ITIC derivative acceptors for ternary organic solar cells: fine-tuning of absorption bands, LUMO energy levels, and cascade charge transfer†
Received
13th September 2021
, Accepted 15th November 2021
First published on 17th November 2021
Abstract
For effective supplementary acceptor molecules (A2) in ternary organic solar cell (TOSC) devices, a series of ITIC derivatives were designed and synthesized by incorporating symmetrically or asymmetrically functional termini of various electron-accepting 1,3-indanedione (IND) groups having distinct different electron affinities to the fused core unit, indacenodithieno[3,2-b]thiophene (IDTT). The absorption, thermal properties and LUMO energy level of the prepared ITIC derivatives were precisely controlled according to the terminal group as well as the open-circuit voltage (VOC) in binary organic solar cells (BOSCs) employing PBDB-T as a host donor polymer and ITIC derivatives as a host acceptor material. In the PBDB-T:ITIC:A2-based ternary system, simply adding ITIC derivatives, which can form a cascade energy alignment between the LUMO levels of the host PBDB-T donor polymer and ITIC acceptor molecule, as a small amount (approximately 5%) of A2 acceptor molecules improved the photoelectric efficiency by up to 10% more than that of a PBDB-T:ITIC binary system of the same structure through enhancing VOC, JSC, and FF, synchronously. These results offer a route to find the selection criteria for A2 acceptor molecules suitable for effective use in TOSC devices and develop high-performance TOSC devices.
Introduction
Although organic solar cells (OSCs) have made significant progress, additional improvement in the efficiency and stability of OSC devices is required for commercialization. Hence, recently, studies have been actively conducted to develop a ternary OSC (TOSC) device with supplementary donor or acceptor molecules added to a binary OSC (BOSC) system.1–3 TOSCs can combine the strengths of both tandem OSCs and BOSCs, i.e., TOSCs can improve further the photon harvesting efficiency by employing third components, also keeping the simple processing of single-junction devices. In a TOSC device system, so far, most efforts have been made to improve the efficiency of TOSC devices by finding appropriate ternary material systems and to understand the working mechanism of TOSCs.1–3 To date, cascade charge transfer,4–7 energy transfer,8–11 parallel-linkage structures,12–14 and alloy models15–17 have been suggested as possible driving mechanisms for TOSC devices; however, owing to insufficient study on TOSC devices, research to identify their driving mechanism remains rudimentary. Nevertheless, clarifying the working mechanism of TOSC devices is crucial to developing a superior TOSC device in the long run. Therefore, a more diverse library of TOSC devices should be established in the future.
Recently, among TOSC devices, the D:A1:A2 TOSC device, where D is the donor and A1 and A2 are acceptors, using two types of acceptor molecules has received enormous attention.2,18–21 The second acceptor (A2) molecule used can play multiple roles of (i) increasing the effect of additional light absorption, (ii) enhancing the effect of open circuit voltage (VOC) adjustment and charge transfer through an appropriate energy level (especially, lowest unoccupied molecular orbital (LUMO) energy level) alignment, and (iii) optimizing the morphology of the blend film, thereby improving the efficiency of the TOSC device. As mentioned earlier, however, owing to the lack of understanding of the driving mechanism of TOSC devices, selection criteria for a suitable A2 acceptor molecule are still unclear.2,3
In the early D:A1:A2 TOSC system, fullerene derivatives were widely used as A2 molecules.1,2 However, recently, non-fullerene acceptor (NFA) derivatives have received considerable attention for their high superiority as A2 acceptor molecules.2,3,20–22 Among the related NFA molecules, ITIC derivatives can be appreciated as a good candidate.2,3,23–25 ITIC is referred to as one of the most excellent acceptor molecules for BOSC devices since it has both high light-absorbing properties and excellent charge transfer properties owing to strong intermolecular interaction. ITIC has an A–D–A structure and its backbone is composed of a fused core as a donor unit, indacenodithieno[3,2-b]thiophene (IDTT or IDDT), and two terminal 1,3-indanedione (IND) derivative units with an excellent electron affinity, 2-(3-oxo-2,3-dihydro-1H-inden-1-ylidene)malononitrile (IC), as acceptor units.23 However, so far, ITIC and its derivatives have been primarily developed as host acceptor molecules used in BOSC devices.23–26 Therefore, for ITIC derivatives to also be used as A2 acceptor molecules of TOSC devices, more kinds of superior ITIC derivatives that can perform the abovementioned roles of the A2 acceptor molecules are needed to be developed and investigated in more detail.
Very recently, along with ITIC, Y6 has been introduced as one of the most efficient NFA materials for OSCs.26 Y6 consists of an A–DA′D–A structure where an electron deficient-core-based central fused ring with a benzothiadiazole unit is employed, and exhibited a very high photoelectric efficiency of 15.7% in BOSCs paired with the PM6 donor polymer.27 However, owing to the very complicated chemical structure, up to 10 steps are required to prepare Y6 molecules from the starting compound,27,28 which inevitably leads to a complex and high cost synthesis process. This is not only a major obstacle for the practical commercialization of OSC devices, but also makes it difficult to readily develop various types of Y6 derivatives compared to the ITIC series. Therefore, in research to systematically find efficient A2 acceptor molecules for TOSCs via a trial-and-error method using various candidate molecules, it is considered that ITIC derivatives are more suitable than the Y6 series.
In consideration of these things, in this study, we tried to develop various ITIC derivatives that can be used as effective A2 acceptor molecules in TOSC devices, especially where ITIC molecules are used as a host acceptor (A1) molecule. To this end, it was assumed that the target ITIC derivatives should have a chemical structure similar to that of ITIC so that they may have mutually good compatibility, and eventually prevent “recombination centers” or “morphological traps” in the ternary active layer.2,29,30 Thus, we fixed the largest and central part of the ITIC molecule, the electron-donating IDTT unit. In addition, in order to precisely regulate the light absorption region and LUMO energy level of the target compounds which affect profoundly the performance of the TOSC devices,23,31–34 several types of IND derivatives with sufficiently different electron affinities were introduced in various combinations at the terminal end of the IDTT core unit. Therefore, the electron affinity of the IND derivatives introduced at the terminal end was increased by gradually introducing an element or a functional group with a high electron-accepting effect into the IND unit.
To achieve this purpose, we prepared benzo[b]thiophen-3(2H)-one 1,1-dioxide (BTD) (one ketone group of IND is substituted with a –SO2 unit), 5-fluorobenzo[b]thiophen-3(2H)-one 1,1-dioxide (BTDF) (fluorine is introduced into the phenyl group of the BTD unit), 2-(3-oxo-2,3-dihydro-1H-inden-1-ylidene)malononitrile (IC) (also so-called ‘INCN’, one ketone of IND is substituted with a –(CN)2 unit), and 2-(1,1-dioxidobenzo[b]thiophen-3(2H)-ylidene)malononitrile (ICS) (the remaining ketone of IC is substituted with a –SO2 unit) as terminal end groups (Fig. 1). Finally, we introduced these IND units symmetrically or asymmetrically at both terminal ends of the IDTT central unit and thus successfully synthesized various target symmetric and asymmetric ITIC derivatives (Fig. 1). Except ITIC and ITICS,35 all the prepared ITIC derivatives are firstly reported for this study. Especially, the prepared asymmetric ITIC derivatives are unique in that there are only a few efforts to develop asymmetric ITIC analogues by terminal end group modulation owing to the complexity and difficulty of the synthesis.36–38 Until now, most conventional asymmetric ITIC derivatives have introduced asymmetric parts into the central core unit instead of the terminal end group.39–45 Moreover, given that the optical properties and the LUMO energy level of ITIC derivatives are strongly influenced by the terminal end groups, for this study, these asymmetric molecules comprising two different terminal end groups are expected to be more useful than the previous asymmetric ITIC derivatives in which the asymmetric core units are employed.
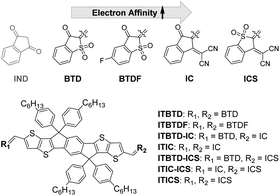 |
| Fig. 1 Chemical structures of terminal IND units and symmetric/asymmetric ITIC derivatives. | |
In this study, we minutely and systematically analyzed the thermal, optical, and electrochemical properties, energy levels, and BOSC device characteristics of the prepared ITIC derivatives according to the terminal group. Based on these results, we prepared PBDB-T:ITIC:A2-based TOSC devices using the selected ITIC derivatives, and evaluated their functions and roles as A2 acceptor molecules. In particular, we realized that by simply adding a small amount, approximately 5% (wt), of the selected A2 acceptor molecules that can form a cascade energy alignment between the LUMO energy levels of the PBDB-T donor polymer and ITIC acceptor molecule, regardless of the symmetric or asymmetric nature, could promote the effective charge transfer, and thus enhance the power conversion efficiency by up to 10% compared with PBDB-T:ITIC-based BOSC devices of the same structure.
Results and discussion
Electron affinity of 1,3-indanedione (IND)-type end groups
We prepared IND derivative units (BTD, BTDF, IC, and ICS) (Scheme S1 and see the ESI for details†) to be introduced at the terminal end of an IDTT core and compared the electron affinity strength of these molecules through cyclic voltammetry (CV) measurements and density functional theory (DFT) calculations. From the measurement of the reduction potentials of BTD, BTDF, IC, and ICS units via CV, we found that the reduction potential values gradually reduced in the order of BTD < BTDF < IC < ICS at constant gaps (Table S1 and Fig. S1†). Furthermore, in the LUMO energy level analysis obtained from the DFT calculations, we confirmed that the LUMO levels of the IND derivatives deepened in the same order as the reduction potential values (Table S1†) and the reduction potential and LUMO levels are highly correlated (Fig. 2), which demonstrates that the fine-tuning of the LUMO energy level of IND derivatives can be achieved by incorporating the electron accepting element or functional group. From these results, the electron affinity strength of the IND derivatives was, as expected, in the BTD < BTDF < IC < ICS order, and the difference in strength was almost constant. Therefore, we expected to effectively control the light-absorbing properties and LUMO levels of the target ITIC derivatives by varying the type and the introduction method (symmetric and asymmetric) of the IND derivatives introduced at the terminal end of the IDTT central unit.
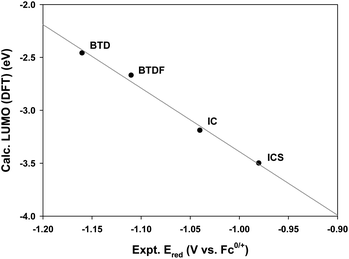 |
| Fig. 2 Correlation plot of the LUMO energy levels of IND-type electron withdrawing groups (BTD, BTDF, IC, and ICS) as determined using DFT calculations (B3LYP/6-31G*) and their reduction potentials as determined using cyclic voltammetry. | |
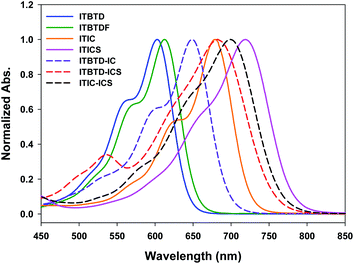 |
| Fig. 3 UV/vis absorption spectra of symmetric and asymmetric ITIC derivatives in CHCl3. | |
Synthesis of symmetric and asymmetric ITIC derivatives
The target ITIC derivatives were prepared by symmetrically and asymmetrically introducing the IND derivatives (BTD, BTDF, IC, and ICS) into IDTT central units (Scheme 1 and see the ESI for details†). The chemical structure of the ITIC derivatives was fully confirmed via1H-NMR, 19F-NMR, FT-IR, mass, and elemental analysis. We prepared symmetric ITIC derivatives, namely, ITBTD, ITBTDF, ITIC, and ITICS compounds, respectively, by introducing BTD, BTDF, IC, and ICS units on both sides of the IDTT-CHO compound by Knoevenagel condensation under certain base conditions. The higher the electron affinity of the IND derivative units introduced, the lower the basicity of the base catalyst used (i.e., piperidine for ITBTD & ITBTDF, and pyridine for ITIC). Even in the case of the synthesis of ITICS, where the ICS units with the largest electron affinity were introduced, no base catalyst was used and the reaction was carried out only under Ac2O solvent conditions.
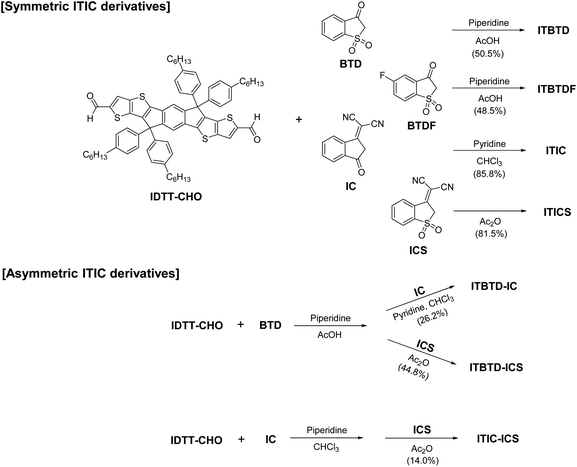 |
| Scheme 1 Synthetic routes of symmetric and asymmetric ITIC derivatives. | |
For asymmetric ITIC derivatives, namely, ITBTD-IC, ITBTD-IC, and ITIC-ICS, unlike symmetric ITIC derivatives, we used a method of introducing the terminal unit with lower electron affinity first into the IDTT-CHO central unit and then gradationally introducing the other terminal unit with higher electron affinities. This was because, when we first introduced the terminal unit with higher electron affinities, such as IC or ICS, obtaining intermediates with the terminal unit introduced on only one side of the central IDTT unit was difficult, and mostly the intermediates with the terminal unit introduced on both sides of the central IDTT unit were obtained. Therefore, for ITBTD-IC and ITBTD-ICS, after introducing the BTD unit on one side, the IC and ICS units were introduced sequentially into the intermediates, respectively. In the same manner, for ITIC-ICS, the introduction of the IC unit into the IDTT central unit was followed by introducing the ICS unit into the intermediate. However, the reaction yield for ITIC-ICS (14.0%) was lower compared to that of ITBTD-IC and ITBTD-ICS (26.2 and 44.8%, respectively) owing to the lower production yield of the intermediate which has the IC unit introduced on one side of the IDTT central unit. All synthesized ITIC derivatives had excellent solubility in general organic solvents, such as dichloromethane, chloroform, and chlorobenzene.
Thermal properties
All the synthesized ITIC derivatives had high thermal stability to the extent that they were suitable for use in OSC devices owing to the rigid structure of the IDTT-CHO central unit, but the thermal properties depended on the introduced terminal IND derivative type or the symmetry/asymmetry in the structure (Table 1, Fig. S2 and S3†). The symmetric ITIC derivative exhibited no specific thermal transition up to 250 °C on differential scanning calorimetry (DSC) (Table 1 and Fig. S2†) and had the thermal decomposition temperature at 5% weight loss (Td) above 330 °C (Table 1 and Fig. S3(a)†). As the electron-accepting strength of the terminal IND derivatives increased, the Td increased. However, ITBTD-F showed a slightly lower Td than ITBTD, despite a higher electron-accepting strength than ITBTD, because the fluorine-substituted aromatic compounds exhibit lower thermal stability compared to hydrogen analogs.46,47
Table 1 Summary of the thermal, optical and electrochemical properties of ITIC derivatives
Compound |
T
d
[°C] |
λ
max
[nm] |
ε
max
[M−1 cm−1] |
E
ox
[V] |
E
red
[V] |
E
HOMO
[eV] |
E
LUMO
[eV] |
The decomposition temperature at 5% weight loss obtained from TGA measurements with a heating rate of 10 °C min−1 under N2.
Measured in CHCl3 solution.
Onset potential vs. Fc/Fc+.
Calculated using the equation of EHOMO/ELUMO = −(4.80 + Eoxonset/Eredonset) eV.
|
ITBTD |
336 |
602 |
1.87 × 105 |
+0.68 |
−1.17 |
−5.48 |
−3.63 |
ITBTDF |
325 |
612 |
1.56 × 105 |
+0.70 |
−1.11 |
−5.50 |
−3.69 |
ITIC |
351 |
679 |
1.89 × 105 |
+0.67 |
−0.95 |
−5.47 |
−3.85 |
ITICS |
372 |
719 |
1.85 × 105 |
+0.73 |
−0.77 |
−5.53 |
−4.03 |
ITBTD-IC |
326 |
648 |
1.64 × 105 |
+0.67 |
−1.02 |
−5.47 |
−3.78 |
ITBTD-ICS |
329 |
683 |
1.16 × 105 |
+0.69 |
−0.81 |
−5.49 |
−3.99 |
ITIC-ICS |
334 |
699 |
1.44 × 105 |
+0.68 |
−0.78 |
−5.48 |
−4.02 |
In all asymmetric derivatives, no melting points were detected up to 250 °C (Fig. S2†), and they had Td above 320 °C (Table 1 and Fig. S3(b)†). However, their average Td was lower than that of the symmetric derivatives, and like the symmetric derivatives, as the overall electron-accepting strength of the terminal IND derivatives increased, the Td also increased.
Photophysical properties
We could ascertain that, depending on the type of terminal IND derivative and the symmetric/asymmetric introduction method, the maximum absorption wavelength of the ITIC derivatives was finely regulated over a wide range of 600–720 nm (Fig. 3). In the symmetric ITIC derivatives, the maximum absorption band of ITIC derivatives shifted toward the long-wavelength region as the electron-accepting strength of the terminal IND derivatives increased. This is because the intramolecular charge transfer (ICT) process in the ITIC molecule, in which charge transfers from the HOMO level of the electron-donating IDTT unit to the LUMO level of the electron-accepting IND derivative,31,48–50 depends on the electron-accepting strength of the IND unit, that is, the stronger the electron-accepting effect in an IND unit the lower the LUMO in the IND, reducing the ICT absorption bandgap in the resulting ITIC derivatives.
The maximum absorption band of asymmetric ITIC derivatives having terminal IND units of different electron-accepting strength was located between the maximum absorption bands of two ITIC derivatives whose terminal units were introduced symmetrically. This means that, in the asymmetric ITIC derivatives, the ICT process from the IDTT-CHO central unit to the terminal IND unit does not occur only exclusively in the single terminal IND unit with a high electron-accepting strength but also in the IND unit with a low electron-accepting strength, i.e., all of which affect the ICT absorption band of ITIC derivatives.51 The light absorption properties of ITIC derivatives in the solution were the same as those in the film state (Fig. S4, S5 and Table S2†).
Electrochemical properties & energy levels
Using the results obtained from electrochemical analysis (Table 1 and Fig. 4), we calculated the HOMO and LUMO energy levels of ITIC derivatives. From the calculations, it was confirmed that all HOMO levels of ITIC derivatives are similar, whereas the LUMO levels are finely modulated depending on the type of terminal IND derivative and symmetry of their introduction (Table 1 and Fig. 5). For the symmetric ITIC derivatives, the greater the electron-accepting effect of the terminal IND derivatives, the deeper the LUMO level (ITBTD > ITBTDF > ITIC > ITICS). For the asymmetric ITIC derivatives with different terminal IND units introduced, the LUMO level decreased with the increase in the sum of electron-accepting strength of both terminal IND units (ITBTD-IC > ITBTD-ICS > ITIC-ICS); in addition, they were located between the LUMO levels of the two ITIC derivatives with mutually different terminal units introduced symmetrically, for example, ITBTD > ITBTD-IC > ITIC. This showed that, as the ICT process, the LUMO levels of the asymmetric ITIC derivatives were undetermined by one IND terminal unit with large electron-accepting strength but by all mutually different terminal IND units. These results are because in HOMO states, most electrons are mainly delocalized in IDTT-CHO central units, whereas electrons extend to terminal IND derivative units to be delocalized in LUMO states.52,53 This was also confirmed by changes in electron cloud density through DFT calculations. For the symmetric ITIC derivatives, electrons were equally concentrated in the same terminal IND units on both sides at the LUMO and LUMO+1 levels (Fig. S6†). However, in the LUMO of the asymmetric ITIC derivatives, electron clouds were concentrically delocalized in the IND terminal unit with a great electron-accepting effect, whereas, in the LUMO+1 level, electron clouds were concentrated primarily in the IND unit with relatively low electron-accepting strength (Fig. S7†). It is also noteworthy that the experimental LUMO levels of ITIC derivatives obtained through solution CV measurements are highly correlated with theoretical LUMO levels obtained through DFT calculations (Fig. 5), and these HOMO and LUMO levels of the ITIC derivatives tend to be the same in film states (Table S2 and Fig. S5†).
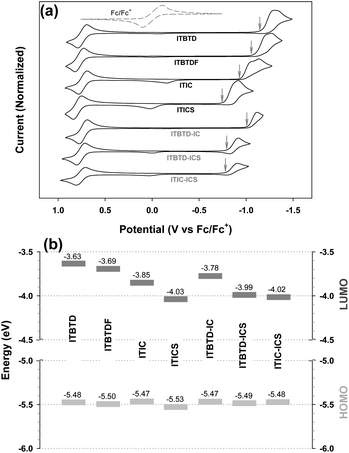 |
| Fig. 4 (a) Cyclic voltammograms of ITIC derivatives in the solution of CH2Cl2. (b) HOMO and LUMO energy levels of ITIC derivatives calculated using the equation of EHOMO/ELUMO = −(4.80 + Eoxonset/Eredonset) eV. | |
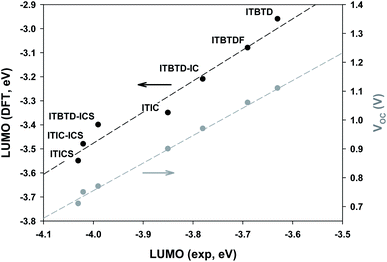 |
| Fig. 5 Black circles: correlation plot of the LUMO energy levels of ITIC derivatives as determined using their reduction potentials and DFT calculations (B3LYP/6-31G*). Grey circles: correlation plot of the LUMO energy levels of ITIC derivatives as determined using their reduction potentials and open-circuit voltages (Voc) obtained from PBDB-T: acceptor-based binary solar cells. | |
Binary device photovoltaic properties
To examine the basic function of ITIC derivatives as solar cell acceptors, we fabricated conventional BOSC devices using PBDB-T donor polymer, which is one of the successful modeling donor polymers paired with ITIC,54 and investigated their solar cell properties (Table 2 and Fig. S8†). The VOC of the solar cells was between 0.75 and 1.11 V, and followed the same trend as the LUMO level of ITIC derivatives (Table 2 and Fig. 5). This would be a textbook example showing that the VOC of an OSC is typically determined by the difference between the HOMO level of the donor polymer and the LUMO level of the acceptor molecule.55–57 Additionally, it can be said that the energy loss generated by these devices also appears to be similar (Table S3†).
Table 2 Photovoltaic parameters of the PBDB-T:acceptor-based binary solar cells
Acceptora |
V
OC [V] |
J
SC [mA cm−2] |
FF |
PCEave (max) [%] |
All the devices were thermally annealed at 100 °C.
|
ITBTD |
1.11 |
5.99 |
0.37 |
2.36 (2.66) |
ITBTDF |
1.06 |
8.67 |
0.41 |
3.73 (3.81) |
ITIC |
0.90 |
14.93 |
0.67 |
8.96 (9.05) |
ITICS |
0.71 |
9.42 |
0.36 |
2.39 (2.80) |
ITBTD-IC |
0.97 |
10.53 |
0.42 |
4.34 (4.60) |
ITBTD-ICS |
0.77 |
5.23 |
0.34 |
1.37 (1.47) |
ITIC-ICS |
0.75 |
9.26 |
0.37 |
2.54 (2.61) |
In terms of device efficiency, derivatives other than ITIC molecules had low overall photoelectric efficiencies due to low Jsc and FF, even if they had high VOC (for ITBTD, ITBTDF, and ITBTD-IC) or a similar light absorption range (for ITICS and ITIC-ICS) compared with ITIC. The main reason for the low efficiency is believed to be due to the bulky steric effects of BTD and ICS units introduced at the terminal end of the ITIC derivatives, resulting in lower intermolecular interactions of these molecules. In other words, the IC terminal group of ITICs can induce high intermolecular interactions due to their planar structure,23–26 whereas the –S
O functional groups of BTD and ICS groups are placed perpendicular to the plane in terminal IND units (Fig. S9†), which disturbs the intermolecular interaction with neighboring molecules. This is presumed to result in preventing the formation of an effective charge transport channel for ITIC derivatives, which adversely affects charge mobility. Through space-charge-limited-current (SCLC) measurement, it was experimentally found that all ITIC derivative-based devices have lower hole and electron mobility than ITIC-based devices; in particular, their electron mobility is significantly lower than that of ITIC-based devices (Table S4†). Because of this, furthermore, ITIC-based devices had balanced hole and electron mobility while other ITIC derivative-based devices did not.
Ternary device photovoltaic properties
We evaluated the photovoltaic properties of the ternary OSC devices using the prepared ITIC derivatives as a third component with the same structure as the binary OSC device system, aiming to use the synthesized ITIC derivatives as the A2 acceptor molecules and obtain higher performance than the ITIC-based BOSC devices (Table 3). Thus, we fabricated PBDB-T:ITIC:A2 ternary devices after selecting ITBTD, ITBTDF, ITBTD-IC, and ITIC-ICS molecules as A2 acceptor molecules, considering the essential role of A2 acceptor molecules in the TOSC, light absorption enhancement and appropriate energy level alignment (Fig. 6 and S10†). In addition, it is here worth mentioning that the selected ITIC derivatives (as A2 acceptors) and ITIC (as a host acceptor) have almost the same HOMO level but different LUMO levels, so we can clearly investigate the effects of the A2 acceptor molecules with different LUMO levels.
Table 3 Photovoltaic parameters of the PBDB-T:ITIC:A2 acceptor-based ternary solar cellsa
Donor (D) |
Acceptors (A1 : A2)b |
D–A blend ratio (D : A1 : A2) (wt) |
V
OC (V) |
J
SC (mA cm−2) |
FF |
PCEave (max) (%) |
PCE change rateb (%) |
All the devices were thermally annealed at 120–130 °C.
Calculated the PCE change rate using [(PCET − PCEb)/(PCEb)] × 100 (%), PCET: average conversion efficiency of PBDB-T:ITIC:A2-based ternary devices, and PCEB: average conversion efficiency of PBDB-T:ITIC binary devices.
|
PBDB-T |
ITIC |
1.00 : 1.00 : 0.00 |
0.896 |
15.01 |
0.66 |
8.90 (9.32) |
0.00 |
ITIC:ITBTD |
1.00 : 0.80 : 0.20 |
0.921 |
14.07 |
0.57 |
7.41 (7.81) |
−16.74 |
1.00 : 0.90 : 0.10 |
0.917 |
14.58 |
0.63 |
8.36 (8.94) |
−6.07 |
1.00 : 0.95 : 0.05 |
0.926 |
15.20 |
0.69 |
9.71 (10.11) |
+9.10 |
ITIC:ITBTDF |
1.00 : 0.80 : 0.20 |
0.934 |
14.79 |
0.62 |
8.59 (9.60) |
−3.48 |
1.00 : 0.90 : 0.10 |
0.944 |
15.32 |
0.66 |
9.56 (9.80) |
+7.42 |
1.00 : 0.95 : 0.05 |
0.933 |
15.15 |
0.69 |
9.79 (10.03) |
+10.00 |
ITIC:ITBTD-IC |
1.00 : 0.80 : 0.20 |
0.916 |
13.89 |
0.56 |
7.15 (8.05) |
−19.66 |
1.00 : 0.90 : 0.10 |
0.908 |
14.55 |
0.64 |
8.40 (9.01) |
−5.62 |
1.00 : 0.95 : 0.05 |
0.923 |
15.28 |
0.69 |
9.69 (10.04) |
+8.88 |
ITIC:ITIC-ICS |
1.00 : 0.80 : 0.20 |
0.854 |
13.81 |
0.46 |
5.40 (5.66) |
−39.33 |
1.00 : 0.90 : 0.10 |
0.887 |
15.10 |
0.58 |
7.79 (8.13) |
−12.47 |
1.00 : 0.95 : 0.05 |
0.893 |
15.29 |
0.60 |
8.13 (8.92) |
−8.65 |
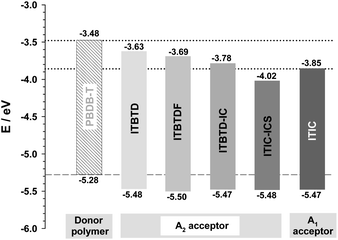 |
| Fig. 6 Molecular energy-level alignments in devices based on the PBDB-T:acceptor 1:acceptor 2-based ternary solar cells. | |
In the PBDB-T:ITIC:ITBTD ternary system with 20% ITBTD content, the A2 acceptor molecule showed increased VOC (from 0.896 to 0.921) compared with the PBDB-T:ITIC binary system (Table 3 and Fig. 7), which is a key advantage of using the A2 acceptor with a higher LUMO level than ITIC. However, unlike VOC, Jsc and FF decreased, resulting in lower photoelectric efficiency PCE = 7.48% (approximately 17% decrease compared with the PBDB-T:ITIC binary system). This is because ITBTD molecules with lower acceptor performance than ITIC molecules adversely affected the device performance. In particular, a sharp decrease in FF (from 0.66 to 0.57 V) indicates that the formation of bulk-heterojunction layers of optimized PBDB-T:ITICs was disturbed by the participation of ITBTD molecules, and this often tended to appear when A2 acceptor molecules with lower performance than the host acceptor molecule were used in the ternary system.58–60 However, when the ITBTD content decreased to 10%, VOC remained the same, whereas JSC and FF improved compared with the 20% content, thereby increasing the photoelectric efficiency to 8.36% (approximately 6% lower than that of the PBDB-T:ITIC binary system). This is because the decrease in the ITBTD content naturally mitigated the adverse effect of the ITBTD A2 acceptor molecules. Remarkably, when the ITBTD content decreased by 5%, both JSC and FF, as well as the VOC, were higher than those of the PBDB-T:ITIC binary system, resulting in an improvement in the photoelectric efficiency by more than 9% (PCE = 9.71%). This tendency also appeared in a ternary system with both ITBTDF and ITBTD-IC used as A2 acceptor molecules (Table 3 and Fig. S11–S14†). Especially, it was confirmed that the TOSC system with 5% ITBTDF added has the highest value of 9.79%, which increased the photoelectric efficiency by approximately 10% compared with the PBDB-T:ITIC binary devices.
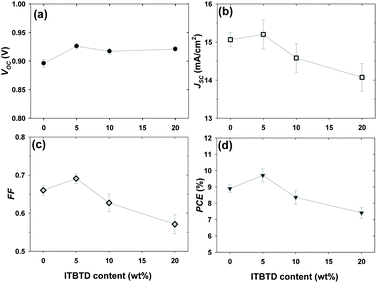 |
| Fig. 7 The changes of (a) VOC, (b) JSC, (c) FF and (d) PCE as a function of ITBTD (A2 acceptor) content in PBDB-T:ITIC:ITBTD-based ternary solar cells. | |
We can construe the fact that the photoelectric efficiency of the ternary devices was improved by up to 10% by only adding a very small amount, approximately 5%, of A2 acceptor molecules which have inferior performance to the host ITIC acceptor molecule as follows. In fact, adding a small amount of A2 molecules cannot significantly affect the bulk-heterojunction morphology in the PBDB-T:ITIC binary system, which is confirmed from the atomic force spectroscopy (AFM) measurements for PBDB-T:ITIC, PBDB-T:ITIC:ITBTD (1.00
:
0.95
:
0.05), and PBDB-T:ITBTD blend films (Fig. S15†). As shown in Fig. S15,† the root-mean-square (RMS) value of the PBDB-T:ITIC:ITBTD (1.00
:
0.95
:
0.05) blend film was found to be 3.0 nm, which is similar to that of the PBDB-T:ITIC blend film (3.3 nm) rather than that of the PBDB-T:ITBTD blend film (2.1 nm), indicating that the ternary blend largely reserves the original bulk-heterojunction framework of the PBDB-T:ITIC blend. Therefore, the holes and electrons produced after charge separation in the ternary system with a small amount of A2 acceptor molecules added were expected to migrate in the channels created mainly by the host donor polymer (PBDB-T) and acceptor molecule (ITIC). This would be beneficial for maintaining efficient charge transfer channels related to the device JSC and FF. As a result, it can be seen that the addition of a small amount of A2 molecules did not deteriorate the good compatibility between active materials in the blended films, which play a very important role in the performance of the TOSC device.61,62
However, the LUMO level of the A2 acceptor molecule being located between the LUMO levels of the host donor polymer and (A1) acceptor molecule, namely, the bridging effect, can create the cascade energy alignment,3 and then enable additional exciton dissociation at the D/A2, D/A1, and A1/A2 interfaces. In particular, we could confirm the efficient exciton dissociation at the A1/A2 interface by the photoluminescence (PL) quenching effect of ITIC (A1):ITBTD (A2) blended films as a function of ITBTD content (Fig. S16†).63,64 More importantly, the cascade energy alignment can play an effective role in enhancing the charge transfer by decreasing the trap density between D and A1.1–7 Such generation of additional exciton dissociation and enhancement of charge transfer consequently can lead to increased JSC and FF of the ternary system. Moreover, it was clearly verified that when we used an ITIC-ICS (LUMO = −4.02 eV) molecule, as an A2 acceptor molecule, which cannot create the cascade energy alignment between the host donor polymers (PBDB-T) and A1 acceptor molecules (ITIC) due to a deeper LUMO level than ITIC (LUMO = −3.85 eV), the photoelectric efficiency of the TOSC device, even after the added content decreased to 5%, was 8.13%, which was still lower than that of the PBDB-T:ITIC binary system (approximately 9% decrease) (Table 3 & Fig. S13†). This is because the ITIC-ICS A2 acceptor molecules, having a deeper LUMO level than the host acceptor ITIC, could not enhance the VOC of the ternary system; instead, they lowered the charge transfer properties by forming a deep charge trap between PBDB-T and ITIC. Therefore, despite the 5% addition of ITIC-ICS, the FF of the TOSC system only increased to 0.60, which is still lower than that of the PBDB-T:ITIC binary system (FF = 0.66).
From the energetic perspective, the energy transfer model can also work with the cascade charge transfer model in the TOSC system. In general, energy transfer can occur in various ways within the TOSC system, mainly through Förster resonance energy transfer (FRET).1–3 For example, energy transfer can occur between the host donor polymer and A2 acceptor molecule8 or between the host (A1) and A2 acceptor molecules.58,65,66 The energy transfer process that has occurred at each interface can help generate more excitons, contributing to enhanced current in devices. For efficient FRET, the emission spectrum of the energy donor molecule must significantly overlap with the absorption spectrum of the energy acceptor molecule.3,67 In this regard, it is unlikely that the energy transfer between PBDB-T donor polymer and A2 acceptor molecules such as ITBTD, ITBTDF, and ITBTD-IC occurs effectively since there is a small overlap in the absorption and emission spectral regions of these materials (Fig. S17(a) and (b)†). However, it was revealed that the emission spectra of these A2 acceptor molecules and the absorption spectrum of the host ITIC molecule overlap in large regions (Fig. S17(c)†), indicating that these molecules are more likely to transfer energy to the ITIC molecule than the host donor polymer (PBDB-T). Another promising positive role of the A2 acceptor molecule in the ternary system is improving photon harvesting through additional light absorption. However, we found out that it is difficult to expect an effective photon harvesting improvement by adding a tiny amount, approximately 5%, of A2 acceptor molecules into the ternary system from the insignificant difference between the absorption spectra of the PBDB-T:ITIC and PBDB-T:ITIC:A2 blending films (Fig. S18†).
Conclusions
In this study, we synthesized various ITIC derivatives that can be used as third components (A2 acceptors) in TOSC units by symmetrically or asymmetrically introducing functional termini of the IND group (BTD, BTDF, IC, and ICS) having different electron affinities to the IDTT central unit. The absorption bands and LUMO energy levels of the synthesized ITIC derivatives were precisely regulated according to the electron affinity strength of the terminal IND groups. In addition, the VOC of the BOSC system that used these ITIC derivatives as host acceptors was controlled accurately by the LUMO energy level of the relevant ITIC derivatives. In the PBDB-T:ITIC:A2-based ternary system, we ascertained that simply adding ITIC derivatives, which can form a cascade energy alignment between the LUMO levels of the host polymer (PBDB-T) and A1 acceptor molecule (ITIC), as a small amount (approximately 5%) of A2 acceptor molecules, regardless of the symmetric or asymmetric structure, can promote effective charge transfer while not disturbing the morphology of the PBDB-T:ITIC blend, and thus enhance VOC, JSC, and FF, synchronously, which resultantly improve the photoelectric efficiency by up to 10% more than that of a PBDB-T:ITIC binary system of the same structure. The results of this study are expected to help not only find the selection criteria for A2 acceptor molecules suitable for effective use in TOSC devices but also develop high-performance TOSC devices.
Conflicts of interest
There are no conflicts to declare.
Acknowledgements
This work was financially supported by the Global Frontier R&D Program on Center for Multiscale Energy System through the NRF funded by the MSIP (2012M3A6A7055540) and Basic Science Research Program through the NRF funded by the Ministry of Science, ICT and Future Planning (2017R1E1A1A01075372).
References
- N. Gasparini, A. Salleo, I. McCulloch and D. Baran, The role of the third component in ternary organic solar cells, Nat. Rev. Mater., 2019, 4, 229–242 CrossRef.
- M. Zhang, J. Wang, X. Ma, J. Gao, C. Xu, Z. Hu, L. Niu and F. Zhang, Review on smart strategies for achieving highly efficient ternary polymer solar cells, APL Mater., 2020, 8, 090703 CrossRef CAS.
- D. Zhou, W. You, H. Xu, Y. Tong, B. Hu, Y. Xie and L. Chen, Recent progress in ternary organic solar cells based on solution-processed non-fullerene acceptors, J. Mater. Chem. A, 2020, 8, 23096–23122 RSC.
- W.-L. Xu, B. Wu, F. Zheng, X.-Y. Yang, H.-D. Jin, F. Zhu and X.-T. Hao, Förster resonance energy transfer and energy cascade in broadband photodetectors with ternary polymer bulk heterojunction, J. Phys. Chem. C, 2015, 119, 21913–21920 CrossRef CAS.
- B. Fan, W. Zhong, X. Jiang, Q. Yin, L. Ying, F. Huang and Y. Cao, Improved performance of ternary polymer solar cells based on a nonfullerene electron cascade acceptor, Adv. Energy Mater., 2017, 7, 1602127 CrossRef.
- Q. An, F. Zhang, W. Gao, Q. Sun, M. Zhang, C. Yang and J. Zhang, High-efficiency and air stable fullerene-free ternary organic solar cells, Nano Energy, 2018, 45, 177–183 CrossRef CAS.
- C. Yang, Y. Sun, Q. Li, K. Liu, X. Xue, Y. Huang, K. Ren, L. Li, Y. Chen, Z. Wang, S. Qu and Z. Wang, Nonfullerene ternary organic solar cell with effective charge transfer between two acceptors, J. Phys. Chem. Lett., 2020, 11, 927–934 CrossRef CAS PubMed.
- M. Zhang, F. Zhang, Q. An, Q. Sun, W. Wang, J. Zhang and W. Tang, Highly efficient ternary polymer solar cells by optimizing photon harvesting and charge carrier transport, Nano Energy, 2016, 22, 241–254 CrossRef CAS.
- H. Lu, J. Zhang, J. Chen, Q. Liu, X. Gong, S. Feng, X. Xu, W. Ma and Z. Bo, Ternary-blend polymer solar cells combining fullerene and nonfullerene acceptors to synergistically boost the photovoltaic performance, Adv. Mater., 2016, 28, 9559–9566 CrossRef CAS.
- N. Gasparini, L. Lucera, M. Salvador, M. Prosa, G. D. Spyropoulos, P. Kubis, H. –J. Egelhaaf, C. J. Brabec and T. Ameri, High-performance ternary organic solar cells with thick active layer exceeding 11% efficiency, Energy Environ. Sci., 2017, 10, 885–892 RSC.
- R. Ma, Y. Chen, T. Liu, Y. Xiao, Z. Luo, M. Zhang, S. Luo, X. Lu, G. Zhang, Y. Li, H. Yan and K. Chen, Improving the performance of near infrared binary polymer solar cells by adding a second non-fullerene intermediate band-gap acceptor, J. Mater. Chem. C, 2020, 8, 909–915 RSC.
- L. Yang, H. Zhou, S. C. Price and W. You, Parallel-like bulk heterojunction polymer solar cells, J. Am. Chem. Soc., 2012, 134, 5432–5435 CrossRef CAS PubMed.
- B. M. Savoie, S. Dunaisky, T. J. Marks and M. A. Ratner, The scope and limitations of ternary blend organic photovoltaics, Adv. Energy Mater., 2015, 5, 1400891 CrossRef.
- W. Zhang, J. Huang, J. Xu, M. Han, D. Su, N. Wu, C. Zhang, A. Xu and C. Zhan, Phthalimide polymer donor guests enable over 17% efficient organic solar cells via parallel-like ternary and quaternary strategies, Adv. Energy Mater., 2020, 10, 2001436 CrossRef CAS.
- P. P. Khlyabich, B. Burkhart and B. C. Thompson, Efficient ternary blend bulk heterojunction solar cells with tunable open-circuit voltage, J. Am. Chem. Soc., 2011, 133, 14534–14537 CrossRef CAS.
- R. A. Street, D. Davies, P. P. Khlyabich, B. Burkhart and B. C. Thompson, Origin of the tunable open-circuit voltage in ternary blend bulk heterojunction organic solar cells, J. Am. Chem. Soc., 2013, 135, 986–989 CrossRef CAS.
- L. Zhan, S. Li, T. K. Lau, Y. Cui, X. Lu, M. Shi, C.-Z. Li, H. Li, J. Hou and H. Chen, Over 17% efficiency ternary organic solar cells enabled by two non-fullerene acceptors working in an alloy-like model, Energy Environ. Sci., 2020, 13, 635–645 RSC.
- X. Liu, Y. Yan and Y. Yao, Ternary blend strategy for achieving high-efficiency organic solar cells with nonfullerene acceptors involved, Adv. Funct. Mater., 2018, 28, 1802004 CrossRef.
- N. Gasparini, A. Salleo, I. McCulloch and D. Baran, The role of the third component in ternary organic solar cells, Nat. Rev. Mater., 2019, 4, 229–242 CrossRef.
- Y. Zhang and G. Li, Functional third components in nonfullerene acceptor-based ternary organic solar cells, Acc. Mater. Res., 2020, 1, 158–171 CrossRef CAS.
- L. Chang, M. Sheng, L. Duan and A. Uddin, Ternary organic solar cells based on non-fullerene acceptors: A review, Org. Electron., 2021, 90, 106063 CrossRef.
- G. Zhang, J. Zhao, P. C. Y. Chow, K. Jiang, J. Zhang, Z. Zhu, J. Zhang, F. Huang and H. Yan, Nonfullerene acceptor molecules for bulk heterojunction organic solar cells, Chem. Rev., 2018, 118, 3447–3507 CrossRef CAS.
- Y. Lin, J. Wang, Z. G. Zhang, H. Bai, Y. Li, D. Zhu and X. Zhan, An electron acceptor challenging fullerenes for efficient polymer solar cells, Adv. Mater., 2015, 27, 1170–1174 CrossRef CAS.
- S. Li, L. Ye, W. Zhao, S. Zhang, S. Mukherjee, H. Ade and J. Hou, Energy-level modulation of small-molecule electron acceptors to achieve over 12% efficiency in polymer solar cells, Adv. Mater., 2016, 28, 9423–9429 CrossRef CAS.
- W. Zhao, S. Li, H. Yao, S. Zhang, Y. Zhang, B. Yang and J. Hou, Molecular optimization enables over 13% efficiency in organic solar cells, J. Am. Chem. Soc., 2017, 139, 7148–7151 CrossRef CAS PubMed.
- F. Zhao, H. Zhang, R. Zhang, J. Yuan, D. He, Y. Zou and F. Gao, Emerging approaches in enhancing the efficiency and stability in non-fullerene organic solar cells, Adv. Energy Mater., 2020, 10, 2002746 CrossRef CAS.
- J. Yuan, Y. Zhang, L. Zhou, G. Zhang, H. –L. Yip, T. –K. Lau, X. Lu, C. Zhu, H. Peng, P. A. Johnson, M. Leclerc, Y. Cao, J. Ulanski, Y. Li and Y. Zou, Single-junction organic solar cell with over 15% efficiency using fused-ring acceptor with electron-deficient core, Joule, 2019, 3, 1140–1151 CrossRef CAS.
- B. Lu, J. Wang, Z. Zhang, J. Wang, X. Yuan, Y. Ding, Y. Wang and Y. Yao, Recent progress of Y-series electron acceptors for organic solar cells, Nano Sel., 2021, 1–11 Search PubMed.
- M. Zhang, F. Zhang, J. Wang, Q. An and Q. Sun, Efficient ternary polymer solar cells with a parallel-linkage structure, J. Mater. Chem. C, 2015, 3, 11930–11936 RSC.
- N. Gasparini, X. C. Jiao, T. Heumueller, D. Baran, G. J. Matt, S. Fladischer, E. Spiecker, H. Ade, C. J. Brabec and T. Ameri, Designing ternary blend bulk heterojunction solar cells with reduced carrier recombination and a fill factor of 77%, Nat. Energy, 2016, 1, 16118 CrossRef CAS.
- L. Hu, Y. Liu, L. Mao, S. Xiong, L. Sun, N. Zhao, F. Qin, Y. Jiang and Y. Zhou, Chemical reaction between an ITIC electron acceptor and an amine-containing interfacial layer in non-fullerene solar cells, J. Mater. Chem. A, 2018, 6, 2273–2278 RSC.
- H. Yao, L. Ye, J. Hou, B. Jang, G. Han, Y. Cui, G. M. Su, C. Wang, B. Gao, R. Yu, H. Zhang, Y. Yi, H. Y. Woo, H. Ade and J. Hou, Achieving highly efficient nonfullerene organic solar cells with improved intermolecular interaction and open-circuit voltage, Adv. Mater., 2017, 29, 1700254 CrossRef.
- G. Han, Y. Guo, L. Ning and Y. Yi, Improving the electron mobility of ITIC by end-group modulation: The role of fluorination and π-extension, Sol. RRL, 2019, 3, 1800251 CrossRef.
- S. S. Li, L. Ye, W. C. Zhao, S. Q. Zhang, S. Mukherjee, H. Ade and J. H. Hou, Energy-level modulation of small-molecule electron acceptors to achieve over 12% efficiency in polymer solar cells, Adv. Mater., 2016, 28, 9423–9429 CrossRef CAS.
- H. Cao, N. Bauer, C. Pang, J. Rech, W. You and P. A. Rupar, End-cap group engineering of a small molecule non-fullerene acceptor: the influence of benzothiophene dioxide, ACS Appl. Energy Mater., 2018, 1, 7146–7152 CrossRef CAS.
- Y. Zhao, Z. Luo, G. Li, J. Luo, Z. –G. Zhang, Y. Li and C. Yang, De novo design of small molecule acceptors via fullerene/non-fullerene hybrids for polymer solar cells, Chem. Commun., 2018, 54, 9801–9804 RSC.
- L. Ye, Y. Xie, Y. Xiao, J. Song, C. Li, H. Fu, K. Weng, X. Lu, S. Tan and Y. Sun, Asymmetric fused-ring electron acceptor with two distinct terminal groups for efficient organic solar cells, J. Mater. Chem. A, 2019, 7, 8055–8060 RSC.
- M. Lia, Y. Zhoua, J. Zhangc, J. Songa and Z. Bo, Tuning the dipole moments of nonfullerene acceptors with an asymmetric terminal strategy for highly efficient organic solar cells, J. Mater. Chem. A, 2019, 1, 8889–8896 RSC.
- W. Gao, M. Zhang, T. Liu, R. Ming, Q. An, K. Wu, D. Xie, Z. Luo, C. Zhong, F. Liu, F. Zhang, H. Yan and C. Yang, Asymmetrical ladder-type donor-induced polar small molecule acceptor to promote fill factors approaching 77% for high-performance nonfullerene polymer solar cells, Adv. Mater., 2018, 30, 1800052 CrossRef PubMed.
- C. Li, Y. Xie, B. Fan, G. Han, Y. Yi and Y. Sun, A nonfullerene acceptor utilizing a novel asymmetric multifused-ring core unit for highly efficient organic solar cells, J. Mater. Chem. C, 2018, 6, 4873–4877 RSC.
- J. Song, C. Li, L. Ye, C. Koh, Y. Cai, D. Wei, H. Y. Woo and Y. Sun, Extension of indacenodithiophene backbone conjugation enables efficient asymmetric A–D–A type non-fullerene acceptors, J. Mater. Chem. A, 2018, 6, 18847–18852 RSC.
- A. Wadsworth, M. Moser, A. Marks, M. S. Little, N. Gasparini, C. J. Brabec, D. Baran and I. McCulloch, Critical review of the molecular design progress in non-fullerene electron acceptors towards commercially viable organic solar cells, Chem. Soc. Rev., 2019, 48, 1596–1625 RSC.
- X. Li, C. Li, L. Ye, K. Weng, H. Fu, H. S. Ryu, D. Wei, X. Sun, H. Y. Woo and Y. Sun, Asymmetric A–D–p–A-type nonfullerene small molecule acceptors for efficient organic solar cells, J. Mater. Chem. A, 2019, 7, 19348–19354 RSC.
- L. Yang, X. Song, J. Yu, H. Wang, Z. Zhang, R. Geng, J. Cao, D. Baran and W. Tang, Tuning of the conformation of asymmetric nonfullerene acceptors for efficient organic solar cells, J. Mater. Chem. A, 2019, 7, 22279–22286 RSC.
- K. Zhua, Y. Zhong, X. Wang, F. Li, L. Yu, L. Chu and M. Sun, Asymmetric ITIC acceptor for asymmetric benzodithiophene polymer solar cells, Dyes Pigm., 2020, 183, 108727 CrossRef.
- G. A. Richardson and E. S. Blake, Synthesis, thermal stability, flammability, and viscosity of some partially fluorinated and perfluorinated aromatic and polyaromatic ethers, Ind. Eng. Chem. Prod. Res. Dev., 1968, 7, 22–25 Search PubMed.
- K. Pareek, A. Ghosh, S. K. Sen and S. Banerjee, Synthesis, characterization and properties of new fluorinated poly(imide siloxane) co-polymers from 4,4′-(hexafluoro-isopropylidene)diphthalic anhydride, Des. Monomers Polym., 2010, 13, 221–236 CrossRef CAS.
- J. Hou, O. Inganäs, R. H. Friend and F. Gao, Organic solar cells based on non-fullerene acceptors, Nat. Mater., 2018, 17, 119–128 CrossRef CAS.
- H. Yao, Y. Cui, R. Yu, B. Gao, H. Zhang and J. Hou, Synthesis, and photovoltaic characterization of a small molecular acceptor with an ultra-narrow band gap, Angew. Chem., Int. Ed., 2017, 56, 3045–3049 CrossRef CAS PubMed.
- Y. Yan, Y. Li, J. W. Wang and C. H. Zhao, Effect of the substitution pattern on the intramolecular charge-transfer emissions in organoboron-based biphenyls, diphenylacetylenes, and stilbenes, Chem.–Asian J., 2013, 8, 3164–3176 CrossRef CAS.
- S. K. Panja, N. Dwivedi and S. Saha, Tuning the intramolecular charge transfer (ICT) process in push–pull systems: effect of nitro groups, RSC Adv., 2016, 6, 105786–105794 RSC.
- B. Kim, X. Ma, C. Chen, Y. Ie, E. W. Coir, H. Hashemi, Y. Aso, P. F. Green, J. Kieffer and J. Kim, Energy level modulation of HOMO, LUMO, and band-gap in conjugated polymers for organic photovoltaic applications, Adv. Funct. Mater., 2013, 23, 439–445 CrossRef CAS.
- L. Benatto and M. Koehler, Effects of fluorination on exciton binding energy and charge transport of π-conjugated donor polymers and the ITIC molecular acceptor: a theoretical study, J. Phys. Chem. C, 2019, 123, 6395–6406 CrossRef CAS.
- Z. Zheng, H. Yao, L. Ye, Y. Xu, S. Zhang and J. Hou, PBDB-T and its derivatives: a family of polymer donors enables over 17% efficiency in organic photovoltaics, Mater. Today, 2020, 35, 115–130 CrossRef CAS.
- C. J. Brabec, A. Cravino, D. Meissner, N. S. Sariciftci, T. Fromherz, M. T. Rispens, L. Sanchez and J. C. Hummelen, Origin of the open circuit voltage of plastic solar cells, Adv. Funct. Mater., 2001, 11, 374–380 CrossRef CAS.
- M. C. Scharber, D. Mühlbacher, M. Koppe, P. Denk, C. Waldauf, A. J. Heeger and C. J. Brabec, Design rules
for donors in bulk-heterojunction solar cells—Towards 10% energy -conversion efficiency, Adv. Mater., 2006, 18, 789–794 CrossRef CAS.
- K. Vandewal, A. Gadisa, W. D. Oosterbaan, S. Bertho, F. Banishoeib, I. Van Severen, L. Lutsen, T. J. Cleij, D. Vanderzande and J. V. Manca, The relation between open-circuit voltage and the onset of photocurrent generation by charge-transfer absorption in polymer:Fullerene bulk heterojunction solar cells, Adv. Funct. Mater., 2008, 18, 2064–2070 CrossRef CAS.
- L. Yang, W. Gu, L. Hong, Y. Mi, F. Liu, M. Liu, Y. Yang, B. Sharma, X. Liu and H. Huang, High performing ternary solar cells through Förster resonance energy transfer between nonfullerene acceptors, ACS Appl. Mater. Interfaces, 2017, 9, 26928–26936 CrossRef CAS PubMed.
- Y. Chen, Y. Qin, Y. Wu, C. Li, H. Yao, N. Liang, X. Wang, W. Li, W. Ma and J. Hou, From binary to ternary: improving the external quantum efficiency of small-molecule acceptor-based polymer solar cells with a minute amount of fullerene sensitization, Adv. Energy Mater., 2017, 7, 1700328 CrossRef.
- M. –Y. Ni, S. –F. Leng, H. Liu, Y. –K. Yang, Q. –H. Li, C. –Q. Sheng, X. Lu, F. Liu and J. –H. Wan, Ternary organic solar cells with 16.88% efficiency enabled by a twisted perylene diimide derivative to enhance the open-circuit voltage, J. Mater. Chem. C, 2021, 9, 3826–3834 RSC.
- M. Zhang, Z. Xiao, W. Gao, Q. Liu, K. Jin, W. Wang, Y. Mi, Q. An, X. Ma, X. Liu and C. Yang, Over 13% efficiency ternary nonfullerene polymer solar cells with tilted up absorption edge by incorporating a medium bandgap acceptor, Adv. Energy Mater., 2018, 8, 1801968 CrossRef.
- C. Xu, H. Chen, Z. Zhao, J. Gao, X. Ma, S. Lu, X. Zhang, Z. Xiao and F. Zhang, 14.46% Efficiency small molecule organic photovoltaics enabled by the well trade-off between phase separation and photon harvesting, J. Energy Chem., 2021, 57, 610–617 CrossRef.
- X. Ma, A. Zeng, J. Gao, Z. Hu, C. Xu, J. H. Son, S. Y. Jeong, C. Zhang, M. Li, K. Wang, H. Yan, Z. Ma, Y. Wang, H. Y. Woo and F. Zhang, Approaching 18% efficiency of ternary organic photovoltaics with wide bandgap polymer donor and well compatible Y6:Y6-1Oas acceptor, Natl. Sci. Rev., 2021, 8, nwaa305 CrossRef PubMed.
- X. Wang, Q. Sun, J. Gao, X. Ma, J. H. Son, S. Y. Jeong, Z. Hu, L. Niu, H. Y. Woo, J. Zhang and F. Zhang, Ternary organic photovoltaic cells exhibiting 17.59% efficiency with two compatible Y6 derivations as acceptor, Sol. RRL, 2021, 5, 2100007 CrossRef CAS.
- G. Cai, Y. Li, J. Zhou, P. Xue, K. Liu, J. Wang, Z. Xie, G. Li, X. Zhan and X. Lu, Enhancing open-circuit voltage of high-efficiency nonfullerene ternary solar cells with a star-shaped acceptor, ACS Appl. Mater. Interfaces, 2020, 12, 50660–50667 CrossRef CAS PubMed.
- J. Gao, W. Gao, X. Ma, Z. Hu, C. Xu, X. Wang, Q. An, C. Yang, X. Zhang and F. Zhang, Over 14.5% efficiency and 71.6% fill factor of ternary organic solar cells with 300 nm thick active layers, Energy Environ. Sci., 2020, 13, 958–967 RSC.
- L. Yang, L. Yan and W. You, Organic solar cells beyond one pair of donor–acceptor: ternary blends and more, J. Phys. Chem. Lett., 2013, 4, 1802–1810 CrossRef CAS PubMed.
Footnotes |
† Electronic supplementary information (ESI) available. See DOI: 10.1039/d1se01429c |
‡ These authors contributed equally. |
|
This journal is © The Royal Society of Chemistry 2022 |