiloidhari et al.29 which classifies the crops as ‘cereals’, ‘oilseeds’, ‘sugarcane’, ‘horticulture’, ‘pulses’, and ‘others’. Cash crops and plantation crops do not fit into these categories and the accessibility factors for ‘others’ were used for them (except sugarcane). Distinct state-wise availability factors were used for different crop residues because they can vary by location and waste kind, depending on the type and extent of local use.
The types and state-wise quantities of agricultural and horticultural crops produced in India were obtained from the respective statistics published by the Indian Government57–59 (tea and coffee productions were obtained from the respective boards' statistics60,61 since these state-wise quantities are not reported in the Horticultural Statistics). These were taken for the Indian financial year of 2018–19 (starting in April 2018 and ending in March 2019) for agricultural products and 2017–18 for horticultural products, as state-wise horticultural statistics have not been published after 2017–18. The change in total horticultural production in 2018–19 from the 2017–18 value was less than 0.2%;58 so, this difference of one year is expected to introduce very minimal inconsistency. Moreover, absolute correctness of the production data has little value as they will change slightly every year; so, the biomethane potential will not remain constant but should be similar over consecutive years under ordinary circumstances. Also, peels of fruits and vegetables were excluded because most fruit peels are expected to end up in residential/municipal and industrial (food-processing) wastes. Loose flowers were the only category in the Horticultural Statistics that were excluded because the RPRs could not be ascertained; also, some flowers may potentially be digested too after their use, which could entail a detailed (separate) study. In any case, their contribution to the total biomethane generation will not impact the obtained results appreciably, based on the relatively low production quantities.
The BMPs and proximate compositions were sourced from the same studies as far as possible. If multiple sources reported very similar values (examples include rice and wheat), the mean of all similar observations was used. Moreover, different studies use different HRTs. When taking the mean values, either studies with similar HRTs were referred to, or yields from higher HRT experiments were scaled down if appropriately reported methane yield data (in days or weeks) were available. When experimental BMPs were not found for a particular crop, but the results of proximate analyses were available, a specific biogas yield of 300 m3 per tonne-VS was assumed with a methane content of 60%39,40,43,46 (indicated in Table 8). The BMPs, TS, VS, accessibility factors, and RPRs are potential sources of uncertainty. However, since these were obtained based on a detailed and comprehensive survey of the academic literature, such errors are expected to be negligible and the results obtained are dependable.
2.1.2. Orchard pruning wastes.
The use of orchard pruning wastes for biogas generation is an understudied subject internationally. These wastes have not been considered for energy potential calculations in India previously, to the best of our knowledge. Being organic, these residues can be managed through anaerobic digestion to generate biomethane, which will also prevent their burning and use as SBFs, thus reducing pollutant and GHG emissions.
There is a dearth of RPR data for most trees. So, data on the production of pruning wastes per hectare (for Europe) for each orchard type sourced from Boer et al.62 were multiplied with the state-wise land areas on which the orchards are grown (from the Horticultural Statistics59) to estimate the corresponding total quantity of pruning residues generated annually in each state. This was multiplied by the suggested availability factor of 0.8 for pruning residues63–65 to obtain the value of ‘W’ in eqn (2c). When the residue production for a particular tree was not found, the mean for other trees was used. These data were validated against the few available RPR values in the literature and were found to mostly agree well.63,66 The trees included and the corresponding residue production and RPR data are summarised in Table 9 in Appendix 1. The mean BMP of pruning residues was found to be 109 m3 per tonne-FM, as the average of olives,67 hazelnut,67 wood,68,69 and other fruit-tree residues.70 So, eqn (2c) was useful here. There could be slight differences in pruning residue generation in India owing to different growing conditions and pruning practices and a study in India is suggested to quantify these more accurately. However, this is not expected to modify the biomethane potentials drastically; as was observed for agricultural wastes, the RPRs differ only slightly (and almost negligibly) in different countries.
2.1.3. Animal wastes.
The state-wise energy generation potential of biogas from animal wastes in India has been evaluated in previously published papers;30,53 similar studies for other countries demonstrate a growing interest in this topic.37,71–80 While most papers assess the biogas generation from both animal excreta and slaughterhouse wastes (wastes generated from meat production), the latter are not included in the Indian studies;30,53 this gap is addressed in this paper. Furthermore, the waste generation quantities depend upon the live weights of the animals under consideration.30,37,53 Most studies across the world use the same values of animal weights.30,72–74,76 However, it is important to acknowledge the differences in weights of the same animal species in different countries/regions, owing to differences in breeds and other local conditions. For example, the live weight of cattle can vary between 135 kg and 800 kg, and that of small ruminants between 30 kg and 75 kg.71 In this paper, the live weights of 35 breeds of cows, 9 breeds of buffaloes, 16 breeds of goats, 46 breeds of sheep, 5 breeds of horses, and 2 breeds of camels raised in India were used to find the average masses of the respective species in India.81–84 For poultry, this was not necessary because the masses themselves being small (between 1.5 kg and 1.8 kg), the variation in masses is not appreciable. The weighted averages of live weights of the corresponding animals would be more appropriate, but these could not be evaluated in the absence of adequate breed population data. There is not sufficient data on the live weights of Indian pigs in the United Nations Food and Agriculture Organisation (FAO) database or elsewhere, so the value of 80 kg from Kaur et al.30 was used. Moreover, the quantity of wastes produced daily by large livestock is about 5–6% of their body weights, as mentioned by Avcioǧlu et al.;71 however, despite mentioning this and citing this source, most other papers use 9% for this value30,37,74,76 without an explanation. Although the difference of 3% is not that high, it significantly influences the final biogas yields because of the large numbers of cattle involved in the calculations; we hence used the value of 6%, as defined in Avcioglu et al.71
The mean values of specific waste generation factors, TS, VS, BMPs, and the corresponding availability factors are compiled from the literature in Table 10 in Appendix 1. The quantities of wastes generated in each state were obtained by multiplying animal populations (including those raised for meat production) from government data85 with the corresponding specific waste productions mentioned in Table 10. These were multiplied by the availability factors and then substituted for ‘W’ in eqn (2c), since the BMPs of animal wastes were available per unit mass of fresh matter (FM) directly (as seen in Table 10).
For slaughterhouse wastes, standard values from the literature were used. The waste generation factors are 20.4% of the body weights for large livestock (cattle, buffaloes), and 28% for small livestock.37,71–74,76 In case of poultry, the corresponding value is 20%.86 A biogas production of 300 m3 per tonne-FM was assumed for all slaughterhouse wastes, with a methane content of 60%.37,71–74,76 The accessibility factor was assumed to be 90% since almost all slaughterhouse wastes should be available for collection and treatment.
2.1.4. Municipal solid wastes (MSW).
Saini et al.87 studied the energy generation potential of 75 Indian cities using a combination of biogas generation and incineration techniques, mostly using data from the Central Pollution Control Board (CPCB) released in 2005.88 No paper was found to evaluate the state-wise biomethane potential of MSW.
Here, we consider the organic fraction of MSW in India's states for estimating their biomethane potentials; the state-wise MSW production quantities for the financial year of 2018–19 were sourced from CPCB's recent report.89 Results of experimental investigations on MSW characteristics, i.e., fractional organic content and TS, in 69 cities across India are compiled in Table 11 in Appendix 1 (these were only used to estimate numeric parameters of wastes in the different states, but not to assess the energy generations in these cities, as ours is a state-wise study). The organic content and TS of MSW in each state were obtained as the averages of the corresponding quantities in the cities situated in that state, since waste characteristics are influenced by local cultural practices and food habits, and show significant variations across cities (as also concluded by Saini et al.87). The mean organic fraction of MSW in each state was substituted for ‘W’ in eqn (2b) to obtain the potential biomethane yields. Paper and textiles/fabric contained in MSW were excluded from this ‘W’ because they are expected to be recycled (after collection). The mean BMP of organic MSW is 350 m3 per tonne-TS, based on multiple experimental studies in the literature,90–94 which was used for Yb,TS in eqn (2b). Also, all the generated (organic) MSW in India were considered available for energy generation because these do not have appreciable alternate uses. The lack of source segregation is problematic for this; this is discussed later.
Since waste characteristics are dynamic,95,96 recent studies were preferred while compiling them; data from Saini et al.87 and Kumar et al.11 (or CPCB data from 2005 (ref. 88)) were used only when published experimental results were not found for a particular city (which was rare and is mentioned in Table 11). The mean content of biodegradables in India's MSW was observed to be considerably higher than that reported in Saini et al.87 Most experimental investigations after 2005 record increasing shares of organic contents, especially in big cities.
2.1.5. Municipal wastewaters.
Municipal wastewaters or sewage contain organic matter, which can be treated by anaerobic digestion to produce biogas.97 This organic content is measured by the chemical oxygen demand (COD), which is the mass of oxygen required for the decay of organic matter present in a unit volume of water.98 Thus the “mass of COD removed” is a measure of the quantity of organic matter removed. The methane yields per unit mass of COD removed (Yb,COD) are comparable for different anaerobic reactor models (with similar HRTs); so, the mean of various observations in the literature was used. The mean rates of COD removal (CODremoved) and methane generation are 88%97,99–103 and 261 m3 per tonne-CODremoved,97,100,101,103 respectively. The average COD content of sewage is 314.9 mg L−1, based on multiple experimental investigations in various Indian cities.104–110 The annual state-wise sewage generation in 2018–19 was obtained from Government data.111 The total biomethane generation volume (V) from a particular volume of wastewater (Wwater) is found using eqn (3):97,112,113 | V = Wwater × COD × Yb,COD × CODremoved | (3) |
Here, COD is the mass of COD present per unit volume of wastewater, Yb,COD is the biomethane yield per unit mass of COD removed, and CODremoved is the fraction of COD removed during anaerobic digestion.
2.1.6. Wastes and wastewaters from industries, fisheries, and sericulture.
The biomethane generation potentials of wastes and wastewaters from industries, sericulture, and fisheries, assessed using the same methodology as this paper, are presented in Dey & Thomson.113 State-wise biomethane yields are reported there for fish and sericulture wastes and for wastewaters of slaughterhouses, palm oil mills, silk, and fish processing. These were added to the state-wise results in this paper (biomethane from palm oil mill effluent was added to the state-wise yields of ‘oilseeds and palm oil’ in agricultural wastes). For most other industrial wastes, the biomethane potential for the entire country has been reported by Dey & Thomson,113 owing to a lack of appropriate state-wise data; this value is 3113.9 million m3 and is added to India's total potential.
The biomethane potentials for each state and waste category obtained were summed up to calculate the total biomethane generation in the country. A visual comparison of the biomethane yields per tonne of fresh matter for all solid wastes considered in this paper (except industrial wastes, which have not particularly been compiled and assessed here) is presented in Fig. 1. It is seen that the methane yield per unit mass of fresh matter is usually greater for crop wastes than for animal wastes; so, increasing the utilisation of the former for biogas generation is highly beneficial.
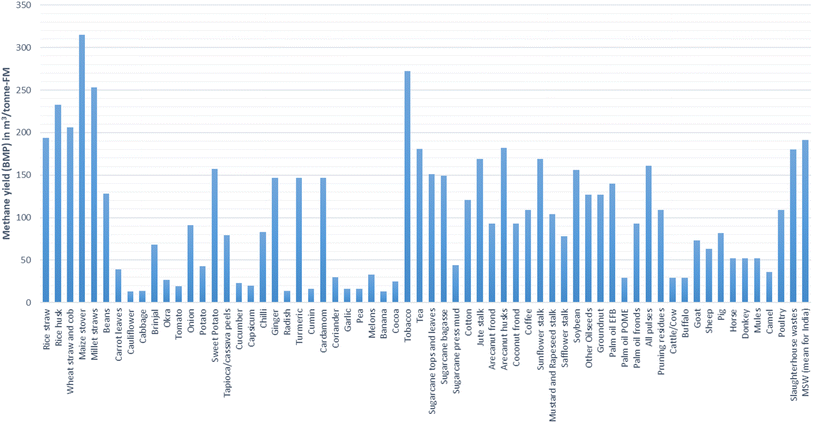 |
| Fig. 1 Methane yields (BMP) per tonne of solid wastes (fresh matter); data and references in Appendix 1. | |
2.2. Energy generation and emissions assessment
Three end use scenarios for biomethane were considered, to assess the potential of displacing fossil fuels and the consequent annual reduction in GHG and PM emissions. These are: electricity generation, cooking fuel, and road transportation fuel. An annual GHG emissions assessment was carried out to quantify the climate benefits of the GHGs avoided in one year on using biomethane (from wastes) to displace fossil fuels in India based on the 100 year global warming potentials (GWPs) of the GHGs involved, according to IPCC guidelines. The PM emission savings due to the avoidance of FBCR were also evaluated. Here, ‘emissions’ refer to both GHGs and PM, unless explicitly clarified. Also, the terms ‘GHG emissions’ and ‘carbon emissions’ have been used interchangeably.
The system boundary for the emissions assessment is explained in Table 1, and the procedure to assess emissions, in the following subsections. All GHG emission conversions and comparisons were done considering a functional unit of 1 MJ of energy, electrical or thermal (the latter for cooking and transportation fuels). For electricity, the functional unit of 1 kWh was used too.
Table 1 System boundary for emissions assessment
Process |
Included (I) or excluded (E) |
Reasons and notes |
Emissions from the respective sectorial activities (like agriculture, animal husbandry, industrial units, etc.) |
E |
Should be allocated to the respective products or services |
Transportation of MSW |
E |
Would occur even on not generating biogas from MSW |
Transportation of crop residues to biogas plants |
E |
Would depend upon the individual biogas plants' transportation modes and waste sources, and the distances between the plants and these sources |
Positive impacts of the field application of the digestate from biogas plants, thus displacing chemical fertilisers |
E |
Beyond the scope of this paper to assess the quantities of digestate produced, the related transportation required, and the acceptability of the digestate fertiliser to farmers |
Particulate emissions from all activities involving the burning of fossil fuels like electricity generation and transportation |
E |
Beyond the scope of this paper to assess the degree of use of mitigation measures in India and the effectiveness of these measures |
Handling and transportation of biomethane to end use consumers |
E |
It depends on how the biomethane supply chains are designed, and emissions can be kept really low if power plants are located adjacent to or very close to biogas plants, or if the cooking fuel is delivered to consumers in the vicinity of biogas plants and using eco-friendly modes of transport. Long distance transportation could be kept low, and the desired amounts could be transported by natural gas pipelines to minimise emissions.114 Furthermore, in case of the use of biomethane as transport fuel, there is no need to consider these emissions because they will be eliminated too. Methane leakages during transportation are covered already in the 3% |
GHGs involved in the construction and operation of biogas plants, including feedstock and digestate handling (not final use as fertiliser). These are collectively termed as the ‘net GHG impact of biogas plants’ |
I |
Though this impact is small and considered negligible by some authors,115,116 it cannot be neglected fully, according to a study in India117 |
Methane leakages across the supply chain of biogas |
I |
Leakages occur from the biogas plants and from upgradation and transportation activities.118–120 About 1% methane leakage occurs from biogas plants.118,119 The leakage from upgradation activities is technology-dependent: amine-based upgradation, water-based scrubbing, and membrane technology cause methane leakages of 0.04–0.07%, 1.97%, and 0.56% respectively.120 There may be further leakages during biogas transportation, bottling, and combustion. Based on these figures, a 3% cumulative methane leakage across the biomethane supply chain was assumed |
Avoided GHG and PM emissions from the prevention of the field burning of crop residues |
I |
Using the crop residues as feedstocks for biogas generation prevents their field burning; the procedure is explained in section 2.2.3 |
Avoided methane emissions from municipal garbage dumps |
I |
Methane is released from municipal garbage dumps and landfills due to the anaerobic digestion of wastes in the deep layers where the supply of atmospheric oxygen is limited;121–123 this is avoided on using up organic MSW for biogas generation. The procedure is explained in Section 2.2.3 |
GHG emissions avoided due to the displacement of fossil fuels by biomethane |
I |
This is the chief purpose of this paper; the procedure is explained in Section 2.2.2 |
Here, the GWP of biogenic CO2 (emitted on burning biomethane and crop residues) is considered zero. Any methane emissions were considered in the assessment since the 100 year GWPs of both, biogenic and fossil methane, are much greater than that of CO2,124 being 27 and 29.8 times respectively125 (lower for biogenic since that carbon is still part of the natural carbon cycle). The GWP of nitrous oxide (N2O) is 273, considering 100 years of cumulative forcing.125
2.2.1. Emissions from biogas production and supply chain.
The net GHG impact of biogas plants per functional unit, was adapted from Singh et al.117 Moreover, methane leakages occur throughout the production and supply chain of biomethane, which were assumed to be 3% (as explained in Table 1). The emissions from leakage per functional unit were added to the GHG impact of biogas plants to obtain the total GHG emissions from biomethane per functional unit. This was compared with the GHG intensities of present-day energy sources to assess avoided emissions.
2.2.2. Fossil fuel displacement and avoided emissions.
Fossil fuel displacement and emissions from the three end use scenarios were analysed as follows (all related efficiencies and LCVs are listed in Tables 2 and 3).
Table 2 Lower calorific values of fuels
Fuel |
Lower calorific value (LCV) |
References |
Methane |
35.8 MJ m−3 or 50 MJ kg−1 |
134–137
|
Natural gas |
36.6 MJ m−3 |
137
|
Petrol (gasoline) |
44 MJ kg−1 |
138
|
Diesel (high speed diesel oil) |
42.6 MJ kg−1 |
124
|
Liquified petroleum gas (LPG) |
45.24 MJ kg−1 |
119
|
Kerosene |
42.86 MJ kg−1 |
119
|
Table 3 Efficiencies of energy systems and engines
Energy system/engine |
Efficiency (%) |
References |
Combined cycle power plant (CCPP) |
60 |
126–129, 139 and 140 |
Methane cookstove |
55 |
119
|
LPG cookstove |
57 |
119
|
Kerosene cookstove |
47 |
119
|
Petrol vehicle |
36 |
141 and 142 |
Diesel vehicle |
44 |
141–144
|
Methane/natural gas vehicle |
38.2 |
145 and 146 |
LPG vehicles |
45 |
147
|
Electricity.
The biomethane was assumed to be combusted for electricity generation in combined cycle gas turbines (CCGTs), which have the highest efficiencies (in the range of 60–65%) based on the lower calorific value (LCV) of the gaseous fuel.126–129 India generated 1584 TWh and consumed 1210 TWh of electricity in 2018–19 (ref. 3) with an average carbon intensity of 675.1 gCO2eq./kWh;130 the generation potential and GHG impact of electricity from biomethane were juxtaposed against these values. There is a minor inconsistency in the electricity generation data available from the IEA14 and the Indian Government;3 we preferred the latter and used IEA data only when something was unavailable in the Government Statistics (results were unaffected by this; only the discussion of the state-of-the-art required data from both sources).
Cooking fuel.
The total final thermal energy used for cooking from a particular fuel in India was evaluated by multiplying its LCV with the corresponding cookstove/burner efficiency (Table 3) and its quantity consumed (for biomethane, the quantity available) annually. The LPG and kerosene consumptions for cooking in India in 2018–19 in the domestic and commercial sectors were sourced from India's Government Statistics,131 while PNG (piped natural gas) use was deduced using Government131 and IEA14 data on natural gas use as cooking and transportation fuels. Therefore, the degree of displacement of these fuels by biomethane was estimated. Additionally, the annual per capita final energy consumption for cooking in India is around 0.77 GJ (as of 2019).132 This was multiplied by India's population in 2019 (ref. 1) to estimate the net annual cooking fuel demand in the country, to compare with the final thermal energy available from biomethane. In the process, the approximate extent of use of SBFs like crop residues, cattle dung cakes, and wood, for cooking in India, was quantified (which is absent in reports and the literature).
To assess the GHG savings, GHG emissions from the life cycle stages of LPG and kerosene were adapted from Garg et al.114 For natural gas, the GHG intensity was adapted from Agrawal et al.133 The GHG savings from the displacement of SBFs (chiefly methane, N2O, and also black carbon, as CO2 would still be biogenic), coal, and charcoal used for cooking have not been assessed here due to the unavailability of recent data on their usage and types, after the countrywide LPG drive.
Road transportation fuel.
The purified and upgraded biomethane could alternatively be used as a fuel in road vehicles. The quantities of petrol, diesel, and LPG used for road transportation in India in 2018–19 are available in India's Petroleum Statistics,131 while the corresponding energy use from natural gas was taken from IEA data.14 The LCV of each transportation fuel was scaled down by the maximum brake thermal efficiency of the corresponding automobile engine (Table 3) and multiplied with its quantity consumed/available annually, to approximate the energy it provides to road vehicles in India. These were compared to find the quantities of present-day fuels that could be displaced by biomethane. The life cycle GHG emissions per MJ of final energy consumed from petrol, diesel, and LPG in India (for transportation purposes) were evaluated from Garg et al.,114 and those of CNG (compressed natural gas) from Agrawal et al.133 Our results on GHG savings are modest and the actual on-road emissions are expected to be higher than our estimates as these are the maximum engine efficiencies, so, the true fuel consumptions are higher, rendering greater emissions.
2.2.3. Other avoided emissions.
Crop residue burning.
The use of agricultural wastes for biomethane generation will prevent their field burning. The avoided emissions from this were estimated based on mean values of emission factors (EFs, in g kg−1) from the literature.148–155 No experimental investigations to estimate emission factors from FBCR in India were found. While Ravindra et al.148 do quantify the atmospheric emissions from FBCR in India, they use emission factors from measurements conducted in other countries. Thus, there was no reason to prefer the results of one paper to another, and the mean values of EFs from multiple studies in the literature148–155 were used.
Other studies indicate that residues of only certain crops undergo field burning in India;25,148 the same was assumed in this paper (Table 4). Orchard pruning residues should be included here too, but the fraction of these burnt for clearing or cooking cannot be ascertained in the absence of data; they were thus excluded.
Table 4 Mean values of emission factors (EFs, in g kg−1) for crop residue burning and other related details obtained from the literature148–155
Pollutant details |
Rice |
Wheat |
Maize/corn |
Millets |
Pulses |
Oilseeds |
Sugarcane |
Cotton |
Jute |
PM2.5 (EF) |
7.52 |
6.76 |
9.10 |
4.10 |
9.54 |
11.50 |
3.91 |
6.42 |
6.55 |
PM10 (EF) |
5.03 |
6.24 |
5.62 |
4.30 |
8.80 |
8.05 |
4.83 |
7.84 |
4.35 |
CH4 (EF) |
3.57 |
2.45 |
3.21 |
4.40 |
2.90 |
3.00 |
2.08 |
3.50 |
4.08 |
N2O (EF) |
0.48 |
0.74 |
0.74 |
0.74 |
0.74 |
0.74 |
0.74 |
0.74 |
0.74 |
Black carbon (EF) |
0.40 |
0.54 |
0.63 |
0.70 |
0.72 |
1.70 |
0.65 |
0.72 |
0.70 |
Burning efficiency |
0.89 |
0.86 |
0.92 |
0.90 |
0.90 |
0.82 |
0.68 |
0.90 |
0.90 |
Accessibility factors29 |
0.29 |
0.29 |
0.29 |
0.29 |
0.38 |
0.30 |
0.39 |
0.38 |
0.38 |
IPCC fraction of residues burnt148 |
0.25 |
0.25 |
0.25 |
0.25 |
0.25 |
0.25 |
0.25 |
0.25 |
0.25 |
CO2 has not been considered while estimating the global warming impact of FBCR burning as these emissions also constitute biogenic carbon. Black carbon (BC) also influences climate change,156 and has been considered separately to estimate the climate impact since it is usually not included in other GHG assessments. Its 100 year GWP is 680–730 times that of CO2 (ref. 157 and 158) (the lower value of 680 was used). The climate impact of BC emitted from FBCR only has been assessed in this paper.
MSW open dumping.
Emissions from the open dumping of MSW in India (which are expected to be avoided on producing biomethane from MSW) were estimated using the IPCC default methodology,121,159,160 with an assumed correction factor to better represent the true methane emissions and ensure that the obtained savings are conservative. The method, along with the associated uncertainties, is described in Appendix 2.
These additional emission savings (from prevented FBCR and MSW dumping) were added to the avoided emissions from the displacement of fossil fuels to obtain the total avoided emissions.
3 Results and discussions
3.1. Waste to biomethane potential assessment
Animal wastes are found to produce the largest volumes of biomethane in India, followed by agricultural and horticultural residues, as can be seen in Table 5 (greater detail on the biomethane yields for each category of crops and animal wastes are presented in the ESI†). The larger states with higher populations, more agriculture and animal husbandry, and consequently higher waste generations, are the larger biomethane producers too. The state of Uttar Pradesh thus has the highest biomethane potential, followed by Madhya Pradesh, Maharashtra, Rajasthan, Bihar, and West Bengal. The union territories like Goa and Puducherry have low biomethane yields.
Table 5 State-wise annual biomethane generation potential from wastes and wastewaters across various sectors
State/union territory |
Methane in milliona m3 |
Agriculture and horticulture wastes |
Animal wastes (including slaughterhouse wastes and wastewaters) |
MSW |
Municipal wastewaters |
Fisheriesb (including processing wastewaters)113 |
Sericulturec (including processing wastewaters)113 |
Total |
The results are rounded off to 5 significant figures.
These are from the results of Dey & Thomson113 and were not obtained in the analysis in this paper, but were used here.
The Agricultural Statistics57,58 do not mention the quantities of all crops grown in each state, but only give data for the major states. The category ‘others’ refers to the agricultural produce not allocated to any particular state, and is the cumulative production of all the states not explicitly mentioned.
|
Andhra Pradesh |
2391.8 |
2573.2 |
197.06 |
75.790 |
53.590 |
20.330 |
5311.7 |
Arunachal Pradesh |
11.790 |
132.11 |
8.3800 |
1.320 |
0 |
0.1000 |
153.70 |
Assam |
648.05 |
2077.7 |
51.270 |
18.560 |
0 |
8.9100 |
2804.5 |
Bihar |
2545.9 |
4575.0 |
79.470 |
49.600 |
0 |
0.1400 |
7250.1 |
Chhattisgarh |
771.43 |
1853.6 |
55.990 |
25.110 |
0.1600 |
0.9800 |
2707.2 |
Goa |
0.2600 |
22.620 |
7.7700 |
3.8300 |
0 |
0 |
34.480 |
Gujarat |
2164.9 |
4081.9 |
392.40 |
108.74 |
84.450 |
0 |
6832.4 |
Haryana |
2943.3 |
1376.0 |
157.15 |
37.300 |
0 |
0 |
4513.8 |
Himachal Pradesh |
141.59 |
573.59 |
9.4800 |
2.9000 |
0 |
0.0900 |
727.88 |
Jammu, Kashmir, and Ladakh |
87.990 |
634.70 |
37.510 |
14.440 |
0 |
0.3200 |
781.06 |
Jharkhand |
150.51 |
2215.2 |
106.25 |
33.530 |
0 |
6.4600 |
2536.8 |
Karnataka |
3259.1 |
2642.9 |
507.08 |
99.710 |
15.200 |
31.510 |
6524.2 |
Kerala |
288.68 |
510.58 |
154.24 |
67.370 |
29.170 |
0.0500 |
1050.3 |
Madhya Pradesh |
4871.4 |
5173.0 |
222.70 |
84.850 |
0 |
0.2200 |
10 353 |
Maharashtra |
4505.0 |
4124.3 |
812.20 |
214.97 |
25.340 |
1.4100 |
9682.7 |
Manipur |
9.8300 |
75.290 |
8.0300 |
3.4800 |
0 |
0.8200 |
98.600 |
Meghalaya |
15.970 |
213.52 |
4.2600 |
2.5100 |
0 |
1.9800 |
236.44 |
Mizoram |
16.650 |
37.440 |
8.0700 |
2.3800 |
0 |
0.1600 |
65.630 |
Nagaland |
21.500 |
61.590 |
7.4900 |
2.4300 |
0.1600 |
1.1000 |
93.530 |
Odisha |
1001.0 |
1905.5 |
52.240 |
29.590 |
10.140 |
0.3500 |
2998.5 |
Punjab |
4208.7 |
1519.5 |
137.30 |
43.930 |
0 |
0.0100 |
5909.4 |
Rajasthan |
2760.9 |
5350.3 |
205.68 |
72.230 |
0 |
0 |
8389.1 |
Sikkim |
7.4900 |
44.020 |
2.9900 |
0.6300 |
0 |
0 |
60.730 |
Tamil Nadu |
2694.6 |
2972.8 |
510.14 |
147.81 |
29.940 |
5.6300 |
6355.9 |
Telengana |
3019.4 |
1857.1 |
368.76 |
44.110 |
1.3800 |
0.6100 |
5290.9 |
Tripura |
25.380 |
154.20 |
18.090 |
4.0700 |
0 |
0.1800 |
202.52 |
Uttar Pradesh |
11 831 |
10 399 |
537.58 |
188.07 |
0 |
0.7800 |
22 955 |
Uttarakhand |
288.90 |
554.03 |
64.940 |
13.070 |
0 |
0.1000 |
927.41 |
West Bengal |
2488.9 |
3790.3 |
472.86 |
123.20 |
17.190 |
6.5100 |
6892.5 |
Andaman and Nicobar |
0 |
13.880 |
2.4800 |
0.5800 |
1.3800 |
0 |
18.320 |
Chandigarh |
0 |
5.9800 |
16.440 |
4.3300 |
0 |
0 |
26.750 |
Daman, Diu, D & N Haveli |
0 |
6.9000 |
3.0000 |
1.4600 |
3.9900 |
0 |
15.350 |
Delhi |
0 |
60.430 |
539.29 |
109.69 |
0 |
0 |
709.41 |
Lakshadweep |
0 |
1.1200 |
1.3900 |
0.2100 |
0.3000 |
0 |
3.0200 |
Puducherry |
0 |
22.240 |
15.850 |
3.5900 |
0.3000 |
0 |
41.980 |
Othersc |
3672.8 |
0 |
0 |
0 |
0 |
0 |
3672.8 |
Total
|
56 844
|
61 611
|
5775.8
|
1635.4
|
272.69
|
88.750
|
126 228
|
The energy available from this biomethane, after considering a methane leakage of 3% during storage, upgradation, and transportation, can displace present-day fuels and electricity, as evaluated in Tables 6 and 7. Table 6 also denotes the gap between the potential and the state-of-the-art. The electrical energy from this 125.46 billion m3 of biomethane (=748.59 TWh) can supply 47% of the electricity generated in the country (based on the gross generation of 1585 TWh in the financial year of 2018–19 (ref. 3)), and is numerically equal to 62% of the country's net electricity demand (1210 TWh (ref. 3)). Currently, the installed capacity of biomass and WTE generators is only sufficient to capture 12% of the potential.
Table 6 State-wise annual thermal energy and electricity generation potentials (2018–19) from biomethane and comparisons with the WTE state-of-the-art
State/union territory |
Annual biomethane after 3% leakage (million m3) |
Annual gross heat energy potentiala (PJ) |
Resultant annual electrical energy potentiala (TWh) |
Potential electric power installation capacitya (MW) |
Biomass power and WTE installed capacity in MW (March 2021)161 |
Harnessed in biomass and WTE (% of biogas energy potential) |
Gross heat energy refers to the total heat available on combusting all the biomethane generated annually; electrical energy refers to the electricity available annually when all this heat is converted to electricity in a combined cycle power plant (at 60% efficiency). Electric power is the instantaneous power that can be generated throughout the year based on the generated electrical energy and gives an idea of the desired installed capacity for waste-to-electricity (WTE) generation.
Also includes the generation from ‘others’ (states) for agricultural wastes, which include the states not explicitly mentioned in the agricultural statistics; so, the total does not directly add up from the column. This also excludes industrial wastes and wastewaters, other than palm oil mills, fisheries, sericulture, and slaughterhouses. The cumulative results on including all industrial wastes and wastewaters are presented in Table 7.
This becomes 11.92% on considering all industrial wastes and wastewaters from the next table.
|
Andhra Pradesh |
5152.4 |
184.46 |
30.742 |
3509.4 |
506.83 |
14.44 |
Arunachal Pradesh |
149.09 |
5.3374 |
0.8896 |
101.55 |
0 |
0 |
Assam |
2720.4 |
97.390 |
16.232 |
1852.9 |
2 |
0.11 |
Bihar |
7032.6 |
251.77 |
41.961 |
4790.1 |
124.7 |
2.60 |
Chhattisgarh |
2626.0 |
94.012 |
15.669 |
1788.7 |
244.9 |
13.69 |
Goa |
33.450 |
1.1975 |
0.1996 |
22.780 |
0.34 |
1.49 |
Gujarat |
6627.4 |
237.26 |
39.544 |
4514.1 |
77.3 |
1.71 |
Haryana |
4378.4 |
156.75 |
26.125 |
2982.3 |
211.86 |
7.1 |
Himachal Pradesh |
706.04 |
25.276 |
4.2127 |
480.90 |
9.2 |
1.91 |
Jammu, Kashmir, and Ladakh |
757.63 |
27.123 |
4.5205 |
516.04 |
0 |
0 |
Jharkhand |
2460.7 |
88.093 |
14.682 |
1676.1 |
4.3 |
0.26 |
Karnataka |
6328.5 |
226.56 |
37.760 |
4310.5 |
1888.3 |
43.81 |
Kerala |
1018.8 |
36.471 |
6.0790 |
693.90 |
2.27 |
0.33 |
Madhya Pradesh |
10 043 |
359.53 |
59.922 |
6840.4 |
122.75 |
1.79 |
Maharashtra |
9392.2 |
336.24 |
56.040 |
6397.3 |
2596.99 |
40.6 |
Manipur |
95.640 |
3.4239 |
0.5707 |
65.140 |
0 |
0 |
Meghalaya |
229.35 |
8.2107 |
1.3685 |
156.22 |
13.8 |
8.83 |
Mizoram |
63.660 |
2.2790 |
0.3798 |
43.360 |
0 |
0 |
Nagaland |
90.720 |
3.2478 |
0.5413 |
61.790 |
0 |
0 |
Odisha |
2908.6 |
104.13 |
17.354 |
1981.1 |
59.22 |
2.99 |
Punjab |
5732.1 |
205.21 |
34.202 |
3904.3 |
484.2 |
12.4 |
Rajasthan |
8137.4 |
291.32 |
48.553 |
5542.61 |
121.25 |
2.19 |
Sikkim |
58.910 |
2.1090 |
0.3515 |
40.130 |
0 |
0 |
Tamil Nadu |
6165.2 |
220.72 |
36.786 |
4199.3 |
1019.05 |
24.27 |
Telengana |
5132.1 |
183.73 |
30.622 |
3495.6 |
205.9 |
5.89 |
Tripura |
196.44 |
7.0326 |
1.1721 |
133.80 |
0 |
0 |
Uttar Pradesh |
22267 |
797.14 |
132.86 |
15 166 |
2117.26 |
13.96 |
Uttarakhand |
899.59 |
32.205 |
5.3676 |
612.73 |
0 |
0 |
West Bengal |
6685.8 |
239.35 |
39.892 |
4553.9 |
319.92 |
7.03 |
Andaman and Nicobar |
17.770 |
0.6362 |
0.1060 |
12.100 |
0 |
0 |
Chandigarh |
25.950 |
0.9290 |
0.1548 |
17.680 |
0 |
0 |
Daman, Diu, D & N Haveli |
14.890 |
0.5331 |
0.0888 |
10.140 |
0 |
0 |
Delhi |
688.13 |
24.635 |
4.10 584 |
468.70 |
52 |
11.09 |
Lakshadweep |
2.9300 |
0.1049 |
0.0175 |
2.0000 |
0 |
0 |
Puducherry |
40.720 |
1.4578 |
0.2430 |
27.740 |
0 |
0 |
Total
|
122 441
|
4383.4
|
730.57
|
83 398 |
10 184
|
12.21
|
Table 7 Important cumulative results
Annual biomethane generation from all sectors except industries, from the state-wise study (billion m3) after considering 3% leakage |
122.44 |
Biomethane generation from industrial wastes and wastewaters (million m3) after considering leakage (except POME and wastewaters from slaughterhouses, fish and silk processing)113 |
3020.5 |
India's total biomethane potential from wastes (billion m3), after accounting for leakage |
125.46 |
Total heat energy available from biomethane annually, based on LCV (in PJ) |
4491.5 |
Average electric power potential (in GW) |
85.455 |
Total annual electrical energy potential (in TWh) |
748.59 |
It is interesting to note the biomethane yields from the sectors mostly neglected in previous studies on India's bioenergy potential: 2676.1, 13
348, 739.21, 273, 88.75, and 3113.9 million m3 of biomethane (excluding leakages) from wastes of horticultural crops, cash crops, slaughterhouses, fisheries, sericulture, and industries respectively. These contribute 16% to the total electrical energy potential.
The biomethane can alternatively be used as a fuel for cooking or road transportation instead of electricity generation. Fig. 2 illustrates the proportion of the total final thermal energy potential of biomethane that would be needed to meet India's total energy demand for each of the respective sectors, adjusted according to the efficiencies of the cookstoves and engines. If all the biomethane is used in transportation, it would be able to displace 94% of the dirtiest petrol and diesel and about 91% of the total, when LPG and CNG are included (derived from Fig. 2). On the other hand, only about 43% of the biomethane would be needed to displace 100% of the cooking fuels used in the country; the remaining biomethane still being available for other applications. If SBFs, coal and charcoal are not considered, less than 32% of the biomethane is needed to displace the popular cooking fuels of LPG, PNG, and kerosene, in India.
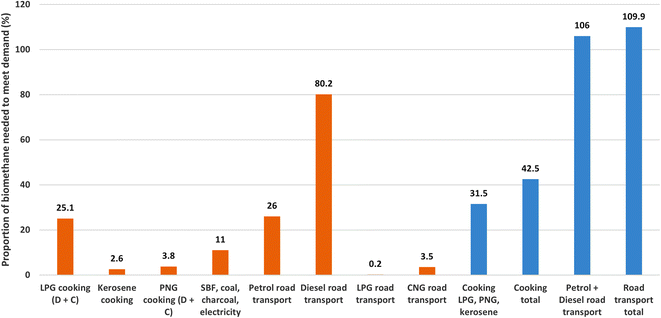 |
| Fig. 2 The proportion of the maximum potential biomethane production needed to meet the heat energy demand of each traditional fuel in India's cooking and transportation sectors in 2018–19. This includes Liquified Petroleum Gas (LPG), Piped Natural Gas (PNG) and Solid Biomass Fuels (SBF). Note that (D + C) means domestic and commercial sectors. | |
3.2. GHG and particulate emissions assessment
The total GHG impact of biogas plants was found to be 691 gCO2eq./m3-CH4, including the methane leakage of 3% throughout the supply chain but excluding the emissions that occur during the transportation of feedstocks to biogas plants. Almost 84% of the emissions come from methane leakages, so reducing leakages to below 3% would be greatly beneficial in terms of climate impact and biomethane availability.
Fig. 3 shows the relative environmental performances of all cooking and transportation fuels based on the corresponding maximum cookstove/engine thermal efficiencies (brake thermal efficiencies for vehicle engines). This includes an estimate of the GHG emissions from using biomethane for that particular application based on typical burner/engine thermal efficiencies. The emission savings per MJ from the displacement of each fuel with biomethane, can be easily obtained from Fig. 3 by subtraction.
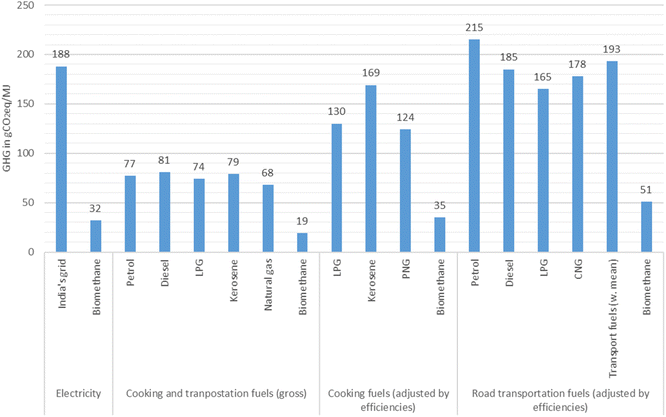 |
| Fig. 3 Comparison of GHG emissions from traditional fuels and biomethane, and the corresponding emissions avoided on using biomethane, both based on gross energy available and adjusted according to burner/engine thermal efficiencies (does not include black carbon); w. mean = weighted mean (according to gross energy consumption from those fuels). | |
In Fig. 3, the mean GHG intensity of the existing transport fuels combined is weighted according to the consumptions (by gross energy) in India, of the four fossil fuels mentioned. This is dominated by the carbon intensities of petrol and diesel, as these are the dominant fuels for transportation in India, such that the mean emissions remain 193 gCO2eq./MJ if LPG and CNG are neglected. Petrol vehicles are the worst with respect to GHG impact (based on engine performance), although diesel causes greater emissions based on gross energy generation. LPG emits the least GHGs among the fossil fuels used for transportation, followed by CNG. This is reversed in the case of cooking fuel, where PNG is the least carbon-intensive fossil fuel; this is attributable to the higher efficiencies of natural gas cookstoves as compared to vehicle engines. All these fossil fuels emit non-biogenic CO2 on burning, which could be replaced by biogenic CO2 on using biomethane. The GHG emissions that occur during the extraction and refining of the fossil fuels would also be avoided.
Using the functional unit of 1 kWh for electrical energy, the GHG emissions from India's electricity grid are 675.1 gCO2eq./kWh and those from biomethane are 115.8 gCO2eq./kWh. Thus, biomethane can save about 559.3 gCO2eq./kWh (of electricity generated).
Crop residue burning annually generates between 37.41 million tonnes of CO2-equivalent (using IPCC burning fraction data148) and 43.68 million tonnes of CO2-equivalent (using SRP29) of GHGs in the form of methane and nitrous oxide. If black carbon is included, the GHG emissions increase to between 100.8 million tonnes of CO2-equivalent (IPCC) and 114.52 million tonnes of CO2-equivalent (SRP). Additionally, the lower and upper limits for PM2.5 emissions from FBCR are 1019 and 1159 thousand tonnes. The corresponding values for PM10 are 814 and 943 thousand tonnes. These emissions can be eliminated if biomethane is produced from these crop residues, also noting that negligible amounts of particulates are released during the construction of biogas plants and the combustion of the gas.119 This PM reduction has direct benefits for public health and the economy. Biogas schemes can also indirectly improve solar energy yields by reducing atmospheric PM concentrations, thus contributing further to sustainable energy transitions and climate change mitigation.162
Methane emissions from the open dumping of MSW generated annually (calculated in Appendix 2) are 4.49 million tonnes of CO2-equivalent (=234 million m3 of methane). Without using the correction factor (Appendix 2), these GHG emissions are 20.21 million tonnes of CO2-equivalent; the former is used here to obtain less optimistic estimates for avoided emissions. These emissions can be avoided on converting biodegradable municipal wastes into biomethane, or at least capturing landfill gas. The former is a better option as the yields of methane are higher on conducting anaerobic digestion in a biogas plant, under conditions that are more suitable for methane generation, as can be observed from Table 5.
The GHG savings on replacing present-day fuels with biomethane, and adding the corresponding cumulative savings achievable on eliminating FBCR (using IPCC burning fraction data, which are conservative) and MSW open dumping, are illustrated in Fig. 4. For cooking fuels, the values include the complete displacement of LPG, PNG, and kerosene only, while the emission savings from displacing all SBFs, coal, and charcoal were excluded for lack of appropriate data. So, greater emission savings are to be expected from the cooking sector.
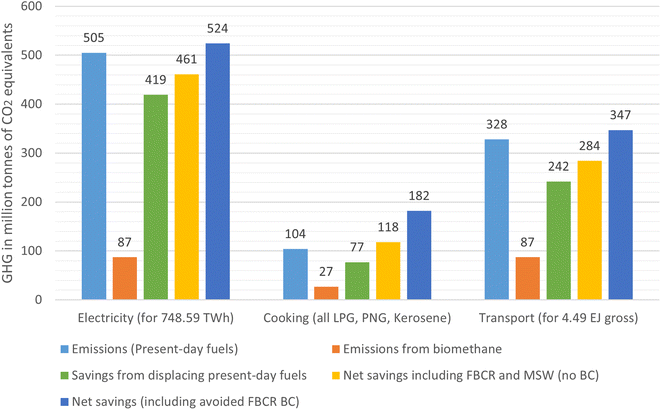 |
| Fig. 4 Cumulative annual GHG emissions avoided on using biomethane, considering the displacement of present-day fuels, and including the emissions avoided from crop residue burning and MSW open dumping (additional 284 million tonnes of savings for using the biomethane remaining after cooking fuel application (i.e., 68% of the total generated biomethane), for electricity generation). Note: the black carbon avoided is only from the FBCR circumvented; it does not include avoided black carbon from fossil fuel burning. | |
The avoided cumulative emissions are highest for the electricity sector, followed by the road transportation sector. This has to do with the greater gross consumption of energy as electricity, compared to transportation and other fuels in India annually, and the high carbon intensity of electricity generation owing to the predominance of coal. It is also noteworthy that cooking fuel displacement denoted in Fig. 4 constitutes 32% of the energy potential of biomethane only (since SBFs, coal, and charcoal are excluded here). If the remaining biomethane is used for electricity generation, it will additionally save 284 million tonnes of CO2-equivalent, which can be added to the savings from cooking to reflect the impact of all the available biomethane. This would raise the total GHG savings from cooking (+electricity generation) to 402 million tonnes (without BC) and 466 million tonnes (including avoided BC from FBCR), i.e., greater than the savings from transportation.
3.3. Limitations and validation
The results presented in this paper are only for a snapshot of one year, i.e., the financial year of 2018–19. While this is a limitation, the paper gives a comprehensive idea of the annual energy generation potential from wastes in India's states and the whole country, and the corresponding GHG abatement. The results are sensitive to changes in annual waste and biomethane production rates from the various sectors, which change every year, but the differences are not expected to be extremely large; for instance, the yearly variation in food grain production in India has typically been below 6% since 2005, and less than 1% for some consecutive years.57,58. (Horticultural products show a similar trend of annual increase.59) Furthermore, the biomethane generation potential is not expected to reduce, but rather increase with future increases in population, GDP (and thus higher standards of living), agricultural produce, and industrial output. This trend is already visible in the data; for example, food grain production has generally shown an increase over the last 5 decades in India, owing to an increase in both production areas and yields.57,58 It is thus expected that a re-analysis using averaged historical data over several years would further underestimate the biomethane potential.
The accessibility factors of crop residues and animal wastes used here already consider the reduction in available material due to some being already allocated to alternate usages, including composting. Since biogas generation will also produce digestate fertiliser, using wastes for biogas generation rather than composting could be better for sustainability and profitability; consequently, the biomethane potentials could increase beyond those calculated here. Using biogas digestate to replace synthetic fertilisers would further raise the GHG savings from the findings of this paper, by removing the emissions incurred for their production.163
The reported GHG emissions from biogas plants (and thus the abatement potential) are also sensitive to the biomethane leakage rates since the leakages constitute most of the life cycle GHG emissions. As detailed in Section 2.2, a methane leakage rate of 3% was assumed for this study. This is on the higher side for biogas plants,119 but expectedly realistic (also giving modest and less optimistic abatement) based on emissions from upgradation and transportation.124 Further data on real leakage rates for installations and pipelines in India would be needed to confirm these values. Also, for our assessment, the GHG impacts of construction of a biogas plant that generates electricity from sewage were adapted from Singh et al.117 A full LCA of biogas plants for different kinds of feedstocks and locations is missing and is suggested as further study; this can further improve the accuracy of the obtained GHG emissions and abatement potential, since multiple waste streams are typically involved.
No additional GHG impacts are considered from the actual emissions that occur while transporting wastes to biogas plants and then biomethane to the end user (except for the leakage mentioned previously). While the inclusion of these would give better estimates of GHG abatement, care would need to be taken to avoid double-counting the transportation impacts of wastes (which might be attributed to the life cycle of the consumed product, rather than its waste). Furthermore, transport distances are dependent on plant location, which requires further design of WTE infrastructure in India. Strategically locating biogas plants based on either the availability of waste resources or the proximity to end users, could maintain very low transportation GHG emissions and render the true emissions to be very close to these estimates.
The avoided BC emissions discussed in Section 3.2 consider only the BC avoided from FBCR; there will be additional BC avoidance from all fossil fuels used in electricity, transportation, cooking sectors, and SBFs. Consideration of these would render much greater GHG savings. Similarly, for lack of appropriate data, the avoided particulate emissions due to coal, other petroleum fuels used in electricity generation and transportation, SBFs (cooking), and emissions from MSW burning were excluded. The inclusion of these would render better environmental performance of WTE schemes. This also requires a separate full LCA study.
In order to validate the results from this analysis, we have compared our results to those of other studies. This paper gives a lower value for India's total biomethane yields compared to Mittal et al.,28 despite considering a wider variety of sectors and individual elements in each sector (e.g., many more types of crops and industries). This may be attributable to the differences in data sources for production of crops and other products, the use of state-wise accessibility factors for crops and distinct experimental methane yields for all substances, which should represent the real-world scenario more closely. Moreover, our results are modest in that co-digestion can increase yields of biomethane, which has not been considered here, for reasons already mentioned in Section 2.1. The biomethane generation obtained in this paper is, however, much higher than the estimates of Rao et al.,27 as we included more sectors and there has been an increase in production and consumption in the decade since Rao et al. was published.
Comparing individual sectors, we found that our calculated biomethane yields (and, consequently, the electricity generation potential) of animal wastes (excluding slaughterhouses) are similar, but lower than those found by Kaur et al.30 (363 TWh from our calculations, as against 477 TWh). This difference is expected due to our detailed methodology, lower availability factors, and more recent data sources, as already explained in the Section 2.1.3.
This paper is the first study to quantify the achievable GHG abatement on using wastes as energy sources in India under different end-use scenarios, so there are no published studies available to cross-validate the end-use-specific results.
The calculated GHG emissions from biomethane production per MJ, in Section 3.2 is found to be about 3 times that obtained by Singh et al.119 (10.5 gCO2eq./MJ), due to the consideration of 3% biomethane leakage here, as opposed to 1% in the earlier study; these leakages constitute most of the emissions. The results on the final emissions of kerosene adapted from Garg et al.114 (169 gCO2eq./MJ) agree well with those adapted from Singh et al.119 (171 gCO2eq./MJ), but there is a small mismatch between the final emissions from LPG used in cooking as adapted from Garg et al.114 (130 gCO2eq./MJ), and those from Singh et al.119 (160 gCO2eq./MJ). We used adapted results from Garg et al.114 for both cases, to maintain consistency in the source. This also renders slightly more modest (and less optimistic) emission savings.
3.4. Comparisons with the state-of-the-art
The Indian Government has already launched the National Biogas and Manure management Programme (NBMM) and the National Project on Biogas Development (NPBD) to encourage electricity generation from biogas, and the generation of energy from municipal, industrial, and animal wastes.164 However, India generated only 32 TWh of electricity from biofuels and wastes in 2019;14i.e., 4.3% of biomethane's estimated potential. India's annual biogas generation was 2.07 billion m3 in 2018–19;165 less than 2% of the estimated potential. The total WTE installed capacity (including direct waste combustion for electricity) in India was 10.18 GW in 2021,161 which is roughly 12% of the annual potential estimated in this paper (a state-wise comparison is provided in Table 6). In 2018–19, the share of renewables (including hydro) in India's electricity generation was roughly 17%,3 which could be increased to 62% if all the waste-to-biomethane potential were harnessed. There was also negligible biofuel use in transportation in India in 2019.14 The transportation sector almost entirely uses fossil fuels, and the utilisation of biomethane could raise the share of renewables to 91% for the sector. These clearly denote the immense gap between the potential and the state-of-the-art in India and encourage policy formulations in favour of WTE generation.
3.5. Policy perspectives
This paper shows a clear incentive for investments and policies to support the conversion of wastes to biogas to reduce pollution and GHG emissions, given the potential, decentralised feedstock availability, and that biogas plants are economically viable and profitable in India.164,166 There is the added benefit of reducing dependency on imported energy (chiefly petroleum) and enhancing India's energy self-sufficiency. The social and economic costs of air pollution will also be eliminated.
WTE projects induce little or no encroachment upon agricultural lands (other than perhaps building some biogas plants); hence, the competition between growing crops for food and energy is largely avoided. The generation of biogas, which has an economic value, can also act as an incentive to collect, segregate, and treat wastes. There is scope for providing more rural employment and ensuring grassroots development and innovation.167 Allocating an economic value to wastes and setting up a market structure in which farmers can sell their crop residues and animal wastes, can generate additional income for farmers, while also preventing the open burning of these valuable energy resources. A country-wide economic assessment of biogas projects is needed to further highlight their economic potential.
There are several challenges for biogas projects: the high upfront investments,17 the timely availability and supply of appropriate feedstocks for mono or co-digestion for all biogas plants throughout the country, the efficient harvesting of crop wastes (especially with the lack of appropriate machinery with farmers), the lack of source segregation of MSW, and the considerable water requirements in biogas plants,168–170 are some major challenges. For electricity generation, the majority of existing power plants in the country use steam turbines for coal; while these can generate electricity from biomethane, the efficiencies are lower, at 40%.171 To exploit the higher efficiencies of CCGTs, these power plants will need retrofitting. A difficulty for the end uses as cooking and transportation fuels is that the domestic ovens and car engines of the masses will need technical modifications or replacements for using biomethane. Government policy support and large private investments are thus necessary. Furthermore, with the rising prices of petroleum products and cooking gas, the citizens might find it favourable to shift to using biomethane for their energy needs if it is cheaper and widely available.
A temporal assessment of waste availability (especially crop residues, which are seasonal and available only after harvests) is desired to design the biogas feedstock supply chains and plan storage effectively. For MSW, further investigation is needed into measures to incentivise and promote source segregation by citizens. The profits generated from biogas can encourage efficient MSW management, especially with the increase in organic content from 2005 levels (Section 2.1.4). To reduce fresh water demands, recirculating the liquid part of the digestate slurry after the solid portion has been removed (to be used as a fertiliser), can be helpful.47 In some cases, wastewater and solid wastes are available together, and the water requirement can be readily met, e.g., municipal solid wastes and wastewaters,172 or wastes in palm oil mills.173
The findings of this study allude to some policy debates. The huge biomethane potential denotes the importance of considering biogas projects for renewable and sustainable energy generation on an equal footing with solar and wind power in India, especially noting that biomethane can generate firm electric power, which can complement the intermittent solar and wind sources (that solar and wind are given importance is evident from the greater corresponding targets and policy support174). Also, biomethane provides greater flexibility for end-use and enables efficient waste management.
Presently, Indian policies encourage an increasing share of natural gas and LPG for transportation175 and cooking;176,177 however, Fig. 3 shows that biomethane can save substantial quantities of GHGs compared to these. This raises the debate on whether natural gas and LPG should be encouraged as transition fuels or at all, and whether undertaking large scale biogas projects could be a better move. Analysing the economics of countrywide biomethane projects will help to answer this, provide data on how replaceable the present-day fuels (especially cooking and transport) actually are, in terms of costs, and shed light on which of the three end-use scenarios discussed in this paper is more practical, despite biomethane-to-electricity giving the maximum GHG savings.
Our results raise another key question on whether heavily favouring electric vehicles (EVs) in India from 2030 onwards178 will be good for GHG emissions and whether encouraging biofuels in transportation would be better, given the high share of coal in electricity generation (at present) and the increased electricity demands that this move will entail. Of course, generating electricity from renewables would help, but the relative energy efficiencies (of using biomethane directly as a transport fuel versus using electricity generated from biomethane and other renewables to power EVs) and economics need further research and consideration.
A major present-day policy supporting biogas is for family-sized biogas plants for clean cooking fuel provisions in rural areas.165,174 Installing large industrial biogas plants for this is a better alternative when considering biomethane yields, since it is difficult to maintain suitable temperature and pH conditions in family-sized biogas plants, thus leading to lower biogas yields. Many family-sized biogas plant users have reported lower yields in winters and unpredictability of fuel generation, which forced them to resort back to SBFs.17,26Fig. 2 shows that nearly 26% of India's current cooking fuel demand is met by SBFs, coal and charcoal (electric cooking is only a meagre share of this179), so, generating biomethane from the wastes that are currently used as SBFs would reduce indoor air pollution and concomitant health issues, in addition to reducing GHGs. The availability factor of wastes for biomethane generation would also increase, accompanied by a reduction in the felling of trees to procure fuelwood. So, there needs to be considerations on how to replace the SBFs180,181 (that are free) and kerosene (which can be purchased daily in small quantities180) in rural areas with the comparatively (and potentially) expensive biogas generated industrially, without adversely affecting the poor populace and also preventing eventual fuel stacking177 due to economic constraints.180
The discussions in this paper show that the use of biomethane (from wastes) can help India in achieving six of the United Nations' (UN's) sustainable development goals: ‘affordable and clean energy’, ‘climate action’, ‘sustainable cities and communities’, ‘good health and wellbeing’, ‘clean water and sanitation’, and ‘responsible consumption and production’.182
4 Conclusions
This paper assesses and demonstrates the immense biomethane generation potential from all types of wastes produced in India. It creates a strong case for policy implementations that favour maximal biomethane generation and utilisation throughout the country. It includes all the states of India, involving every principal waste-producing sector, and is based on data published by the Government of India, reputable international organisations, and results from the academic literature. The characteristics of biodegradable wastes from agriculture, horticulture, animal husbandry, and those generated in various Indian cities are also compiled.
This paper represents the most comprehensive evaluation of India's WTE potential to date, explores the possible displacement of unsustainable and polluting fuels with this biomethane under three end-use scenarios, and estimates the corresponding avoided GHG and PM emissions from such WTE conversion. It is found that more than 125 billion m3 of biomethane could be produced annually from waste resources in India, which would be able to supply around 47% of India's present electricity generation. This could be an important step towards sustainability, as biomethane leads to significant reductions in GHG emissions when compared with India's existing grid electricity. The annual avoided GHG emissions from the electricity sector would be 461 million tonnes of CO2-equivalent (excluding the impacts of black carbon), accompanied by the removal of particulate emissions from fossil fuels and field burning of crop residues, appropriate waste management, reductions in air, water, and soil pollution, and improved energy access in remote areas.
Alternatively, the biomethane could be used to provide for all of India's cooking fuel needs (from only 43% of the generated methane) or 91% of India's fuel requirements for road transportation. The net avoided GHG emissions considering all the three end-use scenarios are between 284 and 461 million tonnes of CO2 equivalent annually (excluding BC). The emission savings of PM2.5 and PM10 combined, from the avoidance of FBCR alone, are between 1.8 and 2.1 million tonnes, and those of GHGs, between 37 million tonnes (excluding BC) and 101 million tonnes (including BC) of CO2 equivalent.
This paper denotes ample scope for improvement from the present-day harness of biogas, as discussed with numeric evidences. There are some additional findings relating to the share of SBFs in cooking fuels, an increase of organic content in MSW over the past 17 years, and the comparisons of the present-day cooking and road transport fuels with biomethane in terms of carbon intensities. Some research areas and policy debates for the future are also explored.
Biomethane from wastes is a renewable and sustainable energy resource. Six sustainable development goals of the UN that can be achieved from waste-to-biomethane generation in India are identified, and the energy potential estimated in this paper is compared with the state-of-the-art, stressing the importance of the uptake of WTE schemes in India and the need for government policy support and private investments. This paper thus emphasises and highlights the importance of large-scale WTE conversion, which can be supported by the provided datasets on biomethane generation for energy pathway planners, policy makers, and environmental scientists.
Appendices
Appendix 1: Data compilations
Agriculture and horticulture wastes.
Data for calculations involving agricultural and horticultural wastes are compiled in Table 8 below.
Table 8 Data for crop residues
Crop residue |
Residue to product ratio (RPR) |
Total solids content (TS, % of fresh matter) |
Volatile solids content (VSTS, % of TS) |
Net volatile solids (VS, % of fresh matter) |
Methane yield (BMP) Yb for mono-digestion (m3 per tonne-VS) |
References |
Physical pre-treatment (cutting and chopping/pulverisation, heating, or microwave) and/or chemical (alkaline) pre-treatment improves biomethane yields.50,165,183,187,238,257–259 The cases for chemical pre-treatment, which unmasks lignin and improves methane yields, are indicated in this table; physical pre-treatments like chopping and cutting is expected for most wastes. However, heat, ultrasound, and microwave treatment would incur energy expenditure and as such, were not included in this study.
Calculated using the basic formula as experimental results were not found.
The results on TS, VS, and BMP for oil palm fronds were reused for coconut and arecanut fronds.
The results for lentils were used for all other pulses in the absence of specific data.
The RPRs for arecanut fronds, coconut fronds, and cotton stalks are not pure ratios, but are expressed in t ha−1 (tonnes per hectare) of wastes generated.
|
Cereals
|
Rice straw (physical and/or chemical pre-treatment with NaOH or Ca(OH)2 or Na2CO3)a |
1.5 |
85.56 |
82.05 |
70.2 |
277 |
29, 31, 51, 183–192 |
Rice husk |
0.2 |
89.2 |
87 |
77.8 |
40 or 300 (used 300, because the higher yield was experimentally possible.) |
29, 31, 46, 51, 184, 185, 193 and 194 |
Wheat straw and cobs (pre-treatment) |
1.8 |
91.55 |
89.38 |
81.83 |
252 |
29, 31, 43, 183, 187, 195–203 |
Maize/corn stover |
2.3 |
90.5 |
95.56 |
86.48 |
364 |
29, 54, 183, 196, 204–206 |
Millets (nutri-cereals, except maize) |
1.55 |
91.48 |
94.43 |
86.38 |
293 |
29, 51, 54, 204, 207 and 208 |
![[thin space (1/6-em)]](https://www.rsc.org/images/entities/char_2009.gif) |
Horticulture (except orchards)
|
Aubergine/eggplant (brinjal) |
0.18 |
19.4 |
94.1 |
18.26 |
374 |
206 and 209–211 |
Carrot leaves |
0.45 |
14.8 |
83.6 |
12.37 |
312 |
194, 212 and 213 |
Potato |
0.81 |
18.2 |
94.6 |
17.22 |
250 |
54, 214 and 215 |
Sweet potato |
0.63 |
91.8 |
85.7 |
78.67 |
200.2 |
216 and 217 |
Tapioca/cassava |
0.75 |
30.92 |
94.64 |
29.26 |
270.3 |
54, 218 and 219 |
Okra (pre-treated with NaOH) |
0.4 |
15.15 |
47.87 |
7.25 |
369.09 |
220
|
Tomato |
0.44 |
10.25 |
78.24 |
8.02 |
240 |
205, 210, 215 and 221 |
Onion |
0.4 |
28.55 |
90.68 |
25.89 |
353 |
215
|
Cauliflower |
1.49 |
5.69 |
90.16 |
5.13 |
250 |
55, 222 and 223 |
Cabbage |
1.49 |
6.7 |
92.3 |
6.18 |
223.88 |
55, 214, 222 and 223 |
Coriander |
1.15 |
11.19 |
85.7 |
9.59 |
309 |
54, 209 and 223 |
Beans |
1.6 |
10 |
|
|
128 (m3 per tonne-waste; not VS) |
216 and 224 |
Cucumber |
0.2 |
10.77 |
74.7 |
8.05 |
280 |
210, 212 and 221 |
Radish |
0.45 |
5.76 |
90.1 |
5.19 |
272 |
212, 213 and 225 |
Capsicum |
0.45 |
20.35 |
80.64 |
16.41 |
120.6 |
210, 212 and 213 |
Chilli |
1.5 |
92.987 |
85.37 |
79.38 |
104.2 |
210 and 226 |
Ginger |
1.15 |
90 |
83.88 |
75.49 |
195b |
212 and 213 |
Pea |
1.1 |
90 |
83.88 |
75.49 |
195b |
206 and 212 |
Banana pseudostems and leaves (cutting and chopping) |
3 |
4.82 |
78.42 |
3.78 |
347 |
55 and 227–229 |
Turmeric |
0.3 (ginger) |
90 |
83.88 |
75.49 |
195b |
212 and 213 |
Cumin |
1.55 |
10 |
83.88 |
8.39 |
195b |
212
|
Cardamom |
0.64 |
90 |
83.88 |
75.49 |
195b |
212
|
Garlic |
0.2 |
10 |
83.88 |
8.39 |
195b |
212
|
Melons (watermelon and muskmelon) |
0.2 |
20 |
83.88 |
16.78 |
195b |
212
|
Arecanut frondc |
3e t ha−1 |
59.46 |
78.3 |
46.56 |
200 |
29 and 54 |
Arecanut husks |
0.8 |
91.12 |
79.87 |
72.78 |
91.12 |
29, 54, 230 and 231 |
Coconut frondc |
4e t ha−1 |
59.46 |
78.3 |
46.56 |
200 |
29 and 54 |
Coconut husk |
0.53 |
86.96 |
27.82 |
24.19 |
Not used |
29, 54 and 232 |
![[thin space (1/6-em)]](https://www.rsc.org/images/entities/char_2009.gif) |
Cash crops and plantation crops (availability factor for ‘others’
29
)
|
Tobacco |
2.95 |
92.86 |
93.79 |
87.09 |
175 without treatment, 312 (7% AHP treated, used here) |
50 and 233 |
Tea |
1.2 |
90.5 |
92 |
83.26 |
217 |
233–235
|
Coffee (pruning residues) |
See Table 9 |
91.3 |
82.15 |
75 |
109 (from orchard pruning wastes section) |
236 and 237 |
Sugarcane tops and leaves (alkaline pre-treatment will improve) |
0.13 |
76.7 |
86.3 |
66.19 |
228.33 (mean) |
206, 233 and 238 |
Sugarcane bagasse (alkaline pre-treatment will improve) (considered 80% sugarcane for this, corresponding to sugar production) |
0.3 |
55.4 |
96 |
53.18 |
281 (mean) |
206, 233 and 238 |
Sugarcane press mud |
0.036 |
27.7 |
80.8 |
22.38 |
198 |
239 and 240 |
Cotton stalk, boll shell, husks |
3.8e t ha−1, 1.1, 1.1, respectively |
85.57 |
94.72 |
81.05 |
148.74 |
29, 216 and 241 |
Jute stalk |
2 |
91.41 |
94.78 |
86.64 |
195a |
29, 242 and 243 |
Cocoa |
1.5 |
|
|
13 |
193 |
78 and 244 |
![[thin space (1/6-em)]](https://www.rsc.org/images/entities/char_2009.gif) |
Oilseeds and palm oil
|
Mustard and rapeseed |
1.8 |
87.97 |
89.05 |
78.34 |
132.3 |
29 and 49 |
Sunflower stalk |
3 |
94 |
93.61 |
88 |
192 |
29 and 245 |
Safflower stalk |
3 |
94 |
93.61 |
87.99 |
89 |
29 and 246 |
Soybean |
1.7 |
87.5 |
91 |
79.63 |
196 |
29, 194 and 247 |
Palm oil empty fruit bunches (EFB) |
0.22 |
43.6 |
89.91 |
39.2 |
358 |
112, 173 and 248–251 |
Oil palm fronds |
0.55 |
59.46 |
78.3 |
46.56 |
200 |
252–255
|
![[thin space (1/6-em)]](https://www.rsc.org/images/entities/char_2009.gif) |
Pulses (straws)
|
Gram |
1.1 |
65 |
88.77 |
57.7 |
279.2 |
29, 242 and 256 |
Lentil |
1.8 |
65 |
88.77 |
57.7 |
279.2 |
29, 242 and 256 |
Tur/Arhar |
2.5 |
65 |
88.77 |
57.7 |
279.2 |
29, 242 and 256 |
Orchard pruning wastes.
Data for calculations involving orchard pruning wastes are compiled in Table 9 below.
Table 9 Data for orchard pruning residues (biomethane generation = 109 m3 per tonne-FM (fresh matter))e
Fruit/nut |
Pruning wastes (tonne per hectare)62 |
RPR (calculated) |
Mean value.
Used the lowest value instead of the mean, as papaya plants are not expected to produce as much pruning residues as larger trees like mango and others, based on the authors' observations.
NR = Not required. These are not required as the fruits are not grown in India in appreciable quantities and hence not reported in the horticultural statistics; these are hence also not included in our assessment. The data were used only to obtain a mean value.
Coffee shrubs are usually smaller than the other fruit trees.
Note: Banana is included as a horticultural product in the previous Table 8.
|
Almond |
3 |
2.65 |
Aonla (Amla) |
3.8a |
0.33 |
Apple |
3.5 |
0.43 |
Grapes |
4.5 |
0.21 |
Guava |
3.8a |
0.25 |
Lime and lemon |
2.1 |
0.21 |
Mango |
3.8a |
0.42 |
Orange |
2.1 |
0.19 |
Papaya |
2.1b |
0.05 |
Pineapple |
3.8a |
0.22 |
Pomegranate |
3.8a |
0.32 |
Sapota/sapodilla |
3.8a |
0.31 |
Strawberry |
3.8a |
0.5 |
Sweet orange |
2.1 |
0.13 |
Walnut |
3 |
1.15 |
Cashew |
3 |
3.9 |
Tamarind |
3.8a |
0.91 |
Olivesc |
1.8 |
NR |
Peachc |
6 |
NR |
Pearc |
8 |
NR |
Cherryc |
4 |
NR |
Apricotc |
5.5 |
NR |
Hazelnutc |
3 |
NR |
Coffee (not used to obtain meand) |
17 (ref. 260) |
NR |
Mean |
3.8 |
NR |
Animal wastes.
Data for calculations involving animal wastes are compiled in Table 10 below.
Table 10 Data for animal wastes
Species |
Mean live weight (kg) |
Wastes per animal per day (% of body weight) |
Availability (%) |
Total solids (TS) as a % of fresh matter |
Volatile solids (VS) as a % of fresh matter |
Biochemical methane potential (BMP, m3 per tonne-FM) |
References |
Same parameters as cattle, other than live weight.
Same parameters as buffalo.
|
Cattle |
336 |
6 |
70 |
18.67 |
14.33 |
29 |
30, 43, 50, 53, 84, 205 and 261–263 |
Buffaloa |
440 |
6 |
70 |
18.67 |
14.33 |
29 |
30, 43, 50, 53, 84, 205 and 261–263 |
Yak and mithunb |
440 |
6 |
50 |
18.67 |
14.33 |
29 |
|
Goat |
34 |
4 |
13 |
59.22 |
47.55 |
73 |
30, 37, 73, 74, 76, 81–84 and 262–265 |
Sheep |
31 |
4 |
13 |
34.51 |
29.465 |
63 |
30, 37, 73, 74, 76, 81–83 and 261–265 |
Pig |
80 |
4 |
80 |
27.8 |
22.89 |
82 |
50, 261 and 262 |
Horse |
268 |
6 (same as cattle) |
50 |
29.79 |
25.77 |
52 |
84, 262, 264 and 266 |
Donkey (same as horse, except live weight) |
160 |
6 |
50 |
29.79 |
25.77 |
52 |
262, 264, 266–268 |
Mules (same as horse) |
268 |
6 |
50 |
29.79 |
25.77 |
52 |
262, 264 and 266–268 |
Camel |
570 |
6 (same as cattle) |
50 |
36 |
21.57 |
35.8 |
84, 263, 269 and 270 |
Poultry |
1.5 (for manures) – 1.7 (for slaughterhouse) |
3 |
90 |
49.16 |
37.27 |
109 |
30, 37, 43, 50, 71–74, 76, 86, 261, 262 and 271 |
India's municipal solid wastes.
Data on the characteristics of MSW in India are compiled in Table 11 below.
Table 11 Characteristics of MSW in Indian cities
City |
State/UT |
Biodegradable fraction (except paper and textile) |
TS (%) |
VS (% of total wastes) |
References |
Agartala |
Tripura |
55.43 |
63.69 |
NR |
272
|
Agra |
Uttar Pradesh |
46.38 |
72 |
NR |
11, 87, 88 and 273 |
Ahmedabad |
Gujarat |
44 |
68 |
NR |
11, 87, 88, 274 and 275 |
Aizwal |
Mizoram |
49 |
57 |
NR |
88 and 276 |
Allahabad |
Uttar Pradesh |
45.3 |
74.14 |
NR |
277
|
Amritsar |
Punjab |
65.05 |
39 |
NR |
11 and 88 |
Asansol |
West Bengal |
47.24 |
56 |
NR |
88 and 278 |
Bangalore |
Karnataka |
81.96 |
45 |
NR |
16 and 88 |
Bhopal |
Madhya Pradesh |
69 |
NR |
46.6 |
279
|
Bhubaneswar |
Odisha |
49.81 |
41 |
NR |
88
|
Chandigarh |
Chandigarh |
52 |
54.22 |
NR |
280 and 281 |
Chennai |
Tamil Nadu |
55 |
45 |
50 |
172 and 282 |
Coimbatore |
Tamil Nadu |
63.24 |
43 |
NR |
11, 87, 88 and 283 |
Cuttack |
Odisha |
50 |
30 |
NR |
284
|
Daman |
Daman and Diu |
29.6 |
47 |
NR |
88
|
Delhi |
Delhi |
73 |
59.4 |
28.2 |
95 and 285–287 |
Dehradun |
Uttarakhand |
80 |
40 |
NR |
88 and 288 |
Dhanbad |
Jharkhand |
75 |
51.6 |
NR |
289
|
Faridabad |
Haryana |
42 |
66 |
NR |
11, 88 and 290 |
Gandhinagar |
Gujarat |
34.3 |
76 |
NR |
88
|
Gangtok |
Sikkim |
52.5 |
66 |
NR |
88 and 276 |
Guwahati |
Assam |
45.1 |
80 |
NR |
88, 276 and 291 |
Gwalior |
Madhya Pradesh |
55.2 |
48.96 |
NR |
292
|
Hardwar |
Uttarakhand |
49.1 |
85.74 |
NR |
293
|
Hyderabad |
Telengana |
69.9 |
54 |
NR |
87, 88 and 294 |
Imphal |
Manipur |
53.28 |
60 |
NR |
88 and 276 |
Indore |
Madhya Pradesh |
43 |
15 |
88.5 |
88, 90 and 295 |
Itanagar |
Arunachal Pradesh |
53.81 |
50 |
NR |
88 and 276 |
Jabalpur |
Madhya Pradesh |
58.07 |
65 |
NR |
11, 87 and 88 |
Jaipur |
Rajasthan |
45 |
60 |
NR |
87, 88 and 280 |
Jalandhar |
Punjab |
37 |
70 |
20.4 |
296
|
Jamshedpur |
Jharkhand |
64 |
69 |
NR |
11, 87 and 88 |
Jorhat |
Assam |
57.1 |
54.91 |
NR |
88 and 276 |
Kanpur |
Uttar Pradesh |
27 |
54 |
NR |
11, 87, 88 and 297 |
Karavatti |
Lakshadweep |
46.01 |
75 |
NR |
88
|
Kochi |
Kerala |
|
58.39 |
19.63 |
88 and 291 |
Kolkata |
West Bengal |
79.8 |
50 |
NR |
122 and 298 |
Kohima |
Nagaland |
55.1 |
54 |
NR |
11, 87 and 88 |
Lucknow |
Uttar Pradesh |
54.86 |
35 |
NR |
87, 88 and 299 |
Ludhiana |
Punjab |
40 |
40 |
NR |
11, 87 and 88 |
Madurai |
Tamil Nadu |
49.8 |
35 |
NR |
11, 87 and 88 |
Meerut |
Uttar Pradesh |
55.32 |
54 |
NR |
11, 87 and 88 |
Mumbai |
Maharashtra |
54.54 |
68 |
NR |
87, 88, 300 and 301 |
Nagpur |
Maharashtra |
42 |
46 |
NR |
11, 87, 88 and 291 |
Nainital |
Uttarakhand |
77.2 |
59 |
NR |
88 and 276 |
Nashik |
Maharashtra |
52.76 |
0 |
NR |
11, 87, 88 and 302 |
Panjim |
Goa |
81.6 |
38 |
NR |
88
|
Patna |
Bihar |
53.95 |
53 |
NR |
87, 88, 94 and 303 |
Pimpri-chinchwad |
Maharashtra |
54.32 |
64 |
NR |
87 and 304 |
Port Blair |
Andaman and Nicobar |
32 |
52 |
NR |
88
|
Puducherry |
Puducherry |
48.25 |
37 |
NR |
280
|
Pune |
Maharashtra |
50 |
46 |
NR |
305
|
Raipur |
Chhattisgarh |
69.3 |
70.28 |
62.61 |
11, 87 and 88 |
Rajkot |
Gujarat |
51.4 |
71 |
NR |
11, 87 and 88 |
Ranchi |
Bihar |
41.5 |
83 |
NR |
11, 87 and 88 |
Roorkee |
Uttarakhand |
51.49 |
51 |
NR |
306
|
Sangamner |
Maharashtra |
39.53 |
86.57 |
NR |
307
|
Shillong |
Meghalaya |
42.22 |
61.5 |
NR |
88 and 276 |
Silvassa |
Dadra and Nagar Haveli |
62.54 |
37 |
NR |
88
|
Simla |
Himachal Pradesh |
71.67 |
58 |
NR |
88 and 276 |
Srinagar |
Jammu and Kashmir |
53.01 |
40 |
NR |
87, 88 and 308 |
Surat |
Gujarat |
54.65 |
39 |
NR |
11, 87 and 88 |
Thane |
Maharashtra |
56.87 |
49 |
NR |
11, 87 and 88 |
Tiruvananthapuram |
Kerala |
42 |
49 |
NR |
88
|
Tura |
Meghalaya |
72.96 |
40 |
NR |
87 and 88 |
Vadodara |
Gujarat |
54.86 |
37 |
NR |
88 and 309 |
Varanasi |
Uttar Pradesh |
60.47 |
52 |
NR |
287
|
Vijayawada |
Andhra Pradesh |
46.13 |
65.28 |
NR |
11, 87 and 88 |
Visakhapatnam |
Andhra Pradesh |
59.43 |
54 |
NR |
11, 87 and 88 |
Mean values
|
|
53.93
|
54.51
|
|
|
Appendix 2
The IPCC default methodology for estimation of methane emissions from MSW is based on the following basic equation:121,159,160,310 | MCH4 = (MSWT × MSWF) × MCF × DOC × DOCF × F × {(16/12) − R} × (1 − OX) × (CF) | (5) |
Here, MCH4 is the annual methane emission from India's MSW (in tonne per year). MSWT is the total quantity of MSW generated in India. MSWF is the fraction of MSW disposed of in open dumps, which has been considered to be 80% of the wastes produced in the urban areas (as published papers suggest that this value is between 72% and 90%11,15). MCF is the methane correction factor, whose value is 0.5 for landfills and 0.25 for open dumpsites;123 the latter is useful for our paper. DOC is the degradable organic carbon; its value can be estimated if the composition of wastes is roughly known (eqn (6)). DOCF is the fraction of organic carbon undergoing decomposition to yield methane, the value of which, has been suggested to be 0.77 for India's countrywide estimate (for a temperature of 35 °C).159,160R is the volume of recovered landfill gas, which is zero for the present case, as landfill gas as an energy source is not being considered here (and such capture is not prevalent in India159), and the gas is assumed to escape into the atmosphere. OX is the oxidation factor, with a default value of zero. The term CF denotes a correction factor introduced by us to provide more realistic estimates of the methane emissions from landfills and avoid overly optimistic estimates of the displaced GHG emissions of biogas production and use. Multiple field studies160,311–313 suggest that the actual methane emissions from a landfill are 2 to 7 times lower than the value estimated by the IPCC default methodology. So, in order to ensure that the results of this analysis are conservative, the value of CF has been assumed to be (1/4.5). This correction factor brings the estimated values of methane emissions close to the results of the first order decay method, as can be observed from the results of Ghosh et al.160, and prevent an overly optimistic result. | DOC = (0.4 × A) + (0.17 × B) + (0.15 × C) + (0.3 × D) | (6) |
Here, A = fraction of wastes like textile, paper, and cardboard, B = fraction of organic wastes from gardens, parks, road sweepings, and other sources, except food. C = fraction of biodegradable food wastes. D = fraction of wood wastes.
To calculate DOC, data from the literature on waste compositions in multiple Indian cities were used (compiled in Table 12). In some cases, the classification of the wastes was not exactly as described above. In cases where only ‘biodegradable wastes’ or ‘compostable wastes’ was mentioned, this entire fraction was categorised under food wastes. This is a potential source of error as some wood or garden wastes may get included in food wastes. However, this is not a major reason for concern as this was only for very few cases among 49 cities. Moreover, the coefficients of B, and C are not very different, hence the introduced error will be small. Paper and textiles were always found to be reported separately. The data are summarised in Table 12. Finally, the mean value of DOC was calculated (=0.1224) for India, which was then used to obtain the total methane emissions (=4.49 million tonnes of CO2-equivalent; 20.21 million tonnes without using our introduced correction factor).
Table 12 Data for DOC calculations
City |
Paper, cardboard, textile (A) |
Garden, park, other (B) |
Food waste (C) |
Wood (D) |
DOC (calculated) |
References |
Agartala |
10 |
|
55.43 |
|
0.1231 |
272
|
Agra |
1.5 |
8 |
37 |
4.6 |
0.0889 |
273
|
Ahmedabad |
3 |
4 |
40 |
|
0.0788 |
274 and 275 |
Aizwal |
9.4 |
|
49 |
|
0.1111 |
276
|
Allahabad |
6.91 |
|
45.3 |
|
0.0956 |
277
|
Asansol |
9.59 |
32.25 |
8 |
6.99 |
0.1262 |
278
|
Bangalore |
12.61 |
|
72 |
|
0.1584 |
16
|
Bhopal |
12.06 |
22.15 |
43.18 |
0.5 |
0.1522 |
279
|
Chandigarh |
7.7 |
|
52 |
|
0.1088 |
280 and 281 |
Chennai |
15 |
26 |
15 |
14 |
0.1687 |
282
|
Coimbatore |
11.225 |
3.827 |
56.505 |
1.924 |
0.1419 |
283
|
Cuttack |
3 |
1 |
49 |
|
0.0872 |
284
|
Delhi |
17.93 |
15.83 |
56.3 |
|
0.1831 |
285
|
Dehradun |
8 |
|
80 |
|
0.152 |
288
|
Dhanbad |
3 |
|
75 |
|
0.1245 |
289
|
Faridabad |
7.16 |
|
42 |
|
0.0916 |
290
|
Gangtok |
10 |
|
52.5 |
|
0.1188 |
276
|
Guwahati |
21.35 |
5.25 |
37.4 |
2.45 |
0.1578 |
291
|
Gwalior |
13.8 |
|
55.2 |
2.54 |
0.1456 |
292
|
Haridwar |
4.5 |
27.325 |
21.77 |
|
0.0971 |
293
|
Hyderabad |
17.4 |
23 |
37.3 |
1.1 |
0.168 |
294
|
Imphal |
6.72 |
|
53.28 |
|
0.1068 |
276
|
Indore |
7 |
|
43 |
|
0.0925 |
295
|
Itanagar |
8.39 |
|
53.81 |
|
0.1143 |
276
|
Jaipur |
8 |
|
45 |
|
0.0995 |
280
|
Jalandhar |
15.5 |
|
35 |
2 |
0.1205 |
296
|
Jorhat |
6.4 |
|
57.1 |
|
0.1113 |
276
|
Kanpur |
3 |
|
12 |
14 |
0.072 |
297
|
Kochi |
4.9 |
|
79.8 |
|
0.1393 |
291
|
Kolkata |
8 |
5 |
45.5 |
1.2 |
0.1124 |
122 and 298 |
Kohima |
7.64 |
|
54.86 |
|
0.1129 |
276
|
Lucknow |
6.4 |
|
40 |
|
0.0856 |
299
|
Mumbai |
21.8 |
6.3 |
35.7 |
|
0.1515 |
300 and 301 |
Nagpur |
9.6 |
|
77.2 |
|
0.1542 |
291
|
Nainital |
11.74 |
|
52.76 |
|
0.1261 |
276
|
Nashik |
6.8 |
24.5 |
54 |
3.1 |
0.1592 |
302
|
Panjim |
0.92 |
1.31 |
52.6 |
0.04 |
0.0849 |
94
|
Patna |
4.58 |
7.735 |
45.94 |
0.645 |
0.1023 |
303
|
Pimpri-chinchwad |
12.17 |
|
33.5 |
11.03 |
0.132 |
304
|
Puducherry |
15 |
|
50 |
|
0.135 |
280
|
Pune |
14.7 |
|
69.3 |
|
0.1628 |
305
|
Roorkee |
12.78 |
22.5 |
17.03 |
|
0.1149 |
306
|
Sangamner |
8.6 |
12.22 |
20.5 |
9.5 |
0.1144 |
307
|
Shillong |
9.46 |
|
62.54 |
|
0.1317 |
276
|
Simla |
7.99 |
|
53.01 |
|
0.1115 |
276
|
Srinagar |
5.77 |
3.7 |
49.7 |
1.25 |
0.1077 |
308
|
Tura |
8.64 |
|
54.86 |
|
0.1169 |
276
|
Vadodara |
7.39 |
0.4 |
60.07 |
|
0.1203 |
309
|
Varanasi |
12.54 |
|
46.13 |
|
0.1194 |
287
|
There are other sources of error in this calculation. First, the generation of methane from wastes depends on the ambient temperature (and rainfall, which decides the moisture in the wastes), the impacts of which have not been accounted for. The temperature of 35 °C, though reasonable for most of India in summer, is not a good assumption for mountainous areas and will lead to overestimations in winters and monsoons. The impact of rainfall, though not explicitly applicable in the IPCC default method, appears as a factor in the LANDGEM procedure,122 which has not been used here. This is because the chief aim of the present paper is not to calculate methane emissions from MSW with high accuracy but to only obtain an approximate estimate of the avoided GHG emissions. Estimating the methane emissions from MSW for the whole country requires separate considerations of the various climatic zones in India, and the seasonal variations of the compositions of wastes in various cities. We suggest this as a separate study.
The correction factor introduced by us is another source of error, as its exact value cannot be ascertained and could depend on the age of the garbage dump being considered.160,313 The default methodology assumes that the same amounts of wastes are deposited yearly in the dumps and that methane emissions are constant every year.159 It is independent of the age of the dump, which is not ideal, but is well suited to our study since we deal with waste quantities in the entire country and not individual dumps or cities. This was corrected for by the stated correction factor.
Another source of uncertainty is the assumption that 80% of the wastes generated are disposed of in open dumpsites. However, there are no better ways of accurately quantifying this and the present assumption is expected to yield a reasonably good estimate, based on literature review. In Singh et al.,159 70% of the wastes generated in India have been assumed to be landfilled. From literature survey,10,11,15,160 80% open dumping seems more plausible. Despite these uncertainties, the eqn (5) and (6) are expected to give a useable estimate of methane generation from open municipal dumpsites in India as it is based on a dependable IPCC methodology and a comprehensive literature review.
Abbreviations and acronyms
AD | Anaerobic digestion |
AF | Accessibility/availability factor |
BC | Black carbon |
BMP | Biochemical methane potential |
CCGT | Combined cycle gas turbine |
CNG | Compressed natural gas |
CPCB | Central Pollution Control Board |
EF(s) | Emission factor(s) |
EV(s) | Electric vehicle(s) |
FAO | Food and Agriculture Organisation of the United Nations |
FBCR | Field burning of crop residues |
FM | Fresh matter |
GHG(s) | Greenhouse gas(es) |
GWP | Global warming potential |
HRT | Hydraulic retention time |
IEA | International Energy Agency |
IPCC | Intergovernmental Panel on Climate Change |
LCA | Life cycle assessment |
LCV | Lower calorific value |
LPG | Liquified petroleum gas |
MSW | Municipal solid wastes |
PM | Particulate matter |
PNG | Piped natural gas |
RCR | Residue-to-crop ratio |
RPR | Residue-to-product ratio |
SBF(s) | Solid biomass fuel(s) |
SRP | Surplus residue production |
TRP | Total residue production |
TS | Total solids (content) |
VS | Volatile solids (content) |
WTE | Waste-to-energy |
kWh | Kilowatt-hours |
TWh | Terawatt-hours |
Author contributions
Dey: conceptualisation, methodology, validation, formal analysis, investigation, writing – original draft, visualisation, funding acquisition. Thomson: conceptualisation, writing – review & editing, supervision.
Conflicts of interest
There are no conflicts to declare.
Acknowledgements
This paper presents unfunded research, building upon previous work that was carried out during Dey's MSc at The University of Edinburgh. The authors gratefully acknowledge the support of the Commonwealth Scholarship Commission in the United Kingdom for providing funding to Dey through the award of a Commonwealth Master's Scholarship for the MSc, which led to the development of this work.
References
-
The World Bank, Population, total – India, https://data.worldbank.org/indicator/SP.POP.TOTL?locations=IN Search PubMed.
- J. Karstensen, J. Roy, B. Deb Pal, G. Peters and R. Andrew, Key drivers of Indian greenhouse gas emissions, Econ. Polit. Wkly, 2020, 55, 46–53 Search PubMed.
-
Government of India, Energy Statistics India 2021, New Delhi, India, 2021 Search PubMed.
-
N. N. Dalei and A. Gupta, in Energy, Environment and Globalization: Recent Trends, Opportunities and Challenges in India, ed. A. Gupta and N. N. Dalei, Springer Nature Singapore Pvt Ltd., Singapore, 1st edn, 2020, pp. 17–33 Search PubMed.
- A. P. Heynen, P. A. Lant, S. Smart, S. Sridharan and C. Greig, Off-grid opportunities and threats in the wake of India’s electrification push, Energy Sustain. Soc., 2019, 9, 16 CrossRef.
- Y. Malakar, Evaluating the role of rural electrification in expanding people's capabilities in India, Energy Policy, 2018, 114, 492–498 CrossRef.
-
L. Srivastava, in International Petroleum Week, Energy Institute, London, UK, 2020 Search PubMed.
-
A. Aggarwal, A. Kumar and T. Joji Rao, in Energy, Environment and Globalization: Recent Trends, Opportunities and Challenges in India, ed. A. Gupta and N. N. Dalei, Springer Nature Singapore Pte Ltd., Singapore, 1st edn, 2020, pp. 117–126 Search PubMed.
-
Government of India, Generation of Solid Waste, Lok Sabha Unstarred Question No. 2974, 2018 Search PubMed.
- S. Kumar, S. R. Smith, G. Fowler, C. Velis, S. J. Kumar, S. Arya, Rena, R. Kumar and C. Cheeseman, Challenges and opportunities associated with waste management in India, R. Soc. Open Sci., 2017, 4(3), 160764 CrossRef PubMed.
- A. Kumar and A. Agrawal, Recent trends in solid waste management status, challenges, and potential for the future Indian cities – A review, Curr. Opin. Environ. Sustain., 2020, 2, 100011 CrossRef.
-
IEA, CO2 Emissions from Fuel Combustion, https://energyatlas.iea.org/#!/tellmap/1378539487 Search PubMed.
- S. Byravan, M. S. Ali, M. R. Ananthakumar, N. Goyal, A. Kanudia, P. V. Ramamurthi, S. Srinivasan and A. L. Paladugula, Quality of life for all: A sustainable development framework for India's climate policy reduces greenhouse gas emissions, Energy Sustain. Dev., 2017, 39, 48–58 CrossRef.
-
IEA, Data and statistics, https://www.iea.org/data-and-statistics?country=INDIA%26fuel=Energysupply%26indicator=ElecGenByFuel Search PubMed.
- K. D. Sharma and S. Jain, Overview of Municipal Solid Waste Generation, Composition, and Management in India, J. Environ. Eng., 2019, 145, 04018143 CrossRef.
- T. v. Ramachandra, H. A. Bharath, G. Kulkarni and S. S. Han, Municipal solid waste: Generation, composition and GHG emissions in Bangalore, India, Renew. Sustain. Energy Rev., 2018, 82, 1122–1136 CrossRef.
- S. Mittal, E. O. Ahlgren and P. R. Shukla, Barriers to biogas dissemination in India: A review, Energy Policy, 2018, 112, 361–370 CrossRef CAS.
- R. Joshi and S. Ahmed, Status and challenges of municipal solid waste management in India: A review, Cogent Environ. Sci., 2016, 2, 1–18 Search PubMed.
- S. Devi, C. Gupta, S. L. Jat and M. S. Parmar, Crop residue recycling for economic and environmental sustainability: The case of India, Open Agric., 2017, 2, 486–494 CrossRef.
- D. H. Cusworth, L. J. Mickley, M. P. Sulprizio, T. Liu, M. E. Marlier, R. S. Defries, S. K. Guttikunda and P. Gupta, Quantifying the influence of agricultural fires in northwest India on urban air pollution in Delhi, India, Environ. Res. Lett., 2018, 13(4), 044018 CrossRef.
- V. P. Kanawade, A. K. Srivastava, K. Ram, E. Asmi, V. Vakkari, V. K. Soni, V. Varaprasad and C. Sarangi, What caused severe air pollution episode of November 2016 in New Delhi?, Atmos. Environ., 2020, 222, 117125 CrossRef CAS.
- A. S. Nagpure, B. R. Gurjar and J. C. Martel, Human health risks in national capital territory of Delhi due to air pollution, Atmos. Pollut. Res., 2014, 5, 371–380 CrossRef CAS.
- S. Bhuvaneshwari, H. Hettiarachchi and J. N. Meegoda, Crop residue burning in India: Policy challenges and potential solutions, Int. J. Environ. Res. Public Health, 2019, 16(5), 832 CrossRef CAS PubMed.
- S. Sarkar, R. P. Singh and A. Chauhan, Crop Residue Burning in Northern India: Increasing Threat to Greater India, J. Geophys. Res., 2018, 123, 6920–6934 CrossRef.
- G. K. Porichha, Y. Hu, K. T. V. Rao and C. C. Xu, Crop Residue Management in India: Stubble Burning vs. Other Utilizations including Bioenergy, Energies, 2021, 14, 1–17 CrossRef.
- K. Ravindra, M. Kaur-Sidhu, S. Mor and S. John, Trend in household energy consumption pattern in India: A case study on the influence of socio-cultural factors for the choice of clean fuel use, J. Clean Prod., 2019, 213, 1024–1034 CrossRef.
- P. V. Rao, S. S. Baral, R. Dey and S. Mutnuri, Biogas generation potential by anaerobic digestion for sustainable energy development in India, Renew. Sust. Energ. Rev., 2010, 14(7), 2086–2094 CrossRef CAS.
- S. Mittal, E. O. Ahlgren and P. R. Shukla, Future biogas resource potential in India: A bottom-up analysis, Renew. Energy, 2019, 141, 379–389 CrossRef.
- M. Hiloidhari, D. Das and D. C. Baruah, Bioenergy potential from crop residue biomass in India, Renew. Sust. Energ. Rev., 2014, 32, 504–512 CrossRef.
- G. Kaur, Y. S. Brar and D. P. Kothari, Potential of livestock generated biomass: Untapped energy source in India, Energies, 2017, 10, 1–15 Search PubMed.
- R. Chandra, H. Takeuchi and T. Hasegawa, Methane production from lignocellulosic agricultural crop wastes: A review in context to second generation of biofuel production, Renew. Sust. Energ. Rev., 2012, 16, 1462–1476 CrossRef CAS.
- H. Khandelwal, A. K. Thalla, S. Kumar and R. Kumar, Life cycle assessment of municipal solid waste management options for India, Bioresour. Technol., 2019, 288, 121515 CrossRef CAS PubMed.
- C. Iordan, C. Lausselet and F. Cherubini, Life-cycle assessment of a biogas power plant with application of different climate metrics and inclusion of near-term climate forcers, J. Environ. Manage., 2016, 184, 517–527 CrossRef CAS PubMed.
- M. Liikanen, J. Havukainen, E. Viana and M. Horttanainen, Steps towards more environmentally sustainable municipal solid waste management – A life cycle assessment study of São Paulo, Brazil, J. Clean Prod., 2018, 196, 150–162 CrossRef.
- M. Poeschl, S. Ward and P. Owende, Environmental impacts of biogas deployment – Part I: Life Cycle Inventory for evaluation of production process emissions to air, J. Clean Prod., 2012, 24, 168–183 CrossRef CAS.
- A. Yasar, R. Rasheed, A. B. Tabinda, A. Tahir and F. Sarwar, Life cycle assessment of a medium commercial scale biogas plant and nutritional assessment of effluent slurry, Renew. Sust. Energ. Rev., 2017, 67, 364–371 CrossRef CAS.
- H. Afazeli, A. Jafari, S. Rafiee and M. Nosrati, An investigation of biogas production potential from livestock and slaughterhouse wastes, Renew. Sust. Energ. Rev., 2014, 34, 380–386 CrossRef.
- F. Tambone, B. Scaglia, G. D'Imporzano, A. Schievano, V. Orzi, S. Salati and F. Adani, Assessing amendment and fertilizing properties of digestates from anaerobic digestion through a comparative study with digested sludge and compost, Chemosphere, 2010, 81, 577–583 CrossRef CAS PubMed.
-
J. W. Twidell and A. D. Weir, Renewable Energy Resources, E & FP Spon, Tailor and Francis Group, London, UK, 3rd edn, 2000 Search PubMed.
-
D. Morris and J. Scurlock, in Renewable Energy – Power for a Sustainable Future, ed. G. Boyle, Oxford University Press, New Delhi, India, 3rd edn, 2016, pp. 117–168 Search PubMed.
-
S. Koonaphapdeelert, P. Aggarangsi and J. Moran, Biomethane: Production and Applications, Springer Nature Singapore Pte Ltd., Singapore, 1st edn, 2020 Search PubMed.
- S. Sarker, J. J. Lamb, D. R. Hjelme and K. M. Lien, Overview of recent progress towards in situ biogas upgradation techniques, Fuel, 2018, 226, 686–697 CrossRef CAS.
-
J. W. A. Langeveld and E. C. Peterson, in Biogas: Fundamentals, Operations and Processes, ed. H. Ghanavati and M. Tabatabaei, Springer International Publishing, Cham, Switzerland, 1st edn, 2018, pp. 35–49 Search PubMed.
-
B. Drosg, R. Braun, G. Bochmann and T. al Saedi, in The Biogas Handbook: Science, Production and Applications, ed. A. Wellinger, J. Murphy and D. Baxter, Woodhead Publishing, Elsevier Ltd., 2013, pp. 52–84 Search PubMed.
-
L. Mauer and R. Bradley Jr, in Food Analysis, ed. S. S. Nielsen, Springer International Publishing, Cham, Switzerland, 5th edn, 2019 Search PubMed.
- M. M. Rahman and J. v. Paatero, A methodological approach for assessing potential of sustainable agricultural residues for electricity generation: South Asian perspective, Biomass Bioenergy, 2012, 47, 153–163 CrossRef.
-
L. Talia, in Biogas: Fundamentals, Operations and Processes, ed. H. Ghanavati and M. Tabatabaei, Springer International Publishing, Cham, Switzerland, 1st edn, 2018, pp. 51–93 Search PubMed.
- P. Weiland, Production and energetic use of biogas from energy crops and wastes in Germany, Appl. Biochem. Biotechnol., 2003, 109, 263–274 CrossRef CAS PubMed.
- C. Wang, F. Hong, Y. Lu, X. Li and H. Liu, Improved biogas production and biodegradation of oilseed rape straw by using kitchen waste and duck droppings as co-substrates in two-phase anaerobic digestion, PLoS One, 2017, 12, 1–19 CAS.
- L. Wang, H. Zhang, Z. Dai, Y. Liu, C. Chen and G. Liu, Effect of nicotine inhibition on anaerobic digestion and the co-digestion performance of tobacco stalks with different animal manures, Process Saf. Environ. Prot., 2021, 146, 377–382 CrossRef CAS.
- J. Singh, Management of the agricultural biomass on decentralized basis for producing sustainable power in India, J. Clean Prod., 2017, 142, 3985–4000 CrossRef.
- M. Łochyńska and J. Frankowski, The biogas production potential from silkworm waste, Waste Manage., 2018, 79, 564–570 CrossRef PubMed.
- N. H. Ravindranath, H. I. Somashekar, M. S. Nagaraja, P. Sudha, G. Sangeetha, S. C. Bhattacharya and P. Abdul Salam, Assessment of sustainable non-plantation biomass resources potential for energy in India, Biomass Bioenergy, 2005, 29, 178–190 CrossRef.
-
R. A. Usmani, in Biofuels and Bioenergy (BICE2016): International Conference, Bhopal, India, 23–25 February 2016, ed. S. Suresh, A. Kumar, A. Shukla, R. Singh and C. M. Krishna, Springer Nature, Cham, Switzerland, 1st edn, 2017, pp. 67–82 Search PubMed.
- D. Cardoen, P. Joshi, L. Diels, P. M. Sarma and D. Pant, Agriculture biomass in India: Part 1. Estimation and characterization, Resour. Conserv. Recycl., 2015, 102, 39–48 CrossRef.
- H. Dhar, S. Kumar and R. Kumar, A review on organic waste to energy systems in India, Bioresour. Technol., 2017, 245, 1229–1237 CrossRef CAS PubMed.
-
Government of India, Agricultural Statistics at a Glance 2019, 2019 Search PubMed.
-
Government of India, Agricultural Statistics at a Glance 2020, New Delhi, India, 2021 Search PubMed.
-
Government of India, Horticultural Statistics at a Glance 2018, 2018 Search PubMed.
-
Tea Board India, State wise and Month wise Production data for the year 2017-18, Kolkata, 2018 Search PubMed.
-
Coffee board of India, Database on coffee, 2021 Search PubMed.
- J. den Boer, A. Dyjakon, E. den Boer, D. García-Galindo, T. Bosona and G. Gebresenbet, Life-cycle assessment of the use of peach pruning residues for electricity generation, Energies, 2020, 13, 1–16 CrossRef.
- N. Scarlat, V. Blujdea and J. F. Dallemand, Assessment of the availability of agricultural and forest residues for bioenergy production in Romania, Biomass Bioenergy, 2011, 35, 1995–2005 CrossRef.
- K. Moustakas, P. Parmaxidou and S. Vakalis, Anaerobic digestion for energy production from agricultural biomass waste in Greece: Capacity assessment for the region of Thessaly, Energy, 2020, 191, 116556 CrossRef.
- K. Moustakas, D. Sotiropoulos and S. Vakalis, Evaluation of the biogas potential of agricultural biomass waste for energy applications in Greece: A case study of the western Greece region, Waste Manag. Res., 2021, 39, 438–447 CrossRef CAS PubMed.
- C. di Blasi, V. Tanzi and M. Lanzetta, A Study On The Production Of Agricultural Residues In Italy, Biomass Bioenergy, 1997, 12, 321–331 CrossRef.
- L. Bianchini, P. Costa, P. P. Dell’omo, A. Colantoni, M. Cecchini and D. Monarca, An industrial scale, mechanical process for improving pellet quality and biogas production from Hazelnut and Olive pruning, Energies, 2021, 14, 1–13 CrossRef.
- R. R. Navarro, Y. Otsuka, K. Matsuo, K. Sasaki, K. Sasaki, T. Hori, H. Habe, M. Nakamura, Y. Nakashimada, K. Kimbara and J. Kato, Combined simultaneous enzymatic saccharification and comminution (SESC) and anaerobic digestion for sustainable biomethane generation from wood lignocellulose and the biochemical characterization of residual sludge solid, Bioresour. Technol., 2020, 300, 122622 CrossRef CAS PubMed.
- P. Salehian, K. Karimi, H. Zilouei and A. Jeihanipour, Improvement of biogas production from pine wood by alkali pretreatment, Fuel, 2013, 106, 484–489 CrossRef CAS.
- A. Vlyssides, S. Mai and E. M. Barampouti, Energy Generation Potential in Greece From Agricultural Residues and Livestock Manure by Anaerobic Digestion Technology, Waste Biomass Valorization, 2015, 6, 747–757 CrossRef.
- A. O. Avcioǧlu and U. Türker, Status and potential of biogas energy from animal wastes in Turkey, Renew. Sust. Energ. Rev., 2012, 16, 1557–1561 CrossRef.
- M. Caliskan and N. F. Tumen Ozdil, Potential of Biogas and Electricity Production from Animal Waste in Turkey, Bioenergy Res., 2021, 14, 860–869 CrossRef.
- S. M. Safieddin Ardebili, Green electricity generation potential from biogas produced by anaerobic digestion of farm animal waste and agriculture residues in Iran, Renew. Energy, 2020, 154, 29–37 CrossRef CAS.
- P. Abdeshahian, J. S. Lim, W. S. Ho, H. Hashim and C. T. Lee, Potential of biogas production from farm animal waste in Malaysia, Renew. Sust. Energ. Rev., 2016, 60, 714–723 CrossRef CAS.
- M. Scheftelowitz and D. Thrän, Unlocking the energy potential of manure-an assessment of the biogas production potential at the farm level in Germany, Agriculture, 2016, 6(2), 20 CrossRef.
- M. Khalil, M. A. Berawi, R. Heryanto and A. Rizalie, Waste to energy technology: The potential of sustainable biogas production from animal waste in Indonesia, Renew. Sust. Energ. Rev., 2019, 105, 323–331 CrossRef.
- J. L. Ramos-Suárez, A. Ritter, J. Mata González and A. Camacho Pérez, Biogas from animal manure: A sustainable energy opportunity in the Canary Islands, Renew. Sust. Energ. Rev., 2019, 104, 137–150 CrossRef.
- S. O. Jekayinfa, J. I. Orisaleye and R. Pecenka, An assessment of potential resources for biomass energy in Nigeria, Resources, 2020, 9, 1–41 CrossRef.
- A. C. L. de Oliveira, R. S. Milagres, W. de A. Orlando Junior and N. dos S. Renato, Evaluation of Brazilian potential for generating electricity through animal manure and sewage, Biomass Bioenergy, 2020, 139, 105654 CrossRef.
- M. U. Khan, M. Ahmad, M. Sultan, I. Sohoo, P. C. Ghimire, A. Zahid, A. Sarwar, M. Farooq, U. Sajjad, P. Abdeshahian and M. Yousaf, Biogas Production Potential from Livestock Manure in Pakistan, Sustainability, 2021, 13, 748–754 CrossRef.
-
Tamil Nadu Agricultural University, Expert system for sheep and goat, https://www.agritech.tnau.ac.in/expert_system/sheepgoat/breeds.html#:%7E:text=Ramsweight80-110kg,performingwellundermostconditions Search PubMed.
-
R. M. Acharya, Sheep and goat breeds of India, FAO, Rome, Italy, 1982 Search PubMed.
-
Central Sheep and Wool Research Institute, Sheep database management system, https://cswri.res.in/breed_profiles.asp Search PubMed.
-
FAO, Domestic Animal Diversity Information System (DAD-IS), https://www.fao.org/dad-is/browse-by-country-and-species/en/ Search PubMed.
-
Government of India, Basic Animal Husbandry Statistics – 2019, New Delhi, India, 2019 Search PubMed.
- M. Arshad, I. Bano, N. Khan, M. I. Shahzad, M. Younus, M. Abbas and M. Iqbal, Electricity generation from biogas of poultry waste: An assessment of potential and feasibility in Pakistan, Renew. Sust. Energ. Rev., 2018, 81, 1241–1246 CrossRef.
- S. Saini, P. Rao and Y. Patil, City Based Analysis of MSW to Energy Generation in India, Calculation of State-Wise Potential and Tariff Comparison with EU, Procedia Soc. Behav. Sci., 2012, 37, 407–416 CrossRef.
-
CPCB, Waste Generation and Composition, 2005 Search PubMed.
-
CPCB, Solid Waste Management Rules 2018–19Annual Report, Delhi, India, 2019 Search PubMed.
- M. S. Rao, S. P. Singh, A. K. Singh and M. S. Sodha, Bioenergy conversion studies of the organic fraction of MSW: Assessment of ultimate bioenergy production potential of municipal garbage, Appl. Energy, 2000, 66, 75–87 CrossRef CAS.
- Å. Davidsson, C. Gruvberger, T. H. Christensen, T. L. Hansen and J. la C. Jansen, Methane yield in source-sorted organic fraction of municipal solid waste, Waste Manage., 2007, 27, 406–414 CrossRef PubMed.
- V. Dhanalakshmi Sridevi, T. Rema and S. v. Srinivasan, Studies on biogas production from vegetable market wastes in a two-phase anaerobic reactor, Clean Technol. Environ. Policy, 2015, 17, 1689–1697 CrossRef CAS.
- B. K. Majhi and T. Jash, Two-phase anaerobic digestion of vegetable market waste fraction of municipal solid waste and development of improved technology for phase separation in two-phase reactor, Waste Manage., 2016, 58, 152–159 CrossRef CAS PubMed.
- V. K. Tyagi, A. Kapoor, P. Arora, J. R. Banu, S. Das, S. Pipesh and A. A. Kazmi, Mechanical-biological treatment of municipal solid waste: Case study of 100 TPD Goa plant, India, J. Environ. Manage., 2021, 292, 112741 CrossRef CAS PubMed.
- V. Talyan, R. P. Dahiya and T. R. Sreekrishnan, State of municipal solid waste management in Delhi, the capital of India, Waste Manage., 2008, 28, 1276–1287 CrossRef CAS PubMed.
- S. Chattopadhyay, A. Dutta and S. Ray, Municipal solid waste management in Kolkata, India – A review, Waste Manage., 2009, 29, 1449–1458 CrossRef CAS PubMed.
- S. S. Baral, D. Dionisi, D. Maarisetty, A. Gandhi, A. Kothari, G. Gupta and P. Jain, Biofuel production potential from wastewater in India by integrating anaerobic membrane reactor with algal photobioreactor, Biomass Bioenergy, 2020, 133, 105445 CrossRef CAS.
-
S. Khan and J. Ali, in Bioassays: Advanced Methods and Applications, ed. D.-P. Häder and G. S. Erzinger, Elsevier Inc., 2018, pp. 21–39 Search PubMed.
- B. Lew, S. Tarre, M. Beliavski, C. Dosoretz and M. Green, Anaerobic membrane bioreactor (AnMBR) for domestic wastewater treatment, Desalination, 2009, 243, 251–257 CrossRef CAS.
- D. Martinez-Sosa, B. Helmreich, T. Netter, S. Paris, F. Bischof and H. Horn, Anaerobic submerged membrane bioreactor (AnSMBR) for municipal wastewater treatment under mesophilic and psychrophilic temperature conditions, Bioresour. Technol., 2011, 102, 10377–10385 CrossRef CAS PubMed.
- E. Meabe, S. Déléris, S. Soroa and L. Sancho, Performance of anaerobic membrane bioreactor for sewage sludge
treatment: Mesophilic and thermophilic processes, J. Memb. Sci., 2013, 446, 26–33 CrossRef CAS.
- P. J. van Zyl, M. C. Wentzel, G. A. Ekama and K. J. Riedel, Design and start-up of a high rate anaerobic membrane bioreactor for the treatment of a low pH, high strength, dissolved organic waste water, Water Sci. Technol., 2008, 57, 291–295 CrossRef CAS PubMed.
- J. B. Giménez, A. Robles, L. Carretero, F. Durán, M. v. Ruano, M. N. Gatti, J. Ribes, J. Ferrer and A. Seco, Experimental study of the anaerobic urban wastewater treatment in a submerged hollow-fibre membrane bioreactor at pilot scale, Bioresour. Technol., 2011, 102, 8799–8806 CrossRef PubMed.
- S. Sarkar, P. B. Ghosh, K. Mukherjee, A. K. Sil and T. Saha, Sewage treatment in a single pond system at East Kolkata Wetland, India, Water Sci. Technol., 2009, 60, 2309–2317 CrossRef CAS PubMed.
- P. Jamwal, A. K. Mittal and J. M. Mouchel, Efficiency evaluation of sewage treatment plants with different technologies in Delhi (India), Environ. Monit. Assess., 2009, 153, 293–305 CrossRef CAS PubMed.
- P. Jamwal, T. M. Zuhail, P. R. Urs, V. Srinivasan and S. Lele, Contribution of sewage treatment to pollution abatement of urban streams, Curr. Sci., 2015, 108, 677–685 CAS.
- B. Kulkarni, R. v. Wanjule and H. H. Shinde, Study on Sewage Quality from Sewage Treatment Plant at Vashi, Navi Mumbai, Mater. Today Proc., 2018, 5, 1859–1863 CrossRef CAS.
- V. Kumar and A. K. Chopra, Monitoring of physico-chemical and microbiological characteristics of Municipal Wastewater at treatment plant, Haridwar city (Uttarakhand) India, J. Environ. Sci. Technol., 2012, 5, 109–118 CrossRef CAS.
- M. Pradesh, N. Shrivastava, D. D. Mishra, P. K. Mishra and A. Bajpai, Water Quality Deterioration of Machna River due to Sewage, J. Environ. Earth Sci., 2013, 3, 1–6 Search PubMed.
- K. R. Venkatesh, M. Rajendran and A. Murugappan, A correlation study on physico-chemical characteristics of domestic sewage, Nat. Environ. Pollut. Technol., 2009, 8, 141–145 CAS.
-
Government of India, National status of waste water generation & treatment, https://www.sulabhenvis.nic.in/Database/STST_wastewater_2090.aspx Search PubMed.
-
C. Sawatdeenarunat, C. Wangnai, W. Songkasiri, P. Panichnumsin, K. Saritpongteeraka, P. Boonsawang, S. K. Khanal and S. Chaiprapat, in Biomass, Biofuels, Biochemicals, Biofuels: Alternative Feedstocks and Conversion Processes for the Production of Liquid and Gaseous Biofuels, Elsevier Inc., 2nd edn, 2019, pp. 779–816 Search PubMed.
-
A. Dey and R. C. Thomson, Biomethane generation potential of wastes and wastewaters from the sericulture, fisheries, and industrial sectors: a case study for India (unpublished), in review.
- A. Garg, S. Vishwanathan and V. Avashia, Life cycle greenhouse gas emission assessment of major petroleum oil products for transport and household sectors in India, Energy Policy, 2013, 58, 38–48 CrossRef CAS.
- O. Hijazi, S. Munro, B. Zerhusen and M. Effenberger, Review of life cycle assessment for biogas production in Europe, Renew. Sust. Energ. Rev., 2016, 54, 1291–1300 CrossRef CAS.
-
N. Labutong, J. Mosley, R. Smith and J. Willard, Life-Cycle Modeling and Environmental Impact Assessment of Commercial Scale, A project submitted in partial fulfillment of the requirements for the degree of Master of Science Natural Resources and Environment, University of Michigan, 2012, https://css.umich.edu/publications/research-publications/life-cycle-modeling-and-environmental-impact-assessment Search PubMed.
- A. D. Singh, A. Upadhyay, S. Shrivastava and V. Vivekanand, Life-cycle assessment of sewage sludge-based large-scale biogas plant, Bioresour. Technol., 2020, 309, 123373 CrossRef CAS PubMed.
- H. Hahn, K. Hartmann, L. Bühle and M. Wachendorf, Comparative life cycle assessment of biogas plant configurations for a demand oriented biogas supply for flexible power generation, Bioresour. Technol., 2015, 179, 348–358 CrossRef CAS PubMed.
- P. Singh, H. Gundimeda and M. Stucki, Environmental footprint of cooking fuels: A life cycle assessment of ten fuel sources used in Indian households, Int. J. Life Cycle Assess., 2014, 19, 1036–1048 CrossRef CAS.
- T. Kvist and N. Aryal, Methane loss from commercially operating biogas upgrading plants, Waste Manage., 2019, 87, 295–300 CrossRef CAS PubMed.
- J. Ciuła, V. Kozik, A. Generowicz, K. Gaska, A. Bak, M. Paździor and K. Barbusiński, Emission and Neutralization of Methane from a Municipal Landfill-Parametric Analysis, Energies, 2020, 13, 6254 CrossRef.
-
B. Das and T. Hazra, in Spatial Modeling and Assessment of Environmental Contaminants: Risk Assessment and Remediation, ed. P. K. Shit, P. P. Adhikary and D. Sengupta, Springer Nature Switzerland, Cham, Switzerland, 1st edn, 2021, pp. 55–68 Search PubMed.
- F. A. Abdulla and Z. D. Al-Ghazzawi, Methane emissions from domestic waste management facilities in Jordan—applicability of ipcc methodology, J. Air Waste Manage. Assoc., 2000, 50, 234–239 CrossRef CAS PubMed.
- K. A. Lyng and A. Brekke, Environmental life cycle assessment of biogas as a fuel for transport compared with alternative fuels, Energies, 2019, 12, 1–12 CrossRef.
-
P. Forster, T. Storelvmo, K. Armour, J.-L. W. Collins, D. F. Dufresne, D. J. Lunt, T. Mauritsen, M. D. Palmer, M. Watanabe, A. M. Wild and H. Zhang, in Climate Change 2021: The Physical Science Basis. Contribution of Working Group I to the Sixth Assessment Report of the Intergovernmental Panel on Climate Change, ed. Masson-Delmotte P. Z. V., A. Pirani, S. L. Connors, C. Péan, S. Berger, N. Caud, Y. Chen, L. Goldfarb, M. I. Gomis, M. Huang, K. Leitzell, E. Lonnoy, J. B. R. Matthews, T. K. Maycock, T. Waterfield, O. Yelekçi, R. Yu and B. Zhou, Cambridge University Press, 2021, vol. 17, pp. 691–698 Search PubMed.
- J. D. Wojcik and J. Wang, Feasibility study of Combined Cycle Gas Turbine (CCGT) power plant integration with Adiabatic Compressed Air Energy Storage (ACAES), Appl. Energy, 2018, 221, 477–489 CrossRef.
- J. Kotowicz and M. Brzęczek, Analysis of increasing efficiency of modern combined cycle power plant: A case study, Energy, 2018, 153, 90–99 CrossRef.
- J. Kotowicz, M. Brzęczek and M. Job, The thermodynamic and economic characteristics of the modern combined cycle power plant with gas turbine steam cooling, Energy, 2018, 164, 359–376 CrossRef.
- J. Kotowicz, M. Job and M. Brzeczek, The characteristics of ultramodern combined cycle power plants, Energy, 2015, 92, 197–211 CrossRef.
-
IEA, Average CO2 emissions intensity of hourly electricity supply in India, 2018 and 2040 by scenario and average electricity demand in 2018, https://www.iea.org/data-and-statistics/charts/average-co2-emissions-intensity-of-hourly-electricity-supply-in-india-2018-and-2040-by-scenario-and-average-electricity-demand-in-2018 Search PubMed.
-
Government of India, Indian Petroleum and Natural Gas Statistics 2019–20, New Delhi, India, 2020 Search PubMed.
- N. D. Rao, J. Min and A. Mastrucci, Energy requirements for decent living in India, Brazil and South Africa, Nat. Energy, 2019, 4, 1025–1032 CrossRef.
- K. K. Agrawal, S. Jain, A. K. Jain and S. Dahiya, A life cycle environmental impact assessment of natural gas combined cycle thermal power plant in Andhra Pradesh, India, Environ. Dev., 2014, 11, 162–174 CrossRef.
- C. Veluchamy, V. W. Raju and A. S. Kalamdhad, Electrohydrolysis pretreatment for enhanced methane production from lignocellulose waste pulp and paper mill sludge and its kinetics, Bioresour. Technol., 2018, 252, 52–58 CrossRef CAS PubMed.
- W. C. Nadaleti and G. Przybyla, Emissions and performance of a spark-ignition gas engine generator operating with hydrogen-rich syngas, methane and biogas blends for application in southern Brazilian rice industries, Energy, 2018, 154, 38–51 CrossRef CAS.
- G. Tang, P. Jin, Y. Bao, W. S. Chai and L. Zhou, Experimental investigation of premixed combustion limits of hydrogen and methane additives in ammonia, Int. J. Hydrog. Energy, 2021, 46, 20765–20776 CrossRef CAS.
-
The Engineering Toolbox, Fuels – Higher and Lower Calorific Values, https://www.engineeringtoolbox.com/fuels-higher-calorific-values-d_169.html, (accessed 15 September 2022) Search PubMed.
- K. Lejda, A. Jaworski, M. Maksymilian, K. Balawender, A. Ustrzycki and D. Savostin-Kosiak, Assessment of Petrol and Natural Gas Vehicle Carbon Oxides Emissions in the Laboratory and On-Road Tests, Energies, 2021, 14(6), 1631 CrossRef CAS.
- Z. Liu and I. A. Karimi, New operating strategy for a combined cycle gas turbine power plant, Energy Convers. Manag., 2018, 171, 1675–1684 CrossRef.
- T. k. Ibrahim, M. Kamil, O. I. Awad, M. M. Rahman, G. Najafi, F. Basrawi, A. N. Abd Alla and R. Mamat, The optimum performance of the combined cycle power plant: A comprehensive review, Renew. Sust. Energ. Rev., 2017, 79, 459–474 CrossRef.
- J. Vancoillie, J. Demuynck, L. Sileghem, M. van de Ginste and S. Verhelst, Comparison of the renewable transportation fuels, hydrogen and methanol formed from hydrogen, with gasoline – Engine efficiency study, Int. J. Hydrog. Energy, 2012, 37, 9914–9924 CrossRef CAS.
- B. S. Verhelst, T. Wallner, H. Eichlseder and K. Naganuma, Electricity Powering Combustion: Hydrogen Engines, Proc. IEEE, 2011, 100(2), 427–439 Search PubMed.
- A. Yousefi, H. Guo, M. Birouk and B. Liko, On greenhouse gas emissions and thermal efficiency of natural gas/diesel dual-fuel engine at low load conditions: Coupled effect of injector rail pressure and split injection, Appl. Energy, 2019, 242, 216–231 CrossRef CAS.
- B. N. Agrawal, S. Sinha, A. v. Kuzmin and V. A. Pinchuk, Effect of vegetable oil share on combustion characteristics and thermal efficiency of diesel engine fueled with different blends, Therm. Sci. Eng. Prog., 2019, 14, 100404 CrossRef.
- C. Park, C. Kim, S. Lee, S. Lee and J. Lee, Comparative evaluation of performance and emissions of CNG engine for heavy-duty vehicles fueled with various caloric natural gases, Energy, 2019, 174, 1–9 CrossRef CAS.
- Q. Zhang, Z. Li, Z. Wei, M. Li and X. Zheng, Experiment investigation on the emission characteristics of a stoichiometric natural gas engine operating with different reference fuels, Fuel, 2020, 269, 117449 CrossRef CAS.
- D. Splitter, V. Boronat, F. D. F. Chuahy and J. Storey, Performance of direct injected propane and gasoline in a high stroke-to-bore ratio SI engine: Pathways to diesel efficiency parity with ultra low soot, Int. J. Engine Res., 2021, 22, 3475–3488 CrossRef CAS.
- K. Ravindra, T. Singh and S. Mor, Emissions of air pollutants from primary crop residue burning in India and their mitigation strategies for cleaner emissions, J J. Clean Prod., 2019, 208, 261–273 CrossRef CAS.
- N. Santiago-De La Rosa, G. González-Cardoso, J. de J. Figueroa-Lara, M. Gutiérrez-Arzaluz, C. Octaviano-Villasana, I. F. Ramírez-Hernández and V. Mugica-Álvarez, Emission factors of atmospheric and climatic pollutants from crop residues burning, J. Air Waste Manage. Assoc., 2018, 68, 849–865 CrossRef CAS PubMed.
- T. Kanabkaew and N. T. K. Oanh, Development of Spatial and Temporal Emission Inventory for Crop Residue Field Burning, Environ. Model. Assess., 2011, 16, 453–464 CrossRef.
- H. Zhang, J. Hu, Y. Qi, C. Li, J. Chen, X. Wang, J. He, S. Wang, J. Hao, L. Zhang, L. Zhang, Y. Zhang, R. Li, S. Wang and F. Chai, Emission characterization, environmental impact, and control measure of PM2.5 emitted from agricultural crop residue burning in China, J. Clean Prod., 2017, 149, 629–635 CrossRef CAS.
- G. CAO, X. ZHANG, S. GONG and F. ZHENG, Investigation on emission factors of particulate matter and gaseous pollutants from crop residue burning, J. Environ. Sci., 2008, 20, 50–55 CrossRef CAS PubMed.
- R. Gao, W. Jiang, W. Gao and S. Sun, Emission inventory of crop residue open burning and its high-resolution spatial distribution in 2014 for Shandong province, China, Atmos. Pollut. Res., 2017, 8, 545–554 CrossRef.
- B. Das, P. v. Bhave, S. P. Puppala, K. Shakya, B. Maharjan and R. M. Byanju, A model-ready emission inventory for crop residue open burning in the context of Nepal, Environ. Pollut., 2020, 266, 115069 CrossRef CAS PubMed.
- X. Zhang, Y. Lu, Q. Wang and X. Qian, A high-resolution inventory of air pollutant emissions from crop residue burning in China, Atmos. Environ., 2019, 213, 207–214 CrossRef CAS.
- V. Ramanathan and G. Carmichael, Global and regional climate changes due to black carbon, Nat. Geosci., 2008, 1, 221–227 CrossRef CAS.
- T. C. Bond and K. Sun, Can reducing black carbon emissions counteract global warming?, Environ. Sci. Technol., 2005, 39, 5921–5926 CrossRef CAS PubMed.
- T. C. Bond, C. Zarzycki, M. G. Flanner and D. M. Koch, Quantifying immediate radiative forcing by black carbon and organic matter with the Specific Forcing Pulse, Atmos. Chem. Phys., 2011, 11, 1505–1525 CrossRef CAS.
- C. K. Singh, A. Kumar and S. S. Roy, Quantitative analysis of the methane gas emissions from municipal solid waste in India, Sci. Rep., 2018, 8, 1–8 CAS.
- P. Ghosh, G. Shah, R. Chandra, S. Sahota, H. Kumar, V. K. Vijay and I. S. Thakur, Assessment of methane emissions and energy recovery potential from the municipal solid waste landfills of Delhi, India, Bioresour. Technol., 2019, 272, 611–615 CrossRef CAS PubMed.
-
Government of India, Energy Statistics India – 2022, 2022 Search PubMed.
- S. Ghosh, S. Dey, D. Ganguly, S. Baidya Roy and K. Bali, Cleaner air would enhance India's annual solar energy production by 6–28 TWh, Environ. Res. Lett., 2022, 17, 054007 CrossRef.
- N. Duan, B. Khoshnevisan, C. Lin, Z. Liu and H. Liu, Life cycle assessment of anaerobic digestion of pig manure coupled with different digestate treatment technologies, Environ. Int., 2020, 137, 105522 CrossRef CAS PubMed.
-
S. Z. Ahammad and T. R. Sreekrishnan, in Green Fuels Technology: Biofuels, ed. C. R. Soccol, S. Kaur Brar, C. Faulds and L. Pereira, Springer International Publishing, 2016, pp. 438–450 Search PubMed.
- P. Satpathy and C. Pradhan, Biogas as an alternative to stubble burning in India, Biomass Convers Biorefin, 2020 DOI:10.1007/s13399-020-01131-z.
- A. Muralidharan, Feasibility, health and economic impact of generating biogas from human excreta for the state of Tamil Nadu, India, Renew. Sust. Energ. Rev., 2017, 69, 59–64 CrossRef.
- G. Surie, Achieving sustainability: Insights from biogas ecosystems in India, Agriculture, 2017, 7(5), 15 CrossRef.
- J. C. Motte, E. Trably, R. Escudié, J. Hamelin, J. P. Steyer, N. Bernet, J. P. Delgenes and C. Dumas, Total solids content: A key parameter of metabolic pathways in dry anaerobic digestion, Biotechnol. Biofuels, 2013, 6, 1–9 CrossRef PubMed.
- D. Brown, J. Shi and Y. Li, Comparison of solid-state to liquid anaerobic digestion of lignocellulosic feedstocks for biogas production, Bioresour. Technol., 2012, 124, 379–386 CrossRef CAS PubMed.
- G. chao Sun, Y. Z. Wu, S. jin Sha and K. xin Liu, Dry digestion of crop wastes: Studies on dry anaerobic digestion with agricultural wastes, Biological Wastes, 1987, 20, 291–302 CrossRef.
-
A. Ohji and M. Haraguchi, in Advances in Steam Turbines for Modern Power Plants, Woodhead Publishing, Elsevier, 2017, pp. 11–40 Search PubMed.
- D. Elango, M. Pulikesi, P. Baskaralingam, V. Ramamurthi and S. Sivanesan, Production of biogas from municipal solid waste with domestic sewage, J. Hazard Mater., 2007, 141, 301–304 CrossRef CAS PubMed.
- S. O-Thong, K. Boe and I. Angelidaki, Thermophilic anaerobic co-digestion of oil palm empty fruit bunches with palm oil mill effluent for efficient biogas production, Appl. Energy, 2012, 93, 648–654 CrossRef CAS.
- J. Charles Rajesh Kumar and M. A. Majid, Renewable energy for sustainable development in India: Current status, future prospects, challenges, employment, and investment opportunities, Energy Sustain. Soc., 2020, 10, 1–36 Search PubMed.
- M. R. Nouni, P. Jha, R. Sarkhel, C. Banerjee, A. K. Tripathi and J. Manna, Alternative fuels for decarbonisation of road transport sector in India: Options, present status, opportunities, and challenges, Fuel, 2021, 305, 121583 CrossRef CAS.
- D. Singh, S. Pachauri and H. Zerriffi, Environmental payoffs of LPG cooking in India, Environ. Res. Lett., 2017, 12(11), 115003 CrossRef.
- C. F. Gould and J. Urpelainen, LPG as a clean cooking fuel: Adoption, use, and impact in rural India, Energy Policy, 2018, 122, 395–408 CrossRef PubMed.
- R. Vidhi and P. Shrivastava, A review of electric vehicle lifecycle emissions and policy recommendations to increase EV penetration in India, Energies, 2018, 11, 1–15 Search PubMed.
-
S. Agrawal, S. Mani, K. Ganesan and A. Jain, Are Indian Homes Ready for Electric Cooking?, Policy Brief, Council on Energy, Environment, and Water, 2020, https://www.ceew.in/publications/are-indian-homes-ready-for-electric-cooking-transition#:~:text=Key%20Findings,users%2C%20followed%20by%20microwave%20ovens Search PubMed.
- S. Mani, A. Jain, S. Tripathi and C. F. Gould, The drivers of sustained use of liquified petroleum gas in India, Nat. Energy, 2020, 5, 450–457 CrossRef PubMed.
- N. Chindarkar, A. Jain and S. Mani, Examining the willingness-to-pay for exclusive use of LPG for cooking among rural households in India, Energy Policy, 2021, 150, 112107 CrossRef.
-
UNDP, Sustainable Development Goals, https://www.undp.org/content/undp/en/home/sustainable-development-goals.html Search PubMed.
- S. Kumar, K. Paritosh, N. Pareek, A. Chawade and V. Vivekanand, De-construction of major Indian cereal crop residues through chemical pretreatment for improved biogas production: An overview, Renew. Sust. Energ. Rev., 2018, 90, 160–170 CrossRef CAS.
- L. M. Contreras, H. Schelle, C. R. Sebrango and I. Pereda, Methane potential and biodegradability of rice straw, rice husk and rice residues from the drying process, Water Sci. Technol., 2012, 65, 1142–1149 CrossRef CAS PubMed.
- M. Hiloidhari and D. C. Baruah, Rice straw residue biomass potential for decentralized electricity generation: A GIS based study in Lakhimpur district of Assam, India, Energy Sustain. Dev., 2011, 15, 214–222 CrossRef.
- Y. Gu, Y. Zhang and X. Zhou, Effect of Ca(OH)2 pretreatment on extruded rice straw anaerobic digestion, Bioresour. Technol., 2015, 196, 116–122 CrossRef CAS PubMed.
- S. K. Sharma, I. M. Mishra, M. P. Sharma and J. S. Saini, Effect of particle size on biogas generation from biomass residues, Biomass, 1988, 17, 251–263 CrossRef CAS.
- B. Jha, A. Isha, A. Trivedi, R. Chandra and P. M. V. Subbarao, Anaerobic co-digestion of rice straw and de-oiled rice bran for biomethane production, Energy Reports, 2021, 7, 704–710 CrossRef.
- M. Elsayed, A. E. F. Abomohra, P. Ai, D. Wang, H. M. El-Mashad and Y. Zhang, Biorefining of rice straw by sequential fermentation and anaerobic digestion for bioethanol and/or biomethane production: Comparison of structural properties and energy output, Bioresour. Technol., 2018, 268, 183–189 CrossRef CAS PubMed.
- A. M. Mustafa, T. G. Poulsen, Y. Xia and K. Sheng, Combinations of fungal and milling pretreatments for enhancing rice straw biogas production during solid-state anaerobic digestion, Bioresour. Technol., 2017, 224, 174–182 CrossRef CAS PubMed.
- R. Chandra, H. Takeuchi and T. Hasegawa, Hydrothermal pretreatment of rice straw biomass: A potential and promising method for enhanced methane production, Appl. Energy, 2012, 94, 129–140 CrossRef CAS.
- M. Dehghani, K. Karimi and M. Sadeghi, Pretreatment of rice straw for the improvement of biogas production, Energy Fuels, 2015, 29, 3770–3775 CrossRef CAS.
- M. S. Kalra and J. S. Panwar, Anaerobic digestion of rice crop residues, Agricultural Wastes, 1986, 17, 263–269 CrossRef CAS.
- N. H. Garcia, A. Mattioli, A. Gil, N. Frison, F. Battista and D. Bolzonella, Evaluation of the methane potential of different agricultural and food processing substrates for improved biogas production in rural areas, Renew. Sust. Energ. Rev., 2019, 112, 1–10 CrossRef CAS.
- M. Pohl, J. Mumme, K. Heeg and E. Nettmann, Bioresource Technology Thermo- and mesophilic anaerobic digestion of wheat straw by the upflow anaerobic solid-state (UASS) process, Bioresour. Technol., 2012, 124, 321–327 CrossRef CAS PubMed.
- J. Singh, B. S. Panesar and S. K. Sharma, Energy potential through agricultural biomass using geographical information system-A case study of Punjab, Biomass Bioenergy, 2008, 32, 301–307 Search PubMed.
- Z. Yong, Y. Dong, X. Zhang and T. Tan, Anaerobic co-digestion of food waste and straw for biogas production, Renew. Energy, 2015, 78, 527–530 CrossRef CAS.
- L. C. Ferreira, P. J. Nilsen, F. Fdz-Polanco and S. I. Pérez-Elvira, Biomethane potential of wheat straw: Influence of particle size, water impregnation and thermal hydrolysis, Chem. Eng. J, 2014, 242, 254–259 CrossRef CAS.
- G. Mancini, S. Papirio, P. N. L. Lens and G. Esposito, Increased biogas production from wheat straw by chemical pretreatments, Renew. Energy, 2018, 119, 608–614 CrossRef CAS.
- R. Chandra, H. Takeuchi, T. Hasegawa and R. Kumar, Improving biodegradability and biogas production of wheat straw substrates using sodium hydroxide and hydrothermal pretreatments, Energy, 2012, 43, 273–282 CrossRef CAS.
- A. A. Rajput, Zeshan and C. Visvanathan, Effect of thermal pretreatment on chemical composition, physical structure and biogas production kinetics of wheat straw, J. Environ. Manage., 2018, 221, 45–52 CrossRef CAS PubMed.
- M. Elsayed, Y. Andres, W. Blel, A. Gad and A. Ahmed, Effect of VS organic loads and buckwheat husk on methane production by anaerobic co-digestion of primary sludge and wheat straw, Energy Convers. Manag., 2016, 117, 538–547 CrossRef CAS.
- T. Liu, X. Zhou, Z. Li, X. Wang and J. Sun, Effects of liquid digestate pretreatment on biogas production for anaerobic digestion of wheat straw, Bioresour. Technol., 2019, 280, 345–351 CrossRef CAS PubMed.
- V. Dandikas, H. Heuwinkel, F. Lichti, J. E. Drewes and K. Koch, Correlation between biogas yield and chemical composition of energy crops, Bioresour. Technol., 2014, 174, 316–320 CrossRef CAS PubMed.
- Y. Li, Y. Li, D. Zhang, G. Li, J. Lu and S. Li, Solid state anaerobic co-digestion of tomato residues with dairy manure and corn stover for biogas production, Bioresour. Technol., 2016, 217, 50–55 CrossRef CAS PubMed.
- D. Cardoen, P. Joshi, L. Diels, P. M. Sarma and D. Pant, Agriculture biomass in India: Part 1. Estimation and characterization, Resour. Conserv. Recycl., 2015, 102, 39–48 CrossRef.
- T. Raj, M. Kapoor, R. Gaur, J. Christopher, B. Lamba, D. K. Tuli and R. Kumar, Physical and chemical characterization of various indian agriculture residues for biofuels production, Energy Fuels, 2015, 29, 3111–3118 CrossRef CAS.
- J. Shen, S. Zhu, X. Liu, H. Zhang and J. Tan, The prediction of elemental composition of biomass based on proximate analysis, Energy Convers. Manag., 2010, 51, 983–987 CrossRef CAS.
- V. N. Gunaseelan, Biochemical methane potential of fruits and vegetable solid waste feedstocks, Biomass Bioenergy, 2004, 26, 389–399 CrossRef CAS.
- P. Li, W. Li, M. Sun, X. Xu, B. Zhang and Y. Sun, Evaluation of biochemical methane potential and kinetics on the anaerobic digestion of vegetable crop residues, Energies, 2019, 12(1), 26 CrossRef CAS.
- K. Hamraoui, A. Gil, H. El Bari, J. A. Siles, A. F. Chica and M. A. Martín, Evaluation of hydrothermal pretreatment for biological treatment of lignocellulosic feedstock (pepper plant and eggplant), Waste Manage., 2020, 102, 76–84 CrossRef CAS PubMed.
-
T. Ronzon, S. Piotrowski and M. Carus, DataM – Biomass estimates (v3): a new database to quantify biomass availability in the European Union, 2015 Search PubMed.
- J. Akinbomi, T. Brandberg, S. A. Sanni and M. J. Taherzadeh, Development and Dissemination Strategies for Accelerating Biogas Production in Nigeria, Bioresources, 2014, 9, 5707–5737 Search PubMed.
- H. Mu, C. Zhao, Y. Zhao, Y. Li, D. Hua, X. Zhang and H. Xu, Enhanced methane production by semi-continuous mesophilic co-digestion of potato waste and cabbage waste: Performance and microbial characteristics analysis, Bioresour. Technol., 2017, 236, 68–76 CrossRef CAS PubMed.
- H. Escalante, L. Castro, P. Gauthier-Maradei and R. Rodríguez De La Vega, Spatial decision support system to evaluate crop residue energy potential by anaerobic digestion, Bioresour. Technol., 2016, 219, 80–90 CrossRef CAS PubMed.
- J. Gao, A. Zhang, S. K. Lam, X. Zhang, A. M. Thomson, E. Lin, K. Jiang, L. E. Clarke, J. A. Edmonds, P. G. Kyle, S. Yu, Y. Zhou and S. Zhou, An integrated assessment of the potential of agricultural and forestry residues for energy production in China, GCB Bioenergy, 2016, 8, 880–893 CrossRef.
- E. Zhang, J. Li, K. Zhang, F. Wang, H. Yang, S. Zhi and G. Liu, Anaerobic digestion performance of sweet potato vine and animal manure under wet, semi-dry, and dry conditions, AMB Express, 2018, 8, 45 CrossRef PubMed.
- S. O. Jekayinfa and V. Scholz, Laboratory scale preparation of biogas from cassava tubers, cassava peels, and palm kernel oil residues, Energy Sources, Part A, 2013, 35, 2022–2032 CrossRef CAS.
- J. Lansche, S. Awiszus, S. Latif and J. Müller, Potential of biogas production from processing residues to reduce environmental impacts from cassava starch and crisp production-a case study from Malaysia, Appl. Sci., 2020, 10(8), 2975 CrossRef CAS.
- S. N. Ugwu and C. C. Enweremadu, Effects of pre-treatments and co-digestion on biogas production from Okra waste, J. Renew. Sustain. Energy, 2019, 11(1), 013101 CrossRef.
- M. Oleszek, J. Tys, D. Wiącek, A. Król and J. Kuna, The Possibility of Meeting Greenhouse Energy and CO2 Demands Through Utilisation of Cucumber and Tomato Residues, Bioenergy Res., 2016, 9, 624–632 CrossRef CAS.
- I. Beniche, H. El Bari, J. A. Siles, A. F. Chica and M. Á. Martín, Methane production by anaerobic co-digestion of mixed agricultural waste: cabbage and cauliflower, Environ. Technol., 2020, 1–9 Search PubMed.
- H. Yan, C. Zhao, J. Zhang, R. Zhang, C. Xue, G. Liu and C. Chen, Study on biomethane production and biodegradability of different leafy vegetables in anaerobic digestion, AMB Express, 2017, 7, 1–9 CrossRef PubMed.
- T. Zhang, Y. Yang and D. Xie, Insights into the production potential and trends of China's rural biogas, Int. J. Energy Res., 2015, 39, 1068–1082 CrossRef.
- M. Kim, S. Kim and S. Kim, Effect of proximate composition ratios for biogas production, J. Biosyst Eng., 2017, 42, 155–162 Search PubMed.
- K. M. Akkoli, P. B. Gangavati, M. R. Ingalagi and R. K. Chitgopkar, Assessment and characterization of agricultural residues, Mater. Today. Proc., 2018, 5, 17548–17552 CrossRef CAS.
- A. B. Guerrero, P. L. Aguado, J. Sánchez and M. D. Curt, GIS-Based Assessment of Banana Residual Biomass Potential for Ethanol Production and Power Generation: A Case Study, Waste Biomass Valorization, 2016, 7, 405–415 CrossRef CAS.
- C. Li, G. Liu, I. A. Nges, L. Deng, M. Nistor and J. Liu, Fresh banana pseudo-stems as a tropical lignocellulosic feedstock for methane production, Energy Sustain. Soc., 2016, 6, 27 CrossRef.
- L. Zhou, F. Guo, S. Pan, B. Lu, L. Du and Y. Wei, Synergistic digestion of banana pseudo-stems with chicken manure to improve methane production: Semi-continuous manipulation and microbial community analysis, Bioresour. Technol., 2021, 328, 124851 CrossRef CAS PubMed.
- P. v. Gokul, P. Singh, V. P. Singh and A. N. Sawarkar, Thermal behavior and kinetics of pyrolysis of areca nut husk, Energy Sources, Part A, 2019, 41, 2906–2916 CrossRef.
- H. N. Chanakya and M. Sreesha, Anaerobic retting of banana and arecanut wastes in a plug flow digester for recovery of fiber, biogas and compost, Energy Sustain. Dev., 2012, 16, 231–235 CrossRef CAS.
- R. A. P. Lomeda-De Mesa, A. N. Soriano, A. R. D. Marquez and A. P. Adornado, Study on the proximate and ultimate analyses and calorific value of coal blending between torrefied biomass from coconut (Cocos nucifera) husk and Semirara coal, IOP Conf. Ser.: Earth Environ. Sci., 2020, 471, 012004 CrossRef.
- J. C. Terrapon-Pfaff, Linking energy- and land-use systems: Energy potentials and environmental risks of using agricultural residues in Tanzania, Sustainability, 2012, 4, 278–293 CrossRef.
- M. M. Manyuchi, C. Mbohwa and E. Muzenda, Biogas and Bio solids production from tea waste through anaerobic digestion, Proc. Int. Conf. Ind. Eng. Oper. Manage., 2018, 2018, 2519–2525 Search PubMed.
- H. Zhang, X. Xiong, J. Zhou and Y. Huang, Characteristics and optimization of anaerobic digestion of tea waste for biogas production, IOP Conf. Ser.: Earth Environ. Sci., 2020, 453, 012036 CrossRef.
- C. A. García, Á. Peña, R. Betancourt and C. A. Cardona, Energetic and environmental assessment of thermochemical and biochemical ways for producing energy from agricultural solid residues: Coffee Cut-Stems case, J. Environ. Manage., 2018, 216, 160–168 CrossRef PubMed.
- S. Garcia-Freites, A. Welfle, A. Lea-Langton, P. Gilbert and P. Thornley, The potential of coffee stems gasification to provide bioenergy for coffee farms: a case study in the Colombian coffee sector, Biomass Convers. Biorefin., 2020, 10, 1137–1152 CrossRef CAS.
- L. Janke, A. Leite, M. Nikolausz, T. Schmidt, J. Liebetrau, M. Nelles and W. Stinner, Biogas Production from Sugarcane Waste: Assessment on Kinetic Challenges for Process Designing, Int. J. Mol. Sci., 2015, 16, 20685–20703 CrossRef CAS PubMed.
- L. M. López González, I. Pereda Reyes, J. Dewulf, J. Budde, M. Heiermann and H. Vervaeren, Effect of liquid hot water pre-treatment on sugarcane press mud methane yield, Bioresour. Technol., 2014, 169, 284–290 CrossRef PubMed.
- L. M. López González, I. Pereda Reyes and O. Romero Romero, Anaerobic co-digestion of sugarcane press mud with vinasse on methane yield, Waste Manage., 2017, 68, 139–145 CrossRef PubMed.
- M. M. El-Shinnawi, M. El-Houssieni, S. A. Aboel-Naga and S. Fahmy, Chemical changes during a two-stage digestion technique for biogas production from combinations of organic wastes, Resour. Conserv. Recycl., 1990, 3, 217–230 CrossRef.
- B. K. Das and S. M. N. Hoque, Assessment of the Potential of Biomass Gasification for Electricity Generation in Bangladesh, J. Renew. Sustain., 2014, 2014, 1–10 Search PubMed.
- J. K. Sarkar and Q. Wang, Characterization of pyrolysis products and kinetic analysis of waste jute stick biomass, Processes, 2020, 8(7), 837 CrossRef CAS.
- N. Acosta, J. de Vrieze, V. Sandoval, D. Sinche, I. Wierinck and K. Rabaey, Cocoa residues as viable biomass for renewable energy production through anaerobic digestion, Bioresour. Technol., 2018, 265, 568–572 CrossRef CAS PubMed.
- F. Monlau, A. Barakat, J. P. Steyer and H. Carrere, Comparison of seven types of thermo-chemical pretreatments on the structural features and anaerobic digestion of sunflower stalks, Bioresour. Technol., 2012, 120, 241–247 CrossRef CAS PubMed.
- S. S. Hashemi, K. Karimi and S. Mirmohamadsadeghi, Hydrothermal pretreatment of safflower straw to enhance biogas production, Energy, 2019, 172, 545–554 CrossRef CAS.
- D. Kovacic, D. Kralik, S. Rupcic, D. Jovicic, R. Spajic and M. Tišmac, Soybean straw, corn stover and sunflower stalk as possible substrates for biogas production in Croatia: A review, Chem. Biochem. Eng. Q., 2017, 31, 187–198 CrossRef CAS.
- J. A. Garcia-Nunez, D. T. Rodriguez, C. A. Fontanilla, N. E. Ramirez, E. E. Silva Lora, C. S. Frear, C. Stockle, J. Amonette and M. Garcia-Perez, Evaluation of alternatives for the evolution of palm oil mills into biorefineries, Biomass Bioenergy, 2016, 95, 310–329 CrossRef CAS.
- S. S. Harsono, P. Grundmann and S. Soebronto, Anaerobic treatment of palm oil mill effluents: Potential contribution to net energy yield and reduction of greenhouse gas emissions from biodiesel production, J. Clean Prod., 2014, 64, 619–627 CrossRef CAS.
- P. Prasertsan, W. Khangkhachit, W. Duangsuwan, C. Mamimin and S. O-Thong, Direct hydrolysis of palm oil mill effluent by xylanase enzyme to enhance biogas production using two-steps thermophilic fermentation under non-sterile condition, Int. J. Hydrog. Energy, 2017, 42, 27759–27766 CrossRef CAS.
- W. Suksong, P. Kongjan, P. Prasertsan, T. Imai and S. O-Thong, Optimization and microbial community analysis for production of biogas from solid waste residues of palm oil mill industry by solid-state anaerobic digestion, Bioresour. Technol., 2016, 214, 166–174 CrossRef CAS PubMed.
- T. Mandang, R. Sinambela and N. R. Pandianuraga, Physical and mechanical characteristics of oil palm leaf and fruits bunch stalks for bio-mulching, IOP Conf. Ser.: Earth Environ. Sci., 2018, 196, 012015 CrossRef.
- T. L. Kelly-Yong, K. T. Lee, A. R. Mohamed and S. Bhatia, Potential of hydrogen from oil palm biomass as a source of renewable energy worldwide, Energy Policy, 2007, 35, 5692–5701 CrossRef.
- S. Sabiha-Hanim, M. A. M. Noor and A. Rosma, Effect of autohydrolysis and enzymatic treatment on oil palm (Elaeis guineensis Jacq.) frond fibres for xylose and xylooligosaccharides production, Bioresour. Technol., 2011, 102, 1234–1239 CrossRef CAS PubMed.
- J. A. Garcia-Nunez, N. E. Ramirez-Contreras, D. T. Rodriguez, E. Silva-Lora, C. S. Frear, C. Stockle and M. Garcia-Perez, Evolution of palm oil mills into bio-refineries: Literature review on current and potential uses of residual biomass and effluents, Resour. Conserv. Recycl., 2016, 110, 99–114 CrossRef.
- S. Ali, T. A. Shah, A. Afzal and R. Tabassum, Analysis of agricultural substrates for nutritive values and biomethane potential, Curr. Sci., 2018, 115, 292–299 CrossRef CAS.
-
G. Bochmann and L. F. R. Montgomery, in The Biogas Handbook: Science, Production and Applications, ed. A. Wellinger, J. Murphy and D. Baxter, Woodhead Publishing, Elsevier Ltd., 2013, pp. 85–103 Search PubMed.
- S. Pérez-Elvira, M. Fdz-Polanco, F. I. Plaza, G. Garralón and F. Fdz-Polanco, Ultrasound pre-treatment for anaerobic digestion improvement, Water Sci. Technol., 2009, 60, 1525–1532 CrossRef PubMed.
- Z. Sapci, The effect of microwave pretreatment on biogas production from agricultural straws, Bioresour. Technol., 2013, 128, 487–494 CrossRef CAS PubMed.
- C. A. García, J. Moncada, V. Aristizábal and C. A. Cardona, Techno-economic and energetic assessment of hydrogen production through gasification in the Colombian context: Coffee Cut-Stems case, Int. J. Hydrog. Energy, 2017, 42, 5849–5864 CrossRef.
- M. Melikoglu and Z. K. Menekse, Forecasting Turkey's cattle and sheep manure based biomethane potentials till 2026, Biomass Bioenergy, 2020, 132, 105440 CrossRef CAS.
- G. K. Kafle and L. Chen, Comparison on batch anaerobic digestion of five different livestock manures and prediction of biochemical methane potential (BMP) using different statistical models, Waste Manage., 2016, 48, 492–502 CrossRef CAS PubMed.
- A. Sowunmi, R. M. Mamone, J. R. Bastidas-Oyanedel and J. E. Schmidt, Biogas potential for electricity generation in the Emirate of Abu Dhabi, Biomass Convers. Biorefin., 2016, 6, 39–47 CrossRef CAS.
- A. Carabeo-Pérez, L. Odales-Bernal, E. López-Dávila and J. Jiménez, Biomethane potential from herbivorous animal's manures: Cuban case study, J. Mater. Cycles Waste Manag., 2021, 23, 1404–1411 CrossRef.
- A. Orangun, H. Kaur and R. R. Kommalapati, Batch anaerobic co-digestion and biochemical methane potential analysis of goat manure and food waste, Energies, 2021, 14, 1–14 CrossRef.
- S. Kusch, H. Oechsner and T. Jungbluth, Biogas production with horse dung in solid-phase digestion systems, Bioresour. Technol., 2008, 99, 1280–1292 CrossRef CAS PubMed.
-
The University of Edinburgh, Donkey Fact Sheet, https://www.ed.ac.uk/files/imports/fileManager/donkeyfactsheet.pdf Search PubMed.
-
M. Ward and P. S. nee’ Nigam, in Biotechnology for Agro-Industrial Residues Utilisation, ed. P. S. nee’ Nigam and A. Pandey, Springer, 1st edn, 2009, pp. 417–430 Search PubMed.
- J. R. Bastidas-Oyanedel, A. Sowunmi and J. E. Schmidt, Valorization of Arid Region Abattoir Animal Waste: Determination of Biomethane Potential, Waste Biomass Valorization, 2018, 9, 2327–2335 CrossRef CAS.
- A. Shanableh, M. Abdallah, A. Tayara, C. Ghenai, M. Kamil, A. Inayat and A. Shabib, Experimental characterization and assessment of bio- and thermo-chemical energy potential of dromedary manure, Biomass Bioenergy, 2021, 148, 106058 CrossRef CAS.
- V. Burg, G. Bowman, M. Haubensak, U. Baier and O. Thees, Valorization of an untapped resource: Energy and greenhouse gas emissions benefits of converting manure to biogas through anaerobic digestion, Resour. Conserv. Recycl., 2018, 136, 53–62 CrossRef CAS.
- T. K. Debnath, M. Pal, P. K. Roy and J. Pal, Analysis of Physical Composition of Municipal Solid Waste of Agartala City, Int. Res. J. Adv. Eng. Sci., 2017, 2, 87–89 Search PubMed.
- A. Singh, J. Zaidi, D. Bajpai, G. Sharma, A. Yadav, D. S. Chauhan and S. Ganesh, Municipal solid waste management challenges and health risk problematic solutions at Agra city, U. P., India, Adv. Appl. Sci. Res, 2014, 5, 397–403 Search PubMed.
- G. S. Manjunatha, D. Chavan, P. Lakshmikanthan, R. Swamy and S. Kumar, Estimation of heat generation and consequent temperature rise from nutrients like carbohydrates, proteins and fats in municipal solid waste landfills in India, Sci. Total Environ., 2020, 707, 135610 CrossRef CAS PubMed.
- J. Sheth, K. Patel, E. Engineers and D. Shah, Solid Waste Management: A Case Study of Malkapur City, Int. J. Sci. Res., 2015, 4, 2146–2152 Search PubMed.
- S. Kumar, H. Dhar, V. v. Nair, J. K. Bhattacharyya, A. N. Vaidya and A. B. Akolkar, Characterization of municipal solid waste in high-altitude sub-tropical regions, Environ. Technol., 2016, 37, 2627–2637 CrossRef CAS PubMed.
- M. Sharholy, K. Ahmad, R. C. Vaishya and R. D. Gupta, Municipal solid waste characteristics and management in Allahabad, India, Waste Manage., 2007, 27, 490–496 CrossRef PubMed.
- S. Haldar, Present Status of Solid Waste Management System in Asansol Municipal Corporation, IOSR j. humanit soc. sci., 2015, 20, 31–36 Search PubMed.
- R. B. Katiyar, S. Suresh and A. K. Sharma, ICGSEE-2013[14th–16th March 2013] International Conference on Global Scenario in Environment and Energy Characterisation Of Municipal Solid Waste Generated By The City Of Bhopal, India, Int.
J. Chemtech Res., 2013, 5, 623–628 CAS.
- R. Rana, R. Ganguly and A. K. Gupta, Physico-chemical characterization of municipal solid waste from Tricity region of Northern India: a case study, J. Mater. Cycles Waste Manag., 2018, 20, 678–689 CrossRef CAS.
- R. Rana, R. Ganguly and A. K. Gupta, Life-cycle assessment of municipal solid-waste management strategies in Tricity region of India, J. Mater. Cycles Waste Manag., 2019, 21, 606–623 CrossRef.
- A. E. Peter, S. M. Shiva Nagendra and I. M. Nambi, Environmental burden by an open dumpsite in urban India, Waste Manage., 2019, 85, 151–163 CrossRef CAS PubMed.
- S. P. Jeyapriya, M. K. Saseetharan and T. Nadu, Study on Municipal Solid Waste Refuse Characteristics and Leachate Samples of Coimbatore City, Nat. Environ. Pollut. Technol., 2007, 6, 149–152 CAS.
- S. Rath, Status and implementing strategies of municipal solid waste management in the millennium City of Cuttack (Odisha, India), Afr. J. Environ. Sci. Tech., 2020 DOI:10.5897/AJEST2018.xxxx.
- M. Chakraborty, C. Sharma, J. Pandey and P. K. Gupta, Assessment of energy generation potentials of MSW in Delhi under different technological options, Energy Convers. Manag., 2013, 75, 249–255 CrossRef.
- S. Mor, K. Ravindra, A. de Visscher, R. P. Dahiya and A. Chandra, Municipal solid waste characterization and its assessment for potential methane generation: A case study, Sci. Total Environ., 2006, 371, 1–10 CrossRef CAS PubMed.
- V. Srivastava, B. Vaish, R. P. Singh and P. Singh, An insight to municipal solid waste management of Varanasi city, India, and appraisal of vermicomposting as its efficient management approach, Environ. Monit. Assess., 2020, 192, 191 CrossRef CAS PubMed.
- S. Suthar and P. Singh, Household solid waste generation and composition in different family size and socio-economic groups: A case study, Sustain. Cities Soc., 2015, 14, 56–63 CrossRef.
- D. Mboowa, S. Quereshi, C. Bhattacharjee, K. Tonny and S. Dutta, Qualitative determination of energy potential and methane generation from municipal solid waste (MSW) in Dhanbad (India), Energy, 2017, 123, 386–391 CrossRef CAS.
- M. Singh and G. Leena, Forecasting of waste-to-energy system: A case study of Faridabad, India, Energy Sources, Part A, 2020, 42, 319–328 CrossRef.
- R. M. Sebastian, D. Kumar and B. J. Alappat, Easy Estimation of Mixed Municipal Solid Waste Characteristics from Component Analysis, J. Environ. Eng., 2019, 145, 04019081 CrossRef CAS.
- S. S. Chauhan and P. D. Rastogi, Utilization of Municipal Solid Waste of Gwalior City for Energy Generation (A Case Study), Int. Res. J. Eng. Technol., 2016, 3, 1986–1990 Search PubMed.
- S. Jain and M. P. Sharma, Power generation from MSW of Haridwar city: A feasibility study, Renewable Sustainable Energy Rev., 2011, 15, 69–90 CrossRef.
- S. Jampala, A. Gellu and D. S. Kala, Current Scenario on Urban Solid Waste with Respect to Hyderabad City, IJRSSET, 2016, 3, 10 Search PubMed.
-
K. Kapadia and A. Agrawal, in Proceedings of Recent Advances in Interdisciplinary Trends in Engineering & Applications (RAITEA) 2019, SSRN-Elsevier, 2019 Search PubMed.
- S. Sethi, N. C. Kothiyal, A. K. Nema and M. K. Kaushik, Characterization of Municipal Solid Waste in Jalandhar City, Punjab, India, J. Hazard Toxic Radioact. Waste, 2013, 17, 97–106 CrossRef CAS.
-
S. Rajesh, in Geotechnics for Natural and Engineered Sustainable Technologies, ed. A. Murali Krishna, A. Dey and S. Sreedeep, Springer Nature Singapore Pte Ltd., Singapore, 1st edn, 2018, pp. 291–302 Search PubMed.
-
S. Das and B. Bhattacharya, in The 19th International Conference on Industrial Engineering and Engineering Management, ed. E. Qi, J. Shen and R. Dou, Springer, 1st edn, 2013, pp. 1399–1409 Search PubMed.
- R. M. Sebastian, D. Kumar and B. Alappat, Variation in incinerability of municipal solid waste in Indian cities, Int. J. Sci. Eng., 2017, 2, 9–14 Search PubMed.
- Y. D. Mehta, Y. Shastri and B. Joseph, Economic analysis and life cycle impact assessment of
municipal solid waste (MSW) disposal: A case study of Mumbai, India, Waste Manag. Res., 2018, 36, 1177–1189 CrossRef PubMed.
- B. K. Sharma and M. K. Chandel, Life cycle cost analysis of municipal solid waste management scenarios for Mumbai, India, Waste Manag., 2021, 124, 293–302 CrossRef PubMed.
- M. Endait and S. Patil, Laboratory investigation of compaction characteristics of fresh and degraded municipal solid waste, Waste Dispos. Sustain. Energy, 2020, 2, 305–312 CrossRef.
- R. V. Singh, Quantification of municipal solid waste for Patna city, Int. J. Environ. Technol. Manag., 2012, 15, 516–523 CrossRef.
-
T. S. Khambekar and S. T. Mali, in Techno-Societal 2020: Proceedings of the 3rd International Conference on Advanced Technologies for Societal Applications—Volume 2, ed. P. M. Pawar, R. Balasubramaniam, B. P. Ronge, S. B. Salunkhe, A. S. Vibhute and B. Melinamath, Springer Nature Switzerland, Cham, Switzerland, 1st edn, 2020 Search PubMed.
- S. Tanaji Mali, K. C. Khare and A. H. Biradar, Characterisation of municipal solid waste at landfill, India, Proc. Inst. Civ. Eng.: Waste Resour. Manag., 2011, 164, 247–255 Search PubMed.
- T. Alam and K. Kulkarni, Municipal Solid Waste Management and its Energy Potential in Roorkee City, Uttarakhand, India, J. Inst. Eng. (India): A, 2016, 97, 9–17 CAS.
- S. N. Thitame, G. M. Pondhe and D. C. Meshram, Characterisation and composition of Municipal Solid Waste (MSW) generated in Sangamner City, District Ahmednagar, Maharashtra, India, Environ. Monit. Assess., 2010, 170, 1–5 CrossRef CAS PubMed.
- J. Mushtaq, A. Q. Dar and N. Ahsan, Spatial–temporal variations and forecasting analysis of municipal solid waste in the mountainous city of north-western Himalayas, SN Appl. Sci., 2020, 2, 1–18 Search PubMed.
- M. Vinodbhai Mewada, S. Albert, M. Mewada and A. Padhiar, Municipal Solid Waste Management System in Vadodara City: Current Scenario Municipal Solid waste characterization and its assessment for fungal bio remediation View project Municipal Solid Waste Management System in Vadodara City: Current Scenario, IOSR J. Environ. Sci., 2020, 14, 45–50 Search PubMed.
- A. N. Srivastava and S. Chakma, Quantification of landfill gas generation and energy recovery estimation from the municipal solid waste landfill sites of Delhi, India, Energy Sources, Part A, 2020, 1–14 Search PubMed.
- M. Chakraborty, C. Sharma, J. Pandey, N. Singh and P. K. Gupta, Methane emission estimation from landfills in Delhi: A comparative assessment of different methodologies, Atmos. Environ., 2011, 45, 7135–7142 CrossRef CAS.
- A. K. Jha, C. Sharma, N. Singh, R. Ramesh, R. Purvaja and P. K. Gupta, Greenhouse gas emissions from municipal solid waste management in Indian mega-cities: A case study of Chennai landfill sites, Chemosphere, 2008, 71, 750–758 CrossRef CAS PubMed.
- M. Gollapalli and S. H. Kota, Methane emissions from a landfill in north-east India: Performance of various landfill gas emission models, Environ. Pollut., 2018, 234, 174–180 CrossRef CAS PubMed.
|
This journal is © The Royal Society of Chemistry 2023 |