DOI:
10.1039/D2SD00037G
(Tutorial Review)
Sens. Diagn., 2022,
1, 686-708
Recent advances in skin-like wearable sensors: sensor design, health monitoring, and intelligent auxiliary
Received
5th March 2022
, Accepted 2nd June 2022
First published on 20th June 2022
Abstract
When entering old age, the tissue structure of the body begins to age, the functions of various organs appear disordered, resistance declines and is prone to various geriatric diseases. Geriatric diseases are characterized by a long course of the disease, slow recovery, and high medical costs. Therefore, a simple, rapid, economical, and effective method is urgently needed to detect the indicators of patients for long-term and continuous monitoring to reduce the medical burden. Wearable sensors, with their advantages of real-time, economical, simple operation, and non-invasion have attracted extensive attention and have good application potential in the health monitoring of elderly patients and the development of intelligent auxiliary devices. This paper reviews the research progress of skin-like wearable sensors in health monitoring and intelligent auxiliary devices in recent years. According to different sensing mechanisms, this paper introduces skin-like wearable sensors for health monitoring, including electrochemistry, bioimpedance, photoelectricity, and other wearable sensors, as well as related research for the development of intelligent auxiliary devices. Finally, this paper summarizes the applications and future challenges of developing the skin-like wearable sensor into a widely used and accepted home medical device for elderly patients.
| Ziyu Huang is a postgraduate student in the School of Public Health, Medical College, Wuhan University of Science and Technology, and her main research interest is aptasensors. |
| Nicole Jaffrezic-Renault received her engineering degree from the Ecole Nationale Supérieure de Chimie, Paris, in 1971 and the Doctoratd'Etatés Sciences Physiques from the University of Paris-Sud in 1976. As Director of Research at the Centre National de la Recherche Scientifique, past president of the French chemical microsensor club (CMC2), president of the Analytical Division of the French Chemical Society, her research activities in the Institute of Analytical Sciences, include conception and design of (bio)chemical sensors and their integration in microsystems. She coordinates several European and national projects for the development of microsystems for biomedical and environmental monitoring and food safety. She has published more than 500 papers with more than 10 000 citations. She is currently Editor-in-Chief of Chemosensors MDPI. |
| Zhenzhong Guo received his PhD from the University of Lyon 1 (France). He was a visiting scholar at Keele University (UK). He is currently the Deputy Director of Hubei Province Key Laboratory of Occupational Hazard Identification and Control at Wuhan University of Science and Technology (P.R. China). His research interest is focused on aptasensors. |
1. Introduction
Geriatric diseases refer to a class of aging-related diseases from which people in old age are prone to suffer with its own characteristics.1,2 As the human body enters the old age, tissues begin to age, and the function of various organs is impaired, while the resistance gradually weakens, the adaptability declines, and it is susceptible to various senile diseases, such as coronary heart disease, bronchitis, diabetes, and Parkinson's disease.3,4 The etiology of these diseases is often not so clear that there are no obvious symptoms and signs in the early stage, and the disease changes in various ways, with a lack of specific treatment. In addition, geriatric diseases have a long course of the disease and slow recovery. Patients in the late stage of the disease often suffer from several geriatric diseases at the same time. Long-term clinical treatment leads to excessive medical burden and poor quality of life for patients. The excessive medical burden may lead to negative treatment of patients, resulting in further deterioration of the elderly, increasing medical costs, and falling into a vicious circle.5 Therefore, there is an urgent need for a simple, rapid, economical, and effective method to monitor patient indicators for a long time and continuously, so as to reduce the medical burden of patients and improve their quality of life.
At present, the traditional health monitoring methods mainly rely on traditional technologies, such as intravenous catheterization and large-scale ECG monitors, which require the use of cumbersome instruments and complex operating procedures, and have drawbacks such as time consuming, high cost, complex operation, and easy pollution, so they are not suitable for long-term real-time monitoring of the body state.6,7 In addition, samples may be obtained in a way that is invasive and may cause discomfort and pain to patients.8,9 In order to achieve the efficiency and acceptable degree of health monitoring, wearable sensors have been widely studied due to their advantages of real-time and long-term monitoring, non-invasive reliability, simplicity, and rapidity.10 Most importantly, wearable sensors have been widely used in health monitoring and in the development of intelligent auxiliary devices.
Global attention to health problems such as obesity has gradually radiated from the pharmaceutical industry to all walks of life, such as software and hardware. At the same time, with the aging of the population and the prevalence of cardiovascular disease, there is an increasing need to develop individual health care systems, thereby enabling early disease detection and timely response.11 The use of wearable technologies, which are becoming more prevalent among the general public, combined with smartphones and health apps that can record and monitor vital signs such as calorie expenditure, fitness activities, pulse, body weight, heart rate, oxygen levels, and sleep patterns without the restrictions of time and place,12,13 will initiate a revolution in health care systems.14 The wearable devices are not affected by the age of the users. They are convenient, non-invasive, and suitable for the elderly. It has a great impact on the growing old population. The new wave of body sensor devices is likely to have a significant impact on health care systems and quality of life. Wearable fitness sensors are popular worldwide and continue to grow in annual sales.15,16
Skin-like wearable sensors with a single function or multifunction play an important role in medical care, the development of smart devices, and other fields.17–21 This kind of wearable sensor can be attached to human skin and clothing surfaces, which has strong scalability, flexibility, and adhesion-ability.22–24 It can be designed as health monitoring devices for real-time monitoring of the user's respiratory rate, exercise frequency, body temperature, blood pressure, pulse, etc., within a certain period of time, and for long-term health monitoring of the body state. In addition, due to their excellent performances, the skin-like wearable sensors can be designed as intelligent auxiliary devices to help users complete necessary daily activities.25 In addition, the skin-like wearable sensor is simple in shape, small in size, and light in weight, which will not cause other burdens to users. For elderly patients with a long disease course and heavy treatment burden, home remote monitoring is helpful to help patients monitor body signals at home and reduce medical costs. The skin-like wearable sensors can be highly attached to the human skin or the surface of clothing with high comfort and acceptability. It has good application potential in health monitoring of elderly patients and assisting elderly patients in their daily life. Recent advances in health monitoring devices and intelligent assistive devices based on skin sensors can be seen in Fig. 1.
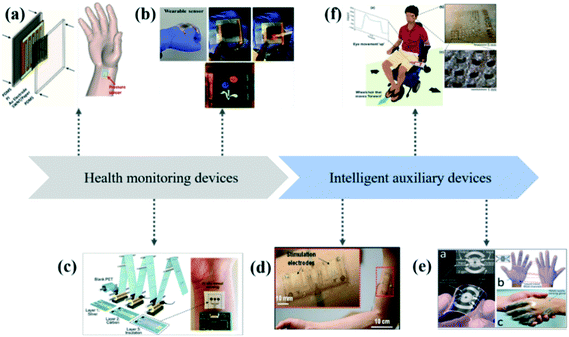 |
| Fig. 1 Recent advances in health monitoring devices and intelligent assistive devices based on skin sensors. (a) A flexible, wearable, and flexible skin-like pressure sensor, which could monitor important physiological signals in real time.97 (b) A wearable tactile sensor that responds instantly to external stimuli.159 (c) A wearable sensor based on the roll-to-roll (R2R) gravure printed electrodes for real-time, in situ perspiration monitoring during exercise.160 (d) An irritable, skin-like wearable sensor can induce muscle contractions by increasing electrical levels to aid in the recovery of paralyzed limbs.157 (e) A smart prosthesis based on skin-like sensors can be used by patients to receive tactile sensations as they grab, grab, squeeze, shake or touch.161 (f) A soft, conformal bioelectronics for a wireless human-wheelchair interface.162 | |
In this review, according to different sensing mechanisms, we reviewed a variety of skin-like wearable sensors, including electrochemical, bioimpedance, photoelectric and other wearable sensors and the research progress on the health monitoring and the development of smart assistive devices in recent years. Finally, we summarized the applications and future challenges of developing the skin-like wearable sensor into a widely used and accepted home medical device for elderly patients.
2. Design of skin-like wearable sensors
The basic components of wearable sensors are:26 1. The flexible substrates used to support other materials such as sensing components and conversion devices usually selected with stretchable and flexible poly-dimethylsiloxane (PDMS) and Ecoflex;27 2. The affinity of wearable sensors is usually improved by modification with biorecognition elements.28 Immobilized biological and sensitive materials used as recognition elements, including enzymes, antibodies, aptamers, and other bioactive substances;29 3. The sensitivity of wearable sensors can then be enhanced by the addition of nanomaterials to achieve signal amplification.28 The commonly used nanomaterials in wearable electrochemical biosensors and their characteristics are as follows:
1) Carbon based nanomaterials, such as graphene oxide (GO), reduced graphene oxide (rGO), and carbon nanotubes (CNTs), have the major advantage of increasing the electron transfer rate. In addition, the electrical conductivity and surface area can also be improved by chemically functionalizing the surface structure, leading to improved sensitivity of wearable sensors.30,31
2) Metallic nanomaterials such as gold nanoparticles (AuNPs), palladium nanoparticles (PdNPs), platinum NPs (PtNPs) and bimetallic composites: Au–PtNPs. Due to the strain and electronic effects of bimetals, bimetal nanomaterials show excellent performance and can be used as stabilizers for biometric components while promoting good electron transfer between target materials and sensing interfaces. The surface of Au–PtNPs with large pores can significantly improve the specific surface area and electrocatalytic performance of the electrodes.32 Amine or thiol linkers and various functional groups (–SH, –NH2, –CN) can assist gold nanoparticles in forming multilayer composite films at the sensor interface.33
3) Magnetic nanoparticles (MNPs), generally composed of a magnetic inner core composed of metal oxides such as iron, cobalt, and nickel and a polymer/silicon/hydroxyapatite shell wrapped outside the magnetic inner core, are a new class of materials that have been developed rapidly and are of great application potential in recent years.34,35 As both magnetic and polymeric particles, magnetic nanoparticles have the characteristics of magnetic guidance, biocompatibility, small size effect, surface effect, active groups, and certain biomedical functions. The surface modification of MNPs can not only enhance the stability of magnetic nanoparticles, but also improve their dispersion and biocompatibility in aqueous solution, improve targeting, prevent protein adsorption, increase their time in blood circulation, and further compound other nanoparticles, compounds, or biological ligands to realize the functionalization of magnetic nanoparticles.36,37 Moreover, MNPs can be controlled by an external magnet, which enables reproducible magnetic virus separation and further signal amplification in real clinical samples when simultaneously attaching target materials and biorecognition elements.38 The components of skin-like wearable sensors are displayed in Fig. 2.
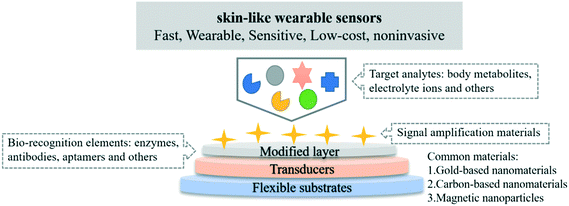 |
| Fig. 2 Schematic description of components for skin-like wearable sensors. | |
The wearable sensors can use different sensing mechanisms to detect target signals, and the selection of sensing mechanisms is determined by the design of the sensor, the materials used, and the target signals. The following will introduce the design of wearable sensors from different types of sensing mechanisms. The recently reported performance reviews of wearable sensors based on different sensing mechanisms are shown in Table 1.
Table 1 Summary of the performance of wearable sensors based on different sensing mechanisms reported recently
Sensing materials |
Mechanism |
Linear range |
Sensitivity |
Response time |
LOD |
Ref. |
Au/PtNP onto ACF |
Electrochemical |
Glucose 0–1000 μM |
22.8 ± 0.7 μA mM−1 cm−2 |
— |
1.7 ± 0.7 μM |
39
|
Choline 0–350 μM |
9.4 ± 3.9 μA mM−1 cm−2 |
10.5 ± 3.7 μM |
Lactate 0–20 mM |
4.1 ± 0.3 μA mM−1 cm−2 |
4.6 ± 3.0 μM |
Transdermal graphite/Teflon/AOx/HRP |
Electrochemical |
Ethanol 0.01–13.0 mM |
0.07 ± 0.003 μA mM−1 |
— |
— |
40
|
AOx/Pt on poly(o-phenylenediamine) film |
Electrochemical |
Alcohol 5.0 ± 80.0 mM |
0.045 nA mM−1 |
— |
— |
41
|
MKR (microfiber)/gold on PDMS |
Bioelectrical impedance |
— |
0.83 kPa−1 |
20 ms |
30 Pa |
42
|
Monolayer graphene on PDMS |
Piezoresistive |
Pressure 0–12 kPa |
8.5 kPa−1 |
40 ms |
1 Pa |
43
|
CuTCNQ nanowire arrays on PI film |
Piezoresistive |
Pressure 0–1500 pa |
6.25 kPa−1 |
10 ms |
0.73 Pa |
44
|
VHB/AgNPs on PDMS |
Resistive |
Pressure 1.5–6.5 kPa |
0.48 kPa−1 |
250 ms |
— |
45
|
2.1 Electrochemical wearable sensors
The electrochemical sensing mechanism is to measure the concentration of the target object according to the change of current, potential, impedance, or other electrical parameters when the target analysis is present.46,47 Electrochemical sensors have great potential in the development of wearable devices because of the advantages such as miniaturization, low budget, and simple operation. Electrochemical wearable sensors can noninvasively monitor the concentration of target biomarkers in different body fluids (such as sweat, urine, saliva, etc.) to analyze the health status of users.48
Anastasova et al.49 designed a fully integrated flexible skin-like microfluidic platform (Fig. 3) that simultaneously detected metabolite concentrations (lactic acid, etc.), electrolyte levels (pH, sodium, etc.) in human sweat, and autonomously calibrated internal temperatures. 1. By using polyvinyl chloride (PVC) functional film, a potential type sodium ion sensor was constructed on the inner layer of electrochemically deposited poly(3,4-ethylenedioxythiophene) (PEDOT) polymer. 2. A pH sensor was constructed based on iridium oxide film (IrOx) with high sensitivity. 3. Ampere-type lactic acid sensor was composed of doping enzymes deposited on the semi-permeable copolymer film and the outer polyurethane layer. The volunteers wore the prepared skin-like sensor patch for cyclic experiments, and the changes in pH measurements, sodium, and lactate concentration measured are shown in Fig. 3. This method had been successfully applied to monitor multiple targets simultaneously, demonstrating the potential of an integrated skin-like wearable platform for the long term, thereby using for monitoring target analytes in body fluids consistently, which could be used to monitor the health status.
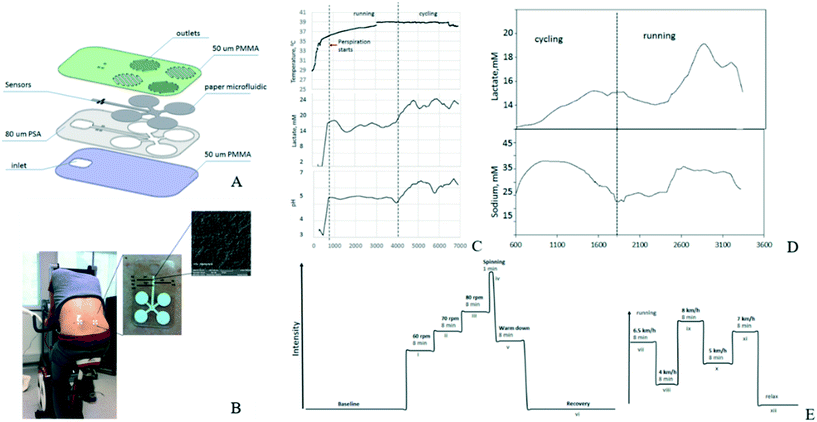 |
| Fig. 3 (A) Schematic representation of the fabrication steps of the micro-fluidic chip; (B) photo of the platform attached to the body and scanning electron microscopy (SEM) image photo of IrOx pH sensor membrane on top of a 50 μm Pt wire. (C–E) Changes in temperature, lactate, pH measurements, lactate and sodium levels during the experiments. Reproduced from ref. 49 with permission from [Elsevier], copyright [1969]. | |
2.2 Optical wearable sensors
Optical sensors have been successfully applied in various applications,50,51 for example, including in a wrist smart watch (for heart rate detection) and a pulsed blood oxygen wearable sensor. In the environment, light enters the body through the skin. Based on the changes in optical properties (e.g., absorption and scattering), the detector can capture changes in body data. The wavelength can extend from infrared to ultraviolet, depending on the penetration depth requirements of each detection application.52 Flexibility and stretchability of the wearable device affect the accuracy of the signal and adhesion to the skin.53
Among them, wearable sensors based on optical fiber have attracted more and more attention because of their advantages of good anti-interference, high sensitivity, small size, and ease in implementing multiplexed or distributed sensors.54,55 In recent years, people have vigorously promoted the development of optical fiber sensors, sensing technology for high sensitivity optical imaging of high resolution and other excellent performance is also gradually increasing in demand. As a combination of fiber optics and nanotechnology, optical micro/nano fibers (MNF) with smooth surface, good mechanical flexibility, micro/nano structure, and other properties have become a good choice for improving sensor sensitivity, response speed, optical resolution, and detection performance.56,57 The basic structure, functional materials, and signal conduction used by the MNF sensor are shown in Fig. 4. The sensing mechanism of the MNF sensor is complex. Next, the sensing mechanism of the pressure MNF sensor will be introduced through an example. For example, Tang et al.58 proposed a compact tactile sensor (CTS) with a diameter of 1.5 mm based on optical MNF materials, which could convert tactile and pressure stimuli into observable and comparable optical signals. As shown in Fig. 5a, the PDMS and Teflon tubing encapsulated a U-shaped MNF that had been attached to a silica capillary, and a micro-dome would be observed at the tip of the Teflon tubing. When the sensor contacts the object and is subjected to pressure stimulation, the crook degree of MNF will increase with the increase of pressure stimulation, resulting in the reduction of the output intensity transmitted by MNF (Fig. 5b). Thus, qualitative and quantitative analysis of the contact object and pressure stimulus is carried out through the output light intensity. The performance of the sensor is tunable in a wide range by changing the MNF diameter or the gap between the MNF and the apex of the micro-dome (Fig. 5c–e). This sensor could instantly sense the contact of objects and pressure stimuli, and the pressure detection sensitivity was up to 0.108 mN−1, and the resolution was 0.031 mN. This sensor provides some ideas for developing artificial intelligence products of object recognition.
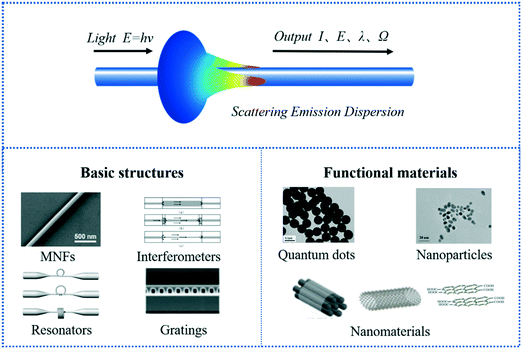 |
| Fig. 4 The basic structure, functional materials, and signal conduction used by the MNF sensor. | |
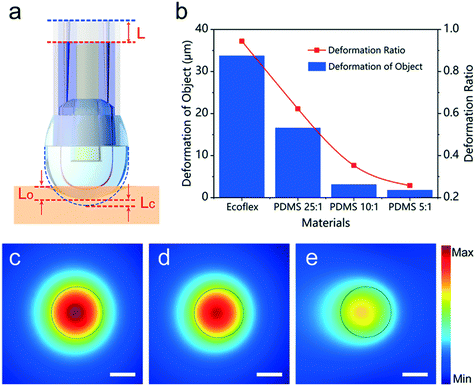 |
| Fig. 5 (a–e) Schematic and simulation of the deformation of the compact tactile sensor and calculated intensity distributions of electric field guided by different diameter MNF. Reproduced from ref. 58 with permission from [American Chemical Society], copyright [2021]. | |
Pan et al.22 developed a skin-shaped wearable optical (SLWO) sensor that could be fitted tightly to human skin via a sensing patch. The SLWO sensor could also monitor the angle of bending in a wide dynamic range that can regulate the sensitivity. Adjustable sensitivity (0.01 to 0.47%) was used for bending sensing, as well as high resolution for temperature detection so that it could monitor breathing, arm movement, and body temperature in real time. Zhu et al.59 proposed a stretchable wearable sensor of self-assembled wavy optical microfiber. The optical sensor had high sensitivity and good repeatability, which depended on the microfibril characteristics of the microfiber. Guo et al.60 reported a stretchable fiber Bragg grating-based optical (SFO) strain sensor interrogated by a free-running, dual-comb fiber laser. The manufacture and detection of the SFO sensor could be seen in Fig. 6. It had the ability of compliance, a highly scalable and flexible substrate, in line with curved and soft human skin, which could be used to monitor body activities. This sensor could be assembled on a suit or mounted directly on different parts of the human skin. Different human activities could be monitored in real time, including not only subtle movements, such as sound, breathing, and facial muscle movements, but also large-scale movements, such as marching, jumping, squatting, twisting joints, and bending.
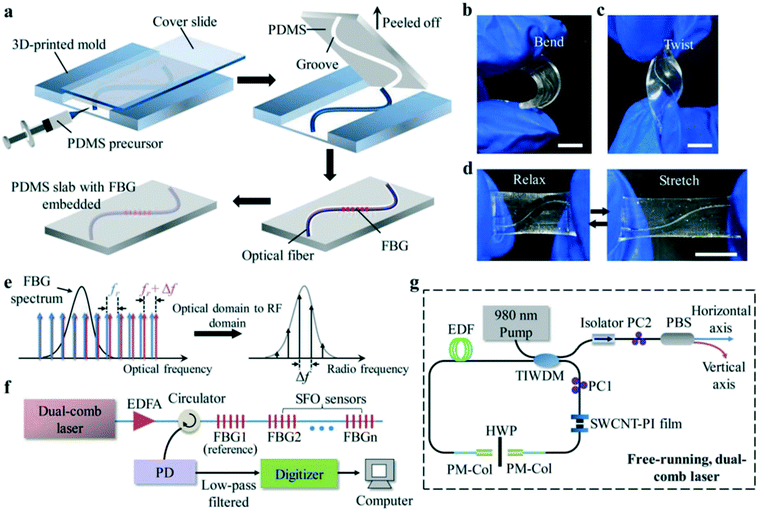 |
| Fig. 6 (a–g) Fabrication and interrogation of the SFO sensor. Reproduced from ref. 60 with permission from [John Wiley and Sons], copyright [2019]. | |
Currently, many researchers explore this technology because of its cost effectiveness, fast response, ultra-high sensitivity and low consumption, portability, accessibility, and simplicity or ease of use in clinical environments. Pan et al.61 designed a wearable flexible liquid filled fiber adapter (FLFFA) optical sensor that was sensitive to mechanical stimulation. The new FLFFA sensor was composed of two quartz fibers and a liquid-filled hose. In the presence of a liquid core, FLFFA had reconfigurability and adjustability in sensibility in properties. By bending or squeezing, the tube was deformed, which could cause a variation in light transmittance to being wavelength insensitive. Thanks to the signal recording system equipped with LED charge coupled device (CCD), 12 FLFFA output signals can be measured simultaneously.
Optical sensors have extraordinary potential in a wide range of clinical measurements and detection.62,63 However, the adjustable optical performance and reconfigurable sensor structure of wearable optical sensors still have huge challenges.61,64 Sometimes, through the optical interface of the sensor, the skin provides a path from the hidden blood vessel structure and organs. Hence, it is necessary to evaluate the optical properties of skin, especially in the design of optical sensors.65 Future research also could pave the improvement of measurement and detection.53
2.3 Bioelectrical impedance wearable sensors
In recent years, due to the popularity and development of wearable technology and mobile medical system, and considering the cost-effectiveness of remote health monitoring, these technologies have increasingly become the solutions of traditional medicine. In electricity, in a circuit with resistance, inductance and capacitance, and the blocking effect on alternating current (AC) is called impedance. Bioelectrical impedance analysis (BIA) is to use the conductive function of the human body to collect and measure the electrical impedance changes of tissues and cells through bioelectrical sensors, and then reconstruct the 3D mathematical model. Then, through the comparison of database samples, a comprehensive and scientific evaluation result of human current health status is obtained.66 BIA has many advantages, such as non-invasion, risk-free, affordable, and simple structure.67
Yao et al.68 designed a wearable electrical impedance tomography sensor with an elastic bandage and 8 electrodes were developed, and gesture recognition could be realized by a machine learning algorithm. Through the study of four kinds of electrodes, rectangular copper electrode was selected as the best electrode. Because the contact impedance engenders large noise, this would reduce the recognition rate in the measurement.69 The researchers reduced the contact impedance by applying a certain amount of medical conductive fluid to the skin in contact with the electrode and increasing the contact area and pressure between the electrode and the skin. To improve the rate of gestural identification, the investigators also designed electrical models of electrode skin contact impedance, which contributed to an improved rate of gestural identification.
In the clinic, BIA is often used to estimate body fat, in principle by measuring electrical impedance in humans. There is some contact resistance between the electrode and skin invariably, and hence there is an impedance error in measurement, which leads to some errors in estimating the percentage of body fat.70 To offset or reduce the effect of contact resistance, many commercial BIA human fat analyzers use electrodes large enough. Nevertheless, large size electrodes cannot be installed in wearable small devices, so it is becoming more and more important to use micro electrodes to accurately measure impedance.71
Jung et al.72 reported a wrist strap bioelectrical impedance sensor with a micro electrode, which could be used for daily obesity management. The composition of the human body was analyzed by exerting a small alternating current on the body and gauging the impedance. The sensor could make up for the contact resistance of the current exerting electrode and the contact resistance of the voltage sensing electrode. Therefore, even if the electrode size was quite small, the human impedance could be accurately estimated. The measurement time of the wrist wearable bioelectrical impedance sensor was only 7 seconds and could be shortened ulteriorly. This type of sensor technology provided novel possibilities for future intelligent wearable sensor devices.
2.4 Resistive wearable sensors
Skin and mucous membrane are the first barriers protecting the human body, and particularly the epidermis plays a pivotal part.73 The epidermis can protect the soft tissue from harmful radiation, and its base corner mold layer causes resistance due to its oily nature. Due to the rigidity of ordinary strain sensors, they cannot meet the requirements of wearable strain sensors.
Kim et al.74 proposed a simple wearable high-sensitive resistive pressure sensor, which was composed of solid carbon composite conductors with irregular surface morphology, which can be seen in Fig. 7. The biggest feature of the sensor was based on a remarkably pliant and strong carbon composite conductor, which was fabricated by perpendicularly arrayed carbon nanotube and inserted in a polydimethylsiloxane matrix with an inordinance surface. The sensor could be used to detect various human locomotion, including fine blood pulses and active joint movement and could be applied to draw the spatial pressure distribution map in the multi-pixel platform.
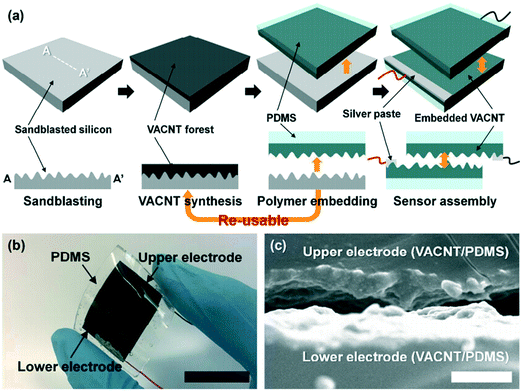 |
| Fig. 7 (a–c) Schematic illustration and material topography of a simple wearable high-sensitive resistive pressure sensor. Reproduced from ref. 68 with permission from [American Chemical Society], copyright [2017]. | |
Liao et al.75 developed an advanced wearable sensor for human motion detection. The micro crack assisted resistance strain sensor could achieve a relatively high sensing performance. The researchers integrated the patterned polydimethylsiloxane with silver nanowires to construct a strain sensor with super sensitivity and large tensile property. The excellent sensitivity depended on the patterned design of specific surface microstructure, which was 136 times higher than that of ordinary sensors. The sensing mechanism depended on the change of resistance. When the mechanical deformation strain was concentrated, the change of resistance was significantly affected by the surface microstructure of infiltration-microcrack. The sensor had excellent sensing, low creep, tensile and ultra-high stability.76 Because the signal of the resistance sensor is easy to collect, and the sensor structure is simple and easy to manufacture, it has also been widely used in pressure sensors in recent years.77,78 This simple, low-cost, and stable wearable resistance pressure sensor provides a certain direction and practicability for the advance of diverse wearable electronic products similar to skin.
2.5 The other wearable sensors
At present, several conductive materials with outstanding electrical and mechanical performances (such as graphene, carbon nanotubes, and metal particles)79,80 have been combined with elastomer composite substrates with success to manufacture a few typical flexible sensors. Nevertheless, these devices still have unfulfilling sensitiveness and high mechanical stiffness.81 Hydrogels are networks of hydrophilic polymer chains, sometimes called colloidal gels, where water is a dispersed medium. Due to inherent crosslinking, the structural integrity of the hydrogel network will not be dissolved by the high concentration of water. Hydrogels can be applied to wearable ionic devices by adjusting the toughness and fluidity of hydrogels.82 The anti-freeze and anti-conductive hydrogels have been widely developed as a flexible wearable sensor, which enables being connected to the skin to monitor all kinds of human motions and subtle physiological signals momentarily.83,84
Nagamine et al.85 reported a hydrogel based chemical touch sensor pad consisting of electrochemical L-lactic acid biosensors, which could extract and analyze sweat compositions noninvasively. The working electrode of the sensor was entirely covered with agar hydrogel holding sweat extract. Sweating could be detected without exercise by temperature or cholinergic drugs. When the sensor touched the skin, agarose hydrogel could incessantly detect L-lactic acid from the sweat of the body.
There are a variety of other new wearable sensors to meet diverse applications. For example, the high-performance wearable strain sensor for tracking human joint movement and muscle vibration is developed in ethylene glycol hydrogels by covalent crosslinking polymers (Paam).86
3. Health monitoring
The flexible wearable skin-like sensors have the ability to monitor and record physiological signals in real time for a long time and have been widely used in the field of medical and health monitoring.87–89 They can monitor the human body for a long time, continuously and in real time, with low burden, low discomfort, and high efficiency. Real-time collection and analysis of important physiological signals are the keys to realizing continuous and long-term monitoring of human health and personalized medicine. The skin-like sensor can be attached directly to clothing or skin to monitor the physical and chemical signals of the body's target in real time.90 In order to be successfully used for long-term health monitoring of the body, in addition to a flexible base and high efficiency, skin-like sensors also need to be highly flexible with strong durability, low power consumption, and high extensibility. These requirements make the construction of sensors more demanding.91,92 The flexible wearable skin-like sensors based on different sensing mechanisms can be used to develop long-term, real-time health monitoring equipment. According to the different sensing mechanisms, the health monitoring equipment prepared can be used to monitor different human health indicators.93,94 For example, wearable skin-like sensors based on optical sensing mechanisms are often used to develop sensitive and fast response blood oxygen monitoring instruments in the human body due to their advantages of good anti-interference, high sensitivity, and easy realization of multi-path or distributed sensors.95,96
To give an example of skin-like wearable sensors, Zhan et al.97 created a flexible, wearable, and flexible skin-like pressure sensor based on a novel SWNT/tissue paper, which could monitor important physiological signals in real time, such as muscle activity and pulse changes at various positions of the body (Fig. 8). It could be used to detect the change of minute stresses caused by small amplitude motion (such as light touch, heartbeat, and breathing) and prevent the occurrence of related diseases. In order to obtain better performance, such as higher sensitivity and lower detection limit, a flexible substrate with microstructure is used to prepare pressure skin-like wearable sensors. On the other hand, the selection of active materials is also crucial to the chemical stability of carbon nanotubes, and there have been a variety of mature technologies for efficient mass production of novel SWNT, which could improve sensor sensitivity as well as stability, and reduce construction costs.98–101 Flexible and wearable skin-like pressure sensors were made by impregnating single-walled carbon nanotubes in tissue paper and sandwiched between a bare PDMS sheet and a polyimide (PI) sheet with gold-forked electrodes. The construction method was simple while the cost was low, and almost no chemical reagents were needed. The typical sensitivity of 2.2 kPa−1 could be achieved in a wide range of 35–2500 Pa, and in the range of 2500–11
700 Pa, the sensitivity was 1.3 kPa−1. It was shown that the method had high sensitivity over a wide range of pressures.
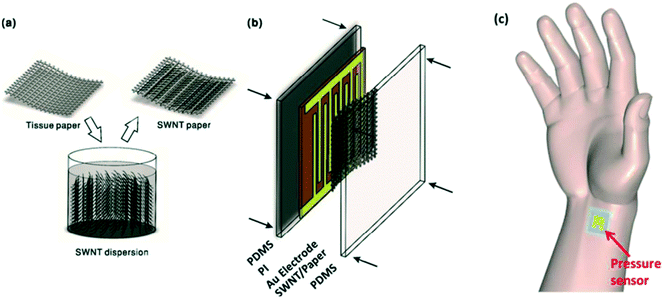 |
| Fig. 8 (a–c) Schematic illustration of the fabrication process of a pressure sensor. Reproduced from ref. 97 with permission from [American Chemical Society], copyright [2017]. | |
The skin-like pressure sensor could also be used as an artificial skin for the development of intelligent assisted robots that could measure the position and magnitude of pressure applied to the robot (Fig. 9). As can be seen from Fig. 9(b–f), the pressure sensor prepared in this experiment has excellent bending performance, and the flexible sensor array can detect the touched object sensitively and could quickly and sensitively identify the position and quantity of finger press. The principle was that when the finger touched the sensing area of the sensor, the matrix would make a quick response and reflect the signal on the monitor and showed the corresponding contact part on display. The response speed of the sensor array depends on the response speed of a single pressure sensor, so a large area of pressure sensing can be achieved without affecting the response speed, so that the shape of the touched object can be determined according to the contact area and quantity. This method has great application prospects in the development of low-cost artificial skin and wearable intelligent electronic products.
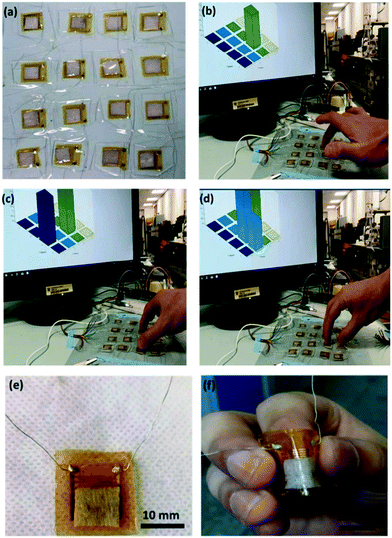 |
| Fig. 9 (a–f) A simple demonstration of flexible pressure sensor array as soft robotic skin. Reproduced from ref. 97 with permission from [American Chemical Society], copyright [2017]. | |
The skin-like wearable sensors are a cutting-edge method of wearable technology and are widely used in medical and health monitoring to help monitor the changes of various signals in the body and prevent the occurrence of potential diseases.88,102 Skin-like sensor, because of its small shape, good biocompatibility, flexible substrate, and other properties, can be a “tattoo”, closely attached to the human skin surface. Thus, on the basis of not affecting the user's daily life, it can continuously monitor a number of human health indicators so as to monitor the health status of the body.103,104 Electronic-skin (E-skin) can mimic human skin, with tactile perception, temperature detection, pressure testing, excellent tensile resistance, and other functions.105 Therefore, the E-skin sensor based on a flexible substrate has great application potential in many fields, including human health monitoring, surrounding environment perception, and the development of intelligent devices.106 At present, this technology has been widely used in human health monitoring, such as measuring heart rate, blood pressure, pulse, temperature, and other human health indicators, and can quickly respond to the changes in health indicators and even realize the early prevention and diagnosis of diseases.107 Chhetry et al.108 designed a dual-function sensor platform with high sensitivity for temperature and strain that can adjust the function of E-skin sensing based on materials with outstanding electrical properties, such as black phosphorus and laser-engraved graphene (BP@LEG). Fig. 10 shows the diagram of multifunctional BP@LEG based E-skin sensor, preparation schematic, and structure characterization results of the materials with outstanding electrical properties, and demonstrates that the E-skin sensor had been successfully prepared. This E-skin sensor showed a high temperature exponent of 8106 K (25–50 °C) with high strain sensitivity (i.e., gauge factor, GF) of up to 2765 (>19.2%), ultralow strain resolution, and longer durability. The good performance of the sensor satisfies the needs of E-skin, which provides a unique technical method and wide application prospects for human health monitoring and the development of multi-functional intelligent devices attached to E-skin.
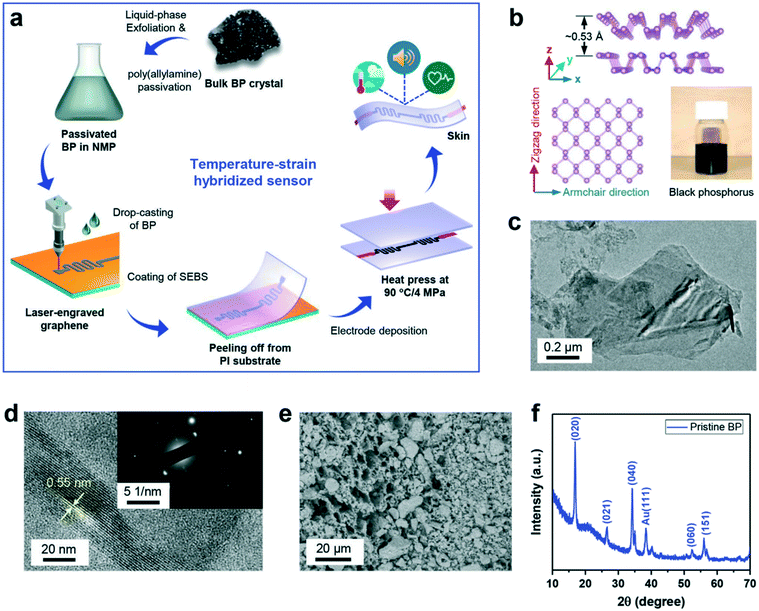 |
| Fig. 10 (a–f) Schematic diagram of multifunctional BP@LEG based E-skin sensor and the preparation schematic and structure characterization results of the materials with outstanding electrical properties. Reproduced from ref. 108 with permission from [John Wiley and Sons], copyright [2020]. | |
The skin-like wearable sensors have been widely used in personal health monitoring of geriatric disease patients, improving the elderly life quality of patients, and reducing the family medical burden.
3.1 Common ion and molecular concentration monitoring
The skin-like wearable sensors can be used for long-term, real-time monitoring of common ion and molecular concentrations in elderly patients with diseases, such as pH,109,110 glucose,111 drug molecules,112,113etc. Under the regulation of the nervous system and body fluids, the normal body maintains a relatively stable internal environment through the coordinated activities of various organs and systems, so the pH in the body should be in a stable state. The base of traditional ion-selective electrodes lacks flexibility and mechanical flexibility, which makes it impossible to make close contact with the human body during the measurement process and thus cannot be made into a wearable device.114 Many elastic materials are used to make flexible substrates for sensors, such as rubber, fibers, hydrogels, etc. Guinovart et al.115 showed a wearable pH sensor using CNT-coated cotton yarn, which was used as a conductive substrate. Nyein et al.116 reported a wearable skin-like electrochemical sensor that continuously monitored Ca2+ and pH in body fluids using a multifunctional flexible sensor array that interfaced with a flexible printed circuit board, and its structure schematic diagram is shown in Fig. 11. The platform has been successfully used for real-time quantitative analysis of specific ion concentrations in body fluids such as sweat, urine, and tears. Results displayed in Table 2 show the Ca2+ concentrations and pH in sweat and urine measured by the wearable flexible sensor array, ICP-MS, and Ca2+ selective sensors. Ca2+ acquired by sensors vary by a maximum 7.0% and 10%, respectively, from ICP-MS results. On the other hand, pH sensors show less than 2.2% and 3.6% variations from a commercial pH meter. Therefore, the sensors can be successfully applied to analyze Ca2+ and pH in sweat and urine. The sensors have the potential to be used to diagnose diseases such as primary hyperparathyroidism.
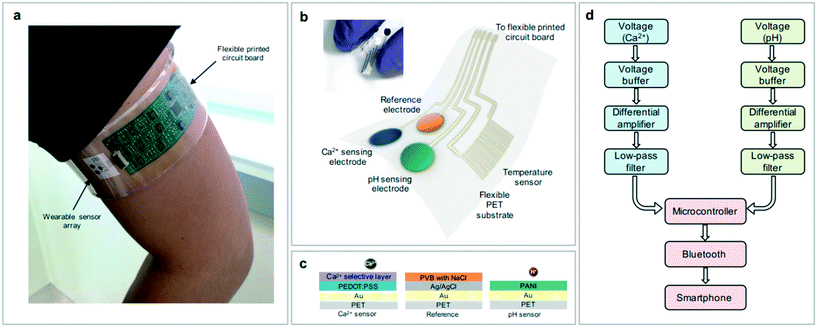 |
| Fig. 11 (a–d) Schematic diagram of the structure and circuit board system of the wearable flexible sensor array. Reproduced from ref. 116 with permission from [American Chemical Society], copyright [2016]. | |
Table 2 Ca2+ concentration and pH in sweat and urine were measured by the wearable flexible sensor array and compared with ICP-MS and commercial pH meter measurements. Reproduced from ref. 116 with permission from [American Chemical Society], copyright [2016]
|
[Ca2+] (mM) |
Ca2+ sensor |
pH |
pH |
Ca2+ sensor |
ICP-MS |
pH sensor |
pH meter |
Sweat 1 |
0.96 |
0.92 |
4.4 |
4.5 |
Sweat 2 |
0.48 |
0.48 |
7.2 |
7.3 |
Sweat 3 |
0.76 |
0.71 |
8.1 |
8.1 |
Urine 1 |
1.8 |
1.8 |
7.6 |
7.8 |
Urine 2 |
7.2 |
7.1 |
6.3 |
6.5 |
Urine 3 |
1.2 |
1.1 |
6.4 |
6.6 |
3.2 Blood glucose monitoring
Blood glucose levels are one of the key indicators of a person's health. The traditional method of measuring glucose is to collect blood samples by piercing the skin. This method is invasive and could increase discomfort. Studies have proved the correlation between glucose concentration in sweat and blood glucose concentration.117 Sweat could be used as the best experimental sample for noninvasive detection of blood glucose concentration. Glucose wearable sensors could realize rapid and noninvasive detection of glucose in sweat. Kim et al.111 reviewed the research progress and challenges of non-invasive skin-like electrochemical glucose sensing systems and demonstrated that human glucose had been successfully monitored using skin-like electrochemical sensors on the skin surface. This skin-like electrochemical biosensor successfully overcomes the shortcomings of traditional skin puncture methods and minimally invasive subcutaneous glucose biosensors, providing hope for blood glucose control in elderly patients with diseases.
3.3 Drug concentration monitoring
In addition to pH and glucose monitoring, drug monitoring also plays an important role in health monitoring. Drug monitoring relies on traditional blood analysis or urine analysis techniques, but these methods cannot provide real-time, on-site monitoring results and consume a long time and high cost.118 In this case, the development of skin-like wearable sensors for drug monitoring has great application prospects. The drug levodopa (L-Dopa), which treats Parkinson's disease, could be monitored using a skin-like wearable sensor. Goud et al.113 reported a continuous, minimally invasive microneedle sensing platform for cross-electrochemical monitoring of L-Dopa. This novel microneedle sensing platform was based on the construction of microneedles with different functions on the same sensor array patch to detect L-Dopa simultaneously and relatively independently using enzyme and non-enzyme voltammetry. L-Dopa was detected by square wave voltammetry and timing amperometry on unmodified and tyrosinase-modified carbon paste microneedle electrodes, respectively. The design and excellent analytical performances of the new wearable microneedle sensor array provided considerable application prospects for reliable, continuous, and minimally invasive monitoring of L-Dopa for the effective management of drug use in patients with Parkinson's disease. More importantly, the method had great potential for drug monitoring for other diseases of the elderly.
3.4 Body temperature monitoring
In addition to monitoring specific ions and molecules in the body, skin-like wearable sensors can also monitor body temperature.119 In addition, temperature sensors can be integrated into other sensor arrays to address the impact of temperature changes during monitoring.120 Trung et al.121 prepared a skin-like wearable temperature sensor based on freestanding single reduction graphene oxide (rGO) fiber, and its structure and basic characteristics are shown in Fig. 12. In this experiment, a kind of independent, wearable, temperature-responsive rGO fiber with adjustable heat index was obtained by a simple wet-spinning method and by controlling the reduction time of GO. This independent temperature sensor based on optical fiber has good performance, such as high responsivity, fast response time (7 s), and fast recovery time (20 s) to temperature. On the basis of high responsiveness, the sensor can also maintain good mechanical deformation ability. This method had great application potential in the field of medical care and real-time medical monitoring. It could be used to continuously monitor the postoperative temperature changes of elderly patients with diseases and assist in clinical drug use.
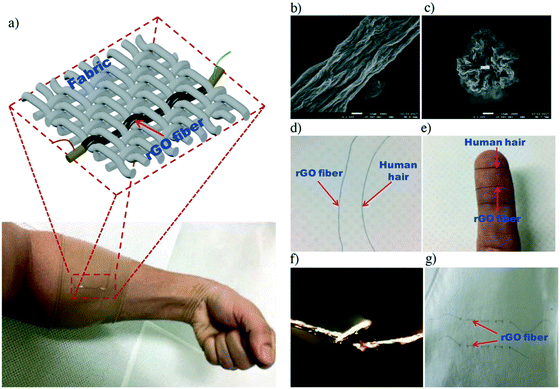 |
| Fig. 12 (a–g) The architecture and fundamental characteristics of the freestanding fiber-based wearable temperature sensor. Reproduced from ref. 121 with permission from [John Wiley and Sons], copyright [2018]. | |
3.5 Cardiovascular health indicators monitoring
The use of skin-like wearable sensors to monitor a user's heart rate, blood pressure, oxygen saturation, pulse, etc., has been widely reported and can be used for health monitoring of the occurrence and development of cardiovascular diseases.122,123 The technology is ripe for use in the field of aftercare for elderly patients with geriatric diseases. For example, Chen et al.124 developed a skin-like self-supplied voltage sensor based on hierarchical elastomer microstructure based on the strategy of adjusting the performance of self-supplied voltage sensors with different spatial densities. The sensor allowed continuous, long-term monitoring of the health of the heart and blood vessels and has been successfully used in continuous measurements of arterial stiffness, blood pressure, and the heart to assess cardiovascular health. The sensitivity could reach 7.989 V kPa−1; the working pressure range was across 0.1–60 kPa; the response was fast (40 ms); the signal-noise ratio was high (38 db); the stability was high. These good performances ensured its successful use in health monitoring of the cardiovascular status of the body. The sensor had the advantages of low cost, miniaturization, light weight, and low burden, and had great potential in the fields of medical care and intelligent auxiliary equipment development.
In addition to the above applications, the skin-like wearable sensor can also be used for the monitoring of bioelectrical signals, basic metal ions, body fluid specific molecules, etc. At present, more and more wearable sensors with high sensitivity, high specificity, and diverse functions have been reported, while wearable sensor technology in the field of medical monitoring has been more and more mature, but it is widely used in geriatric diseases in the elderly, and there are still some challenges yet breakthroughs, such as the elderly acceptance, degree of difficulty operating procedures, fully functioning, etc.
4. Intelligent auxiliary
Due to its characteristics of non-invasive, long-term use, portability, high biocompatibility and high detection accuracy, skin-like sensors with better performance are developing rapidly.125,126 In the implementation of the human body physiological signal monitoring, comprehensive building real-time man–machine control systems, movement state monitoring system, development pressure contact auxiliary equipment such as intelligence have excellent application prospects.127–130 Similarly, skin-like sensors based on different sensing mechanisms can be used to develop intelligent assistive devices with different functions.
Wearable skin-like sensors because of its shape characteristics, on the one hand, can fit closely on the human skin surface, according to the joint parts to determine the need to detect indicators, such as in the inner wrist using skin-like sensors can be used to monitor the body heart rate, pulse, blood oxygen index, etc.,131,132 and then through a simple data cable or Bluetooth connection with the mobile phone or computer terminal link.133,134 The detection signal received by the terminal can be processed twice by specific software or programs; for example, the change of the detection index is drawn into a broken line graph, so that the users can intuitively observe the changes in their own indicators, so as to achieve intelligent monitoring of human health, so as to prevent the occurrence of disease early.135–137 At present, smart watches have been widely put into the market, with a variety of functions such as monitoring sleep, heart rate, and running status, but there are still some aspects that need to be further improved, such as battery life, detection accuracy, and cannot be used alone.138 In addition, it can also be installed on the lumbar spine and cervical spine. The wearable sensor can accurately and in real-time record the signal of the user's sitting position through the bending deformation state, which is used to prevent cervical spondylosis and lumbar disc herniation, and guide a healthy lifestyle.139
On the other hand, due to its excellent performance, the skin-like wearable sensor can be used to develop a non-invasive intelligent man–machine detection interface, which uses the sensors to detect physiological signals of the body and connects with external receiving devices through Bluetooth, so as to realize the system interaction between people and devices.140–142 For example, many of the current elderly patients may end up bedridden and unable to move or express their thoughts. Mishra et al.143 designed a human–computer interaction system combining skin-like sensors and Bluetooth components and connected the sensor components to the smart wheelchair chain. Tightly on the skin around the eyes by the skin-like sensors, monitoring the movement of the eye, and will be collected by wireless transmission signals to the data collecting unit for signal processing, and then according to the preset program realization of a wheelchair intelligence operation, as shown in Fig. 13, such as eye upward movement, the wheelchair to go forward, down to stop movement, left for counterclockwise, for clockwise rotation to the right, etc. This human–computer interaction system, which can detect human data and control wheelchairs wirelessly in real time, shows great potential in the field of preparing long-term wearable and clinically applicable human–computer interaction intelligent assistive devices.
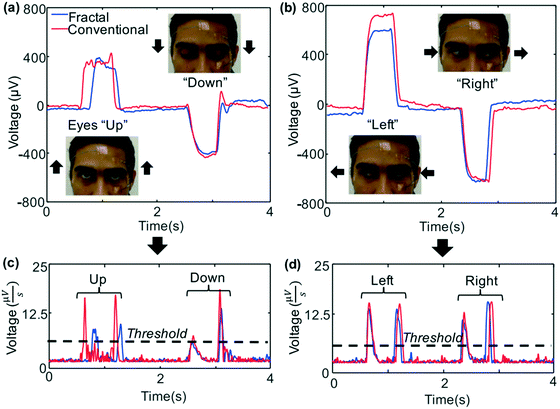 |
| Fig. 13 (a–d) A representative set of EOG signals involving four kinds of eye movements. Reproduced from ref. 143 with permission from [Elsevier], copyright [2017]. | |
Recently, devices that use photoplethysmography (PPG) to detect heart rate in real time have become popular.144–147 It is inexpensive and non-invasive to obtain various parameters that facilitate monitoring and control of exercise intensity. The PPG sensor wearing on the wrist showed satisfactory readings than wearing on the rest of the body.50 The most popular form of wearable electronic device is a wristband smartwatch equipped with a PPG sensor that senses changes in blood volume associated with the heart cycle, acquiring a wide range of information, including heart rate (HR), peripheral oxygen saturation, and respiratory rate.148–150 However, one of the key drawbacks of PPG sensors is the noise error caused by physical motion artifacts.16,151–154 However, there are still significant differences in the accuracy of optical heart rate sensors in different physical activities. Specifically, the device is more accurate in sedentary behavior than active exercise.12 Therefore, care should be taken when using wrist band optical sensors to monitor real-time heart rate during aerobic exercise.50
Lee et al.155 developed a skin-attachable PPG sensor with an orthogonal polarizer-analyzer (OPA) pair to reduce the amount of scattered light from the epidermis, which is the main cause of motion artifacts. The schematic diagram of the OPA sensor is shown in Fig. 14. The sensor, with two orthogonal polarizers, could successfully monitor PPG signals during wrist angular movements corresponding to physical activity, thus achieving continuous monitoring of individual daily health care activities. Getting high-quality PPG signals during physical activity can diagnose a variety of cardiovascular-related diseases.156 There are still significant differences in the accuracy of optical heart rate sensors in different physical activities. Specifically, the device is more accurate in sedentary behavior than active exercise.16 Thus, caution should be exercised when monitoring real-time heart rate during aerobic exercise using wristband fitness equipment.
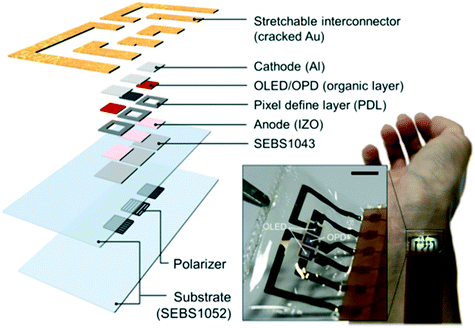 |
| Fig. 14 Schematic diagram of the layered layout of OPA sensor and retractable patch sensor in contact with the inner skin of wrist (scale bar, 3 mm). Reproduced from ref. 155 with permission from [Science], copyright [2022]. | |
Xu et al.157 reported on an ultra-thin and comfortable electronic device that integrated tactile stimuli, electromyography, and temperature and strain sensors on a simple platform. The metabolic response to the corresponding increase in skin temperature is related to the increase in blood flow. At this time, the temperature sensor could provide a reliable index of muscle strength and an important index in monitoring muscle fatigue. Fig. 15 shows the denatured status and EMG and strain comparison of skin-like strain sensors worn by volunteers in three different body postures during weightlifting. It was observed that the EMG signal remained constant, but the temperature increased significantly with time.
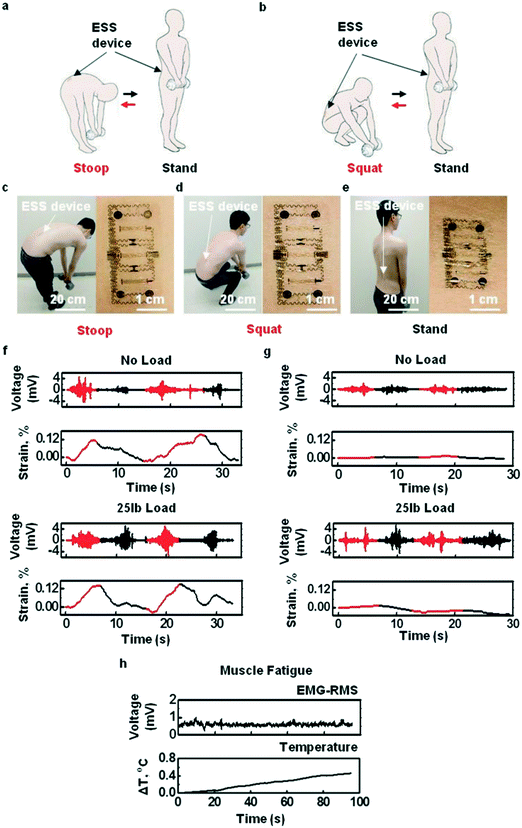 |
| Fig. 15 (a and b) Assessment of exertion and posture of the lower back during lifting. (c–e) Observed deformation during bending, squatting, and standing when the skin-like strain sensor is worn on the muscles of the lower back. (f–h) The EMG and strain comparison at different body postures and simultaneously recorded lower back EMG-RMS and temperature signals during muscle fatigue. Reproduced from ref. 157 with permission from [John Wiley and Sons], copyright [2015]. | |
The improved stimulation electrode of the device adopts a serpentine structure, which allows large strain deformation and covers a larger area, so as to stimulate multiple motion units. Therefore, as shown in the device with large-area electrodes for electrical muscle stimulation and corresponding experimental results (Fig. 16), the device could not only provide sensory input to the body, but it could also induce muscle contractions by increasing current levels. This has potential applications in the recovery of paralyzed limbs in patients, the assessment of neuromuscular function in athletes, or strength training.158
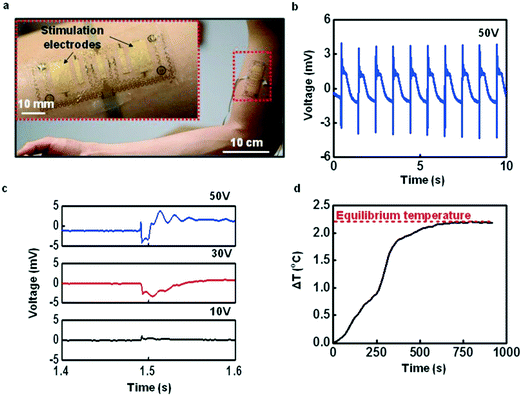 |
| Fig. 16 Device with large-area electrodes for electrical muscle stimulation. (a) Image of a device mounted on the biceps (magnified view, inset). (b and c) Example of evoked M-waves from induced contraction of the biceps at different stimuli. (d) Example of evoked M-waves from induced contraction of the biceps at different stimuli. Reproduced from ref. 157 with permission from [John Wiley and Sons], copyright [2015]. | |
A device installed on the patient's lower back allows simultaneous monitoring of temperature, strain, and electromyography as an indicator of force and provides stimulus feedback to prevent overextension. In this study, a 22-year-old male was fitted with a device (right lumbar paraspinal area) on his lower back that simultaneously monitored multiple physical and chemical signals and recorded motor skills. The bending action will cause the characteristic response of the strain gauge, which is different from the squatting position. The electromyography and strain measurement results of different movements were summarized. Compared with a no-load condition, the EMG activity under load condition is significantly increased, which indicates that the equipment has the ability to detect load lifting. Through a series of experiments, researchers have shown that multiple operation modes of this type of equipment can be used simultaneously in sensory motor control, lower back motion monitoring, and muscle stimulation of the robot system. It provides a valuable research direction and a basis for simultaneously recording physiological data and presenting the ability of nerve stimulation input.
According to the current smart device market demand, the skin-like sensor has a good application prospect in the construction of intelligent motion monitoring systems. Because of its rapid response, flexibility, and quantitative analysis of physiological parameters, skin sensor can realize intelligent monitoring of athletes' training process and sports recovery effect by performing real-time analysis of saliva and sweat in a non-invasive manner.163 Although wearable sensor research and commercialization process has had the very big advance, but truly non-invasive intelligent device commercialization inevitably has a lot of challenges to be overcome in retaining high performance at the same time, need to study how to make it more diversified joint human body function, high efficiency range, equipment of retrieval, etc.164,165
5. Conclusion and future prospects
Due to their excellent performance, skin-like wearable sensors have become a very promising research field in recent years. In this paper, we summarized the recent progress of wearable sensors for skin samples in health monitoring and intelligent assistive devices, according to the different sensing mechanisms of the sensor, introduced a variety of wearable sensors used in health monitoring of the skin samples, including electrochemistry, biological impedance, and photoelectric sensors. In addition, because wearable sensors have flexible substrates and can be in close contact with the body, situ detection can be achieved, and extremely high sensitivity can be obtained. So, the application of wearable sensors in the development of intelligent auxiliary devices has a good development prospect, and relevant research results have been reported.
Because flexible skin-like wearable sensors have the advantages of transparency, good flexibility, strong stretching ability, free folding and bending, close fitting, etc., they have great application potential in the fields of medical care and post-care for geriatric patients and have attracted more and more attention. Although significant progress has been made in the field of geriatric health care, there are still some areas for improvement and challenges in the future. First of all, in order to achieve long-term and continuous monitoring of patients' body signals, the sensitivity of wearable sensors is highly required. Sensors convert light, heat, chemicals, or other signals into electrical signals, so wearable sensors need good electrical conductivity. In addition, the samples are usually sweat, saliva, or the subtle changes in the skin surface, and pulse. The complex composition, the number of monitoring signals, and some subtle movements of the body may make it difficult for wearable sensors to make efficient and accurate measurements. Introducing nanomaterials with good conductive properties can effectively improve the stability, sensitivity, and reliability of wearable sensors. More and more research results on nanomaterials have been published, but there are still problems to be solved in the selection of nanomaterials. Nanomaterials should not only have good stability, electrical conductivity, and sensing performance, but also biodegradable materials can be selected to prevent environmental pollution after a large number of preparations. Further research on the properties of nanomaterials can also be carried out to obtain better performance.
More importantly, the world is now experiencing lower fertility rates, longer life expectancy, more elderly people, and more diseases of old age. There are many challenges to overcome before skin-like wearables can be widely used in healthcare for elderly patients. For example, the cost, comfort, portability, and popularization of wearable devices need to be solved. While retaining high sensitivity and specificity, multiple sensing components should be integrated as much as possible to achieve multi-functional wearable devices, while reducing costs, improving comfort, and product aesthetics.
Author contributions
Ziyu Huang: writing – original draft, conceptualization. Yaqi Xu: writing – original draft, conceptualization. Ya Cheng: investigation, conceptualization. Min Xue: investigation. Mengtian Deng: investigation. Nicole Jaffrezic-Renault: funding acquisition, writing – review & editing. Zhenzhong Guo: funding acquisition, conceptualization, supervision.
Conflicts of interest
The authors declare that they have no known competing financial interests or personal relationships that could have appeared to influence the work reported in this paper.
Acknowledgements
This work was supported by the National Natural Science Foundation of China No. 81973097, Key Research and Development Project of Hubei Province No. 2020BCB022, funded by Open Research Fund Program of the State Key Laboratory of Virology of China No. 2022KF002, Outstanding Young and Middle-Aged Technology Innovation Team Project of Hubei Provincial Department of Education T2020003, and the Opening Project of Key Laboratory of Optoelectronic Chemical Materials and Devices of Ministry of Education of Jianghan University JDGD-202018. CNRS France is acknowledged for support through IRN MAREES # 0876.
References
- A. Krishnaswami, C. Beavers and M. P. Dorsch,
et al., Gerotechnology for Older Adults With Cardiovascular Diseases: JACC State-of-the-Art Review, J. Am. Coll. Cardiol., 2020, 76(22), 2650–2670, DOI:10.1016/j.jacc.2020.09.606.
- Y. T. Choo, Y. Jiang and J. F. Hong,
et al., Effectiveness of Tai Chi on quality of life, depressive symptoms and physical function among community-dwelling older adults with chronic disease: A systematic review and meta-analysis, Int. J. Nurs. Stud., 2020, 111, 103737, DOI:10.1016/j.ijnurstu.2020.103737.
- P. Lange, J. Parner and E. Prescott,
et al., Chronic bronchitis in an elderly population, Age Ageing, 2003, 32(6), 636–642, DOI:10.1093/ageing/afg108.
- P. Vemuri, T. G. Lesnick and S. A. Przybelski,
et al., Age, vascular health, and Alzheimer disease biomarkers in an elderly sample, Ann. Neurol., 2017, 82(5), 706–718, DOI:10.1002/ana.25071.
- L. Huang, B. Zheng and C. Chen,
et al., To explore clinical value of single-port video-assisted thoracoscopic surgery in elderly patients with non-small cell lung cancer: Lobectomy, segmentectomy and lobectomy vs segmentectomy, Zhongguo Feiai Zazhi, 2018, 21(4), 287–295, DOI:10.3779/j.issn.1009-3419.2018.04.11.
- L. Franco-Martinez, A. Tvarijonaviciute and S. Martinez-Subiela,
et al., Chemiluminescent assay as an alternative to radioimmunoassay for the measurement of cortisol in plasma and skin mucus of Oncorhynchus mykiss, Ecol. Indic., 2019, 98, 634–640, DOI:10.1016/j.ecolind.2018.11.046.
- A. R. Alaejos, S. A. Klaassen and S. E. Fernández,
et al., Urine-free cortisol assessment using immunoassay and gas chromatography coupled to mass spectrometry (GC-MS) - Result review, Clin. Chim. Acta, 2019, 493, S340–S341, DOI:10.1016/j.cca.2019.03.736.
- D. Liang and H. Lu, Salivary biological biomarkers for Alzheimer's disease, Arch. Oral Biol., 2019, 105, 5–12, DOI:10.1016/j.archoralbio.2019.06.004.
- N. L. Kazzanskiy, M. A. Butt and S. N. Khonina,
et al., Recent Advances in Wearable Optical Sensor Automation Powered by Battery versus Skin-like Battery-Free Devices for Personal Healthcare-A Review, Nanomaterials, 2022, 12(3), 334, DOI:10.3390/nano12030334.
- R. A. Nawrocki, H. Jin and L. Sunghoon,
et al., Self-Adhesive and Ultra-Conformable, Sub-300 nm Dry Thin-Film Electrodes for Surface Monitoring of Biopotentials, Adv. Funct. Mater., 2018, 28(36), 1803279, DOI:10.1002/adfm.201803279.
- H. Xu, J. Liu and J. Zhang,
et al., Flexible Organic/Inorganic Hybrid Near-Infrared Photoplethysmogram Sensor for Cardiovascular Monitoring, Adv. Mater., 2017, 29(31), 1700975, DOI:10.1002/adma.201700975.
- E. Jeon and H. A. Park, Factors affecting acceptance of smartphone application for management of obesity, Healthc. Inform. Res., 2015, 21(2), 74–82, DOI:10.4258/hir.2015.21.2.74.
- E. Jeon and H. A. Park, Development of a smartphone application for clinical-guideline-based obesity management, Healthc. Inform. Res., 2015, 21(1), 10–20, DOI:10.4258/hir.2015.21.1.10.
- A. Darwish and A. E. Hassanien, Wearable and implantable wireless sensor network solutions for healthcare monitoring, Sensors, 2011, 11(6), 5561–5595, DOI:10.3390/s110605561.
- E. A. Fatema and N. M. Ismail, Are Currently Available Wearable Devices for Activity Tracking and Heart Rate Monitoring Accurate, Precise, and Medically Beneficial?, Healthc. Inform. Res., 2015, 21(4), 315–320, DOI:10.4258/hir.2015.21.4.315.
- S. Gillinov, M. Etiwy and R. Wang,
et al., Variable Accuracy of Wearable Heart Rate Monitors during Aerobic Exercise, Med. Sci. Sports Exercise, 2017, 49(8), 1697–1703, DOI:10.1249/MSS.0000000000001284.
- M. A. Yokus and M. A. Daniele, Integrated non-invasive biochemical and biophysical sensing systems for health and performance monitoring: A systems perspective, Biosens. Bioelectron., 2021, 184, 113249, DOI:10.1016/j.bios.2021.113249.
- S. Anastasova, B. Crewther and P. Bembnowicz,
et al., A wearable multisensing patch for continuous sweat monitoring, Biosens. Bioelectron., 2017, 93, 139–145, DOI:10.1016/j.bios.2016.09.038.
- W. Gao, S. Emaminejad and H. Nyein,
et al., Fully integrated wearable sensor arrays for multiplexed in situ perspiration analysis, Nature, 2016, 529(7587), 509–514, DOI:10.1038/nature16521.
- Y. Yamamoto, D. Yamamoto and M. Takada,
et al., Efficient Skin Temperature Sensor and Stable Gel-Less Sticky ECG Sensor for a Wearable Flexible Healthcare Patch, Adv. Healthcare Mater., 2017, 6(17), 1700495, DOI:10.1002/adhm.201700495.
- L. Manjakkal, S. Dervin and R. Dahiya,
et al., Flexible potentiometric pH sensors for wearable systems, RSC Adv., 2020, 10(15), 8594–8617, 10.1039/d0ra00016g.
- J. Pan, Z. Zhang and C. P. Jiang,
et al., A multifunctional skin-like wearable optical sensor based on an optical micro-/nanofibre, Nanoscale, 2020, 12(33), 17538–17544, 10.1039/d0nr03446k.
- Y. Z. Chen, Y. Xi and Y. J. Ke,
et al., A skin-like stretchable colorimetric temperature sensor, Sci. China Mater., 2018, 61(7), 969–976, DOI:10.1007/s40843-018-9266-8.
- J. J. Wei, J. J. Xie and P. C. Zhang,
et al., Bioinspired 3D Printable, Self-Healable, and Stretchable Hydrogels with Multiple Conductivities for Skin-like Wearable Strain Sensors, ACS Appl. Mater. Interfaces, 2021, 13(2), 2952–2960, DOI:10.1021/acsami.0c19512.
- K. Sim, Z. Rao and Z. Zou,
et al., Metal oxide semiconductor nanomembrane–based soft unnoticeable multifunctional electronics for wearable human-machine interfaces, Sci. Adv., 2019, 5(8), eaav9653, DOI:10.1126/sciadv.aav9653.
- Y. B. Yang, X. D. Yang and Y. N. Tan,
et al., Recent progress in flexible and wearable bio-electronics based on nanomaterials, Nano Res., 2017, 10(5), 1560–1583, DOI:10.1007/s12274-017-1476-8.
- C. R. Liu, S. J. Han and H. H. Xu,
et al., Multifunctional Highly Sensitive Multiscale Stretchable Strain Sensor Based on a Graphene/Glycerol-KCl Synergistic Conductive Network, ACS Appl. Mater. Interfaces, 2018, 10(37), 31716–31724, DOI:10.1021/acsami.8b12674.
- Z. Zhao, C. Huang and Z. Huang,
et al., Advancements in electrochemical biosensing for respiratory virus detection: A review, TrAC, Trends Anal. Chem., 2021, 139, 116253, DOI:10.1016/j.trac.2021.116253.
- N. Dhull, G. Kaur and V. Gupta,
et al., Highly sensitive and non-invasive electrochemical immunosensor for salivary cortisol detection, Sens. Actuators, B, 2019, 293, 281–288, DOI:10.1016/j.snb.2019.05.020.
- F. Xie, M. Yang and M. Jiang,
et al., Carbon-based nanomaterials – A promising electrochemical sensor toward persistent toxic substance, TrAC, Trends Anal. Chem., 2019, 119, 115624, DOI:10.1016/j.trac.2019.115624.
- Y. B. Yang, X. D. Yang and Y. J. Yang,
et al., Aptamer-functionalized carbon nanomaterials electrochemical sensors for detecting cancer relevant biomolecules, Carbon, 2018, 129, 380–395, DOI:10.1016/j.carbon.2017.12.013.
- S. Manivannan, I. Kang and Y. Seo,
et al., M13 Virus-Incorporated Biotemplates on Electrode Surfaces to Nucleate Metal Nanostructures by Electrodeposition, ACS Appl. Mater. Interfaces, 2017, 9(38), 32965–32976, DOI:10.1021/acsami.7b06545.
- L. T. Qian, S. Durairaj and S. Prins,
et al., Nanomaterial-based electrochemical sensors and biosensors for the detection of pharmaceutical compounds, Biosens. Bioelectron., 2021, 175, 112836, DOI:10.1016/j.bios.2020.112836.
- W. Wang, P. X. Ma and H. Dong,
et al., A magnetic nanoparticles relaxation sensor for protein-protein interaction detection at ultra-low magnetic field, Biosens. Bioelectron., 2016, 80(1), 661–665, DOI:10.1016/j.bios.2016.02.037.
- E. A. Afshar, M. A. Taher and H. K. Maleh,
et al., Magnetic nanoparticles based on cerium MOF supported on the MWCNT as a fluorescence quenching sensor for determination of 6-mercaptopurine, Environ. Pollut., 2022, 305, 119230, DOI:10.1016/j.envpol.2022.119230.
- Y. Y. Liu, Z. X. Cai and L. Sheng,
et al., A magnetic relaxation switching and visual dual-mode sensor for selective detection of Hg2+ based on aptamers modified Au@Fe3O4 nanoparticles, J. Hazard. Mater., 2020, 388, 121728, DOI:10.1016/j.jhazmat.2019.121728.
- H. D. Cancar, S. Soylemez and Y. Akpinar,
et al., A Novel Acetylcholinesterase Biosensor: Core–Shell Magnetic Nanoparticles Incorporating a Conjugated Polymer for the Detection of Organophosphorus Pesticides, ACS Appl. Mater. Interfaces, 2016, 8(12), 8058–8067, DOI:10.1021/acsami.5b12383.
- S. H. Li, T. R. Tian and T. Zhang,
et al., Advances in biological applications of self-assembled DNA tetrahedral nanostructures, Mater. Today, 2019, 24(3), 57–68, DOI:10.1016/j.mattod.2018.08.002.
- Y. C. Zhao, B. Wang and H. Hojaiji,
et al., A wearable freestanding electrochemical sensing system, Sci. Adv., 2020, 6(12), eaaz0007, DOI:10.1126/sciadv.aaz0007.
- M. Gamella, S. Campuzano and J. Manso,
et al., A novel non-invasive electrochemical biosensing device for in situ determination of the alcohol content in blood by monitoring ethanol in sweat, Anal. Chim. Acta, 2014, 806, 1–7, DOI:10.1016/j.aca.2013.09.020.
- A. M. V. Mohan, J. R. Windmiller and R. K. Mishra,
et al., Continuous minimally-invasive alcohol monitoring using microneedle sensor arrays, Biosens. Bioelectron., 2017, 91(1), 574–579, DOI:10.1016/j.bios.2017.01.016.
- J. H. Li, J. H. Chen and F. Xu,
et al., Sensitive and Wearable Optical Microfiber Sensor for Human Health Monitoring, Adv. Mater. Technol., 2018, 3(12), 1800296, DOI:10.1002/admt.201800296.
- G. Y. Bae, S. W. Pak and D. Kim,
et al., Linearly and Highly Pressure-Sensitive Electronic Skin Based on a Bioinspired Hierarchical Structural Array, Adv. Mater., 2016, 28(26), 5300–5306, DOI:10.1002/adma.201600408.
- X. L. Fu, H. L. Dong and Y. G. Zhen,
et al., Solution-processed large-area nanocrystal arrays of metal-organic frameworks as wearable, ultrasensitive, electronic skin for health monitoring, Small, 2015, 11(27), 3351–3356, DOI:10.1002/smll.201402890.
- L. W. Lo, H. Y. Shi and H. C. Wan,
et al., Inkjet-Printed Soft Resistive Pressure Sensor Patch for Wearable Electronics Applications, Adv. Mater. Technol., 2020, 5(1), 1900717, DOI:10.1002/admt.201900717.
- Y. Kim, T. Lim and C.-H. Kim,
et al., Organic electrochemical transistor-based channel dimension-independent single-strand wearable sweat sensors, NPG Asia Mater., 2018, 10(11), 1086–1095, DOI:10.1038/s41427-018-0097-3.
- S. Yao, P. Swetha and Y. Zhu,
et al., Nanomaterial-Enabled Wearable Sensors for Healthcare, Adv. Healthcare Mater., 2018, 7(1), 1700889, DOI:10.1002/adhm.201700889.
- P. C. Ferreira, V. N. Ataíde and C. L. S. Chagas,
et al., Wearable electrochemical sensors for forensic and clinical applications, TrAC, Trends Anal. Chem., 2019, 19, 115622, DOI:10.1016/j.trac.2019.115622.
- S. Anastasova, B. Crewther and P. Bembnowicz,
et al., A wearable multisensing patch for continuous sweat monitoring, Biosens. Bioelectron., 2017, 93, 139–145, DOI:10.1016/j.bios.2016.09.038.
- H. W. Chow and C. C. Yang, Accuracy of Optical Heart Rate Sensing Technology in Wearable Fitness Trackers for Young and Older Adults: Validation and Comparison Study, JMIR Mhealth Uhealth, 2019, 8, e14707, DOI:10.2196/14707.
- T. L. Chen, Y. L. Lo and C. C. Liao,
et al., Noninvasive measurement of glucose concentration on human fingertip by optical coherence tomography, J. Biomed. Opt., 2018, 23, 1, DOI:10.1117/1.JBO.23.4.047001.
- C. Ash, M. Dubec and K. Donne,
et al., Effect of wavelength and beam width on penetration in light-tissue interaction using computational methods, Lasers Med. Sci., 2017, 32, 1909–1918, DOI:10.1007/s10103-017-2317-4.
- A. Tarar, U. Mohammad and S. K. Srivastava,
et al., Wearable Skin Sensors and Their Challenges: A Review of Transdermal, Optical, and Mechanical Sensors, Biosensors, 2020, 10, 56, DOI:10.3390/bios10060056.
- W. Y. X. Yang, Y. X. Ma and H. Sun,
et al., Molecularly imprinted polymers based optical fiber sensors: A review, TrAC, Trends Anal. Chem., 2022, 152, 116608, DOI:10.1016/j.trac.2022.116608.
- Y. J. Zhang, X. Y. Li and J. Kim,
et al., Thermally Drawn Stretchable Electrical and Optical Fiber Sensors for Multimodal Extreme Deformation Sensing, Adv. Opt. Mater., 2021, 9(6), 1–9, DOI:10.1002/adom.202001815.
- G. G. Liu and K. W. Li, Micro/nano optical fibers for label-free detection of abrin with high sensitivity, Sens. Actuators, B, 2015, 215, 146–151, DOI:10.1016/j.snb.2015.03.056.
- L. Y. Li, Y. F. Liu and C. Y. Song,
et al., Wearable Alignment-Free Microfber-Based Sensor Chip for Precise Vital Signs Monitoring and Cardiovascular Assessment, Adv. Fiber Mater., 2022, 4(3), 475–486, DOI:10.1007/s42765-021-00121-8.
- Y. Tang, H. T. Liu and J. Pan,
et al., Optical Micro/Nanofiber-Enabled Compact Tactile Sensor for Hardness Discrimination, ACS Appl. Mater. Interfaces, 2021, 12(3), 4560–4566, DOI:10.1021/acsami.0c20392.
- H. T. Zhu, L. W. Zhan and Q. Dai,
et al., Self-Assembled Wavy Optical Microfiber for Stretchable Wearable Sensor, Adv. Opt. Mater., 2021, 9(11), 2170042, DOI:10.1002/adom.202002206.
- J. J. Guo, K. J. Zhao and B. Q. Zhou,
et al., Wearable and Skin-Mountable Fiber-Optic Strain Sensors Interrogated by a Free-Running, Dual-Comb Fiber Laser, Adv. Opt. Mater., 2019, 7(12), 1900086, DOI:10.1002/adom.201900086.
- J. Pan, C. P. Jiang and Z. Zhang,
et al., Flexible Liquid-Filled Fiber Adapter Enabled Wearable Optical Sensors, Adv. Mater. Technol., 2020, 5(6), 1–7, DOI:10.1002/admt.202000079.
- Y. H. Liu, M. Pharr and G. A. Salvatore,
et al., Lab-on-Skin: A Review of Flexible and Stretchable Electronics for Wearable Health Monitoring, ACS Nano, 2017, 11(10), 9614–9635, DOI:10.1021/acsnano.7b04898.
- X. Z. Chen, X. L. Tong and C. Zhang,
et al., High-precision optical fiber Fabry–Perot composite sensor for pressure and temperature, Opt. Commun., 2022, 506(1), 127580, DOI:10.1016/j.optcom.2021.127580.
- J. Heikenfeld, A. Jajack and J. Rogers,
et al., Wearable sensors: modalities, challenges, and prospects, Lab Chip, 2018, 18(2), 217–248, 10.1039/c7lc00914c.
- S. A. Filatova, I. A. Shcherbakov and V. B. Tsvetkov,
et al., Optical properties of animal tissues in the wavelength range from 350 to 2600 nm, J. Biomed. Opt., 2017, 22(3), 035009, DOI:10.1117/1.JBO.22.3.035009.
- Z. F. Xu, J. F. Yao and Z. Wang,
et al., Development of a Portable Electrical Impedance Tomography System for Biomedical Applications, IEEE Sens. J., 2018, 18(19), 8117–8124, DOI:10.1109/JSEN.2018.2864539.
- J. F. Yao and M. Takei, Application of Process Tomography to Multiphase Flow Measurement in Industrial and Biomedical Fields: A Review, IEEE Sens. J., 2017, 17(24), 8196–8205, DOI:10.1109/JSEN.2017.2682929.
- J. F. Yao, H. J. Chen and Z. F. Xu,
et al., Development of a Wearable Electrical Impedance Tomographic Sensor for Gesture Recognition with Machine Learning, IEEE J. Biomed. Health Inform., 2020, 24(6), 1550–1556, DOI:10.1109/JBHI.2019.2945593.
- A. Boyle and A. Adler, The impact of electrode area, contact impedance and boundary shape on EIT images, Physiol. Meas., 2011, 32, 745–754, DOI:10.1088/0967-3334/32/7/S02.
- P. Bogonez-Franco, L. Nescolarde and R. Bragos,
et al., Measurement errors in multifrequency bioelectrical impedance analyzers with and without impedance electrode mismatch, Physiol. Meas., 2009, 30, 573–587, DOI:10.1088/0967-3334/30/7/004.
- L. Corchia, G. Monti and F. Raheli,
et al., Dry Textile Electrodes for Wearable Bio-Impedance Analyzers, IEEE Sens. J., 2020, 20(11), 6139–6147, DOI:10.1109/JSEN.2020.2972603.
- M. H. Jung, K. Namkoong and Y. Lee,
et al., Wrist-wearable bioelectrical impedance analyzer with miniature electrodes for daily obesity management, Sci. Rep., 2021, 11(1), 1238, DOI:10.1038/s41598-020-79667-3.
- J. M. Benítez and F. J. Montáns, The mechanical behavior of skin: Structures and models for the finite element analysis, Comput. Struct., 2017, 190, 75–107, DOI:10.1016/j.compstruc.2017.05.003.
- K. H. Kim, S. K. Hong and N. S. Jang,
et al., Wearable Resistive Pressure Sensor Based on Highly Flexible Carbon Composite Conductors with Irregular Surface Morphology, ACS Appl. Mater. Interfaces, 2017, 9(20), 17499–17507, DOI:10.1021/acsami.7b06119.
- X. Q. Liao, Z. Zhang and Z. Kang,
et al., Ultrasensitive and Stretchable Resistive Strain Sensors Designed for Wearable Electronics, Mater. Horiz., 2017, 4(3), 502–510, 10.1039/c7mh00071e.
- D. Kang, P. V. Pikhitsa and Y. W. Choi,
et al., Ultrasensitive mechanical crack-based sensor inspired by the spider sensory system, Nature, 2014, 516(7530), 222–226, DOI:10.1038/nature14002.
- Y. P. Zang, F. J. Zhang and C. Di,
et al., Advances of flexible pressure sensors toward artificial intelligence and health care applications, Mater. Horiz., 2014, 2(2), 140–156, 10.1039/c4mh00147h.
- Y. T. Wu, T. Yan and Z. J. Pan,
et al., Wearable Carbon-Based Resistive Sensors for Strain Detection: A Review, IEEE Sens. J., 2021, 21(4), 4030–4043, DOI:10.1109/JSEN.2020.3034453.
- X. Xuan, H. S. Yoon and J. Y. Park,
et al., A Wearable Electrochemical Glucose Sensor based on Simple and Low-Cost Fabrication Supported Micro-Patterned Reduced Graphene Oxide Nanocomposite Electrode on Flexible Substrate, Biosens. Bioelectron., 2018, 109, 75–82, DOI:10.1016/j.bios.2018.02.054.
- Z. Y. Wang, X. Guan and H. Y. Huang,
et al., Full 3D Printing of Stretchable Piezoresistive Sensor with Hierarchical Porosity and Multimodulus Architecture, Adv. Funct. Mater., 2018, 29(11), 1807569, DOI:10.1002/adfm.201807569.
- L. R. Wang, T. L. Xu and X. J. Zhang,
et al., Multifunctional conductive hydrogel-based flexible wearable sensors, TrAC, Trends Anal. Chem., 2018, 134, 116130, DOI:10.1016/j.trac.2020.116130.
- H.-R. Lee, C.-C. Kim and J.-Y. Sun,
et al., Stretchable Ionics - A Promising Candidate for Upcoming Wearable Devices, Adv. Mater., 2021, 30(42), 1704403, DOI:10.1002/adma.201704403.
- Q. H. Wang, X. F. Pan and C. M. Lin,
et al., Biocompatible, self-wrinkled, antifreezing and stretchable hydrogel-based wearable sensor with PEDOT: sulfonated lignin as conductive materials, Chem. Eng. J., 2019, 370, 1039–1047, DOI:10.1016/j.cej.2019.03.287.
- G. F. Cai, J. X. Wang and K. Qian,
et al., Extremely Stretchable Strain Sensors Based on Conductive Self-Healing Dynamic Cross-Links Hydrogels for Human-Motion Detection, Adv. Sci., 2017, 4(2), 1600190, DOI:10.1002/advs.201600190.
- K. Nagamine, T. Mano and A. Namura,
et al., Noninvasive Sweat-Lactate Biosensor Emplsoying a Hydrogel-Based Touch Pad, Sci. Rep., 2019, 9(1), 10102, DOI:10.1038/s41598-019-46611-z.
- H. X. Zhang, W. B. Niu and S. F. Zhang,
et al., Extremely Stretchable, Stable and Durable Strain Sensors Based on Double-Network Organogels, ACS Appl. Mater. Interfaces, 2018, 10(38), 32640–32648, DOI:10.1021/acsami.8b08873.
- M. Amjadi, K. U. Kyung and I. Park,
et al., Stretchable, Skin-Mountable, and Wearable Strain Sensors and Their Potential Applications: A Review, Adv. Funct. Mater., 2016, 26(11), 1678–1698, DOI:10.1002/adfm.201504755.
- R. C. Webb, A. P. Bonifas and A. Behnaz,
et al., Ultrathin conformal devices for precise and continuous thermal characterization of human skin, Nat. Mater., 2013, 12(11), 1078, DOI:10.1038/NMAT3755.
- C. Pang, G.-Y. Lee and T. Kim,
et al., A flexible and highly sensitive strain-gauge sensor using reversible interlocking of nanofibres, Nat. Mater., 2012, 11(9), 795–801, DOI:10.1038/nmat3380.
- M. Amjadi, A. Pichitpajongkit and S. Lee,
et al., Highly Stretchable and Sensitive Strain Sensor Based on Silver Nanowire–Elastomer Nanocomposite, ACS Nano, 2014, 8(5), 5154–5163, DOI:10.1021/nn501204t.
- N. S. Lu, C. Lu and S. X. Yang,
et al., Highly Sensitive Skin-Mountable Strain Gauges Based Entirely on Elastomers, Adv. Funct. Mater., 2012, 22(19), 4044–4050, DOI:10.1002/adfm.201200498.
- C. Pang, C. Lee and K. Y. Suh,
et al., Recent advances in flexible sensors for wearable and implantable devices, J. Appl. Polym. Sci., 2013, 130(3), 1429–1441, DOI:10.1002/app.39461.
- P. C. Zhu, Y. L. Wang and Y. Wang,
et al., Flexible 3D Architectured Piezo/Thermoelectric Bimodal Tactile Sensor Array for E-Skin Application, Adv. Energy Mater., 2020, 10(39), 1–8, DOI:10.1002/aenm.202001945.
- D. K. Kwon and J. M. Myoung, Wearable and Semitransparent Pressure-Sensitive Light-Emitting Sensor Based on Electrochemiluminescence, ACS Nano, 2020, 14(7), 8716–8723, DOI:10.1021/acsnano.0c03186.
- C. Liu, R. Correia and H. K. Ballaji,
et al., Optical Fibre-Based Pulse Oximetry Sensor with Contact Force Detection, Sensors, 2018, 18(11), 3632, DOI:10.3390/s18113632.
- H. Lee, W. Lee and H. Lee,
et al., Organic–Inorganic Hybrid Approach to Pulse Oximetry Sensors with Reliability and Low Power Consumption, ACS Photonics, 2021, 8(12), 3564–3572, DOI:10.1021/acsphotonics.1c01161.
- Z. Y. Zhan, R. Z. Lin and V.-T. Tran,
et al., Paper-Carbon Nanotube Based Wearable Pressure Sensor for Physiological Signal Acquisition and Soft Robotic Skin, ACS Appl. Mater. Interfaces, 2017, 9(43), 37921–37928, DOI:10.1021/acsami.7b10820.
- Z.-Y. Zhan, Y.-N. Zhang and G.-Z. Sun,
et al., The Effects of Catalyst Treatment on Fast Growth of Millimeter-Long Multi-walled Carbon Nanotube Arrays, Appl. Surf. Sci., 2011, 257(17), 7704–7708, DOI:10.1016/j.apsusc.2011.04.013.
- J. An, Z. Zhan and G. Sun,
et al., Direct Preparation of Carbon Nanotube Intramolecular Junctions on Structured Substrates, Sci. Rep., 2016, 6, 38032, DOI:10.1038/srep38032.
- J. An, Z. Zhan and S. H. Krishna,
et al., Growth Condition Mediated Catalyst Effects on the Density and Length of Horizontally Aligned Single-Walled Carbon Nanotube Arrays, Chem. Eng. J., 2014, 237, 16–22, DOI:10.1016/j.cej.2013.10.012.
- J. An, Z. Zhan and H. K. S. V. Mohan,
et al., Trench Structure Assisted Alignment in Ultralong and Dense Carbon Nanotube Arrays, J. Mater. Chem., 2015, 3(10), 2215–2222, 10.1039/c4tc02317j.
- D.-H. Kim, N. Lu and R. Ma,
et al., Epidermal electronics, Science, 2011, 333(6044), 838–843, DOI:10.1126/science.1206157.
- V. Kedambaimoole, N. Kumar and V. Shirhatti,
et al., Laser-Induced Direct Patterning of Free standing Ti3C2-MXene Films for Skin Conformal Tattoo Sensors, ACS Sens., 2020, 5(7), 2086–2095, DOI:10.1021/acssensors.0c00647.
- S. K. Ameri, R. Ho and H. Jang,
et al., Graphene Electronic Tattoo Sensors, ACS Nano, 2017, 11(8), 7634–7641, DOI:10.1021/acsnano.7b02182.
- P. C. Zhu, Y. L. Wang and Y. Wang,
et al., Flexible 3D Architectured Piezo/Thermoelectric Bimodal Tactile Sensor Array for E-Skin Application, Adv. Energy Mater., 2020, 10(39), 1–8, DOI:10.1002/aenm.202001945.
- G. Lee, G. Y. Bae and J. H. Son,
et al., User-Interactive Thermotherapeutic Electronic Skin Based on Stretchable Thermochromic Strain Sensor, Adv. Sci., 2020, 7(17), 2001184, DOI:10.1002/advs.202001184.
- H. Charaya, T.-G. La and J. Rieger,
et al., Thermochromic and Piezocapacitive Flexible Sensor Array by Combining Composite Elastomer Dielectrics and Transparent Ionic Hydrogel Electrodes, Adv. Mater. Technol., 2019, 4(9), 1900327, DOI:10.1002/admt.201900327.
- A. Chhetry, S. Sharma and S. C. Barman,
et al., Black Phosphorus@Laser-Engraved Graphene Heterostructure-Based Temperature–Strain Hybridized Sensor for Electronic-Skin Applications, Adv. Funct. Mater., 2021, 31(10), 1–14, DOI:10.1002/adfm.202007661.
- S. Nakata, M. Shiomi and Y. Fujita,
et al., A wearable pH sensor with high sensitivity based on a flexible charge-coupled device, Nat. Electron., 2018, 1(11), 596–603, DOI:10.1038/s41928-018-0162-5.
- L. Manjakkal, S. Dervin and R. Dahiya,
et al., Flexible potentiometric pH sensors for wearable systems, RSC Adv., 2020, 10(15), 8594–8617, 10.1039/d0ra00016g.
- J. Kim, A. S. Campbell and J. Wang,
et al., Wearable non-invasive epidermal glucose sensors: A review, Talanta, 2018, 177, 163–170, DOI:10.1016/j.talanta.2017.08.077.
- L. C. Tai, W. Gao and M. Chao,
et al., Methylxanthine Drug Monitoring with Wearable Sweat Sensors, Adv. Mater., 2018, 30, 1707442, DOI:10.1002/adma.201707442.
- K. Y. Goud, C. Moonla and R. K. Mishra,
et al., Wearable Electrochemical Microneedle Sensor for Continuous Monitoring of Levodopa: Toward Parkinson Management, ACS Sens., 2019, 4(8), 2196–2204, DOI:10.1021/acssensors.9b01127.
- X. V. Chen, M. P. S. Mousavi and P. Bühlmann,
et al., Fluorous-Phase Ion-Selective pH Electrodes: Electrode Body and Ionophore Optimization for Measurements in the Physiological pH Range, ACS Omega, 2020, 5(23), 13621–13629, DOI:10.1021/acsomega.0c00582.
- T. Guinovart, M. Parrilla and G. A. Crespo,
et al., Potentiometric sensors using cotton yarns, carbon nanotubes and polymeric membranes, Analyst, 2013, 138(18), 5208–5215, 10.1039/c3an00710c.
- H. Y. Y. Nyein, W. Gao and Z. Shahpar,
et al., A Wearable Electrochemical Platform for Noninvasive Simultaneous Monitoring of Ca2+ and pH, ACS Nano, 2016, 10(7), 7216–7224, DOI:10.1021/acsnano.6b04005.
- M. Bariya, H. Y. Y. Nyein and N. Javey,
et al., Wearable sweat sensors, Nat. Electron., 2018, 1, 160–171, DOI:10.1038/s41928-018-0043-y.
- M. Protti, C. Marasca and M. Cirrincione,
et al., Assessment of capillary volumetric blood microsampling for the analysis of central nervous system drugs and metabolites, Analyst, 2020, 145(17), 5744–5753, 10.1039/d0an01039a.
- Y. Y. Yu, S. H. Peng and P. Blanloeuil,
et al., Wearable Temperature Sensors with Enhanced Sensitivity by Engineering Microcrack Morphology in PEDOT: PSS–PDMS Sensors, ACS Appl. Mater. Interfaces, 2020, 12(32), 36578–36588, DOI:10.1021/acsami.0c07649.
- Y. Yamamoto, D. Yamamoto and M. Takada,
et al., Efficient Skin Temperature Sensor and Stable Gel-Less Sticky ECG Sensor for a Wearable Flexible Healthcare Patch, Adv. Healthcare Mater., 2017, 6(17), 1700495, DOI:10.1002/adhm.201700495.
- T. Q. Trung, H. S. Le and T. M. L. Dang,
et al., Freestanding, Fiber-Based, Wearable Temperature Sensor with Tunable Thermal Index for Healthcare Monitoring, Adv. Healthcare Mater., 2018, 7(12), 1800074, DOI:10.1002/adhm.201800074.
- S. W. Chen, J. M. Qi and S. C. Fan,
et al., Flexible Wearable Sensors for Cardiovascular Health Monitoring, Adv. Healthcare Mater., 2021, 10(17), e2100116, DOI:10.1002/adhm.202100116.
- M. Lon, S. Dinulescu and B. Firtat,
et al., Design and Fabrication of a New Wearable Pressure Sensor for Blood Pressure Monitoring, Sensors, 2021, 21(6), 2075, DOI:10.3390/s21062075.
- S. W. Chen, N. Wu and S. Z. Lin,
et al., Hierarchical elastomer tuned self-powered pressure sensor for wearable multifunctional cardiovascular electronics, Nano Energy, 2020, 70, 104460, DOI:10.1016/j.nanoen.2020.104460.
- D.-H. Kim, N. Lu and R. Ghaffari,
et al., Materials for multifunctional balloon catheters with capabilities in cardiac electrophysiological mapping and ablation therapy, Nat. Mater., 2011, 10, 316–323, DOI:10.1038/nmat2971.
- D. Son, J. Lee and S. Qiao,
et al., Multifunctional wearable devices for diagnosis and therapy of movement disorders, Nat. Nanotechnol., 2014, 9, 397–404, DOI:10.1038/NNANO.2014.38.
- M. Ying, A. P. Bonifas and N. S. Lu,
et al., Silicon nanomembranes for fingertip electronics, Nanotechnology, 2012, 23, 344004, DOI:10.1088/0957-4484/23/34/344004.
- C. Frigo, M. Ferrarin and W. Frasson,
et al., EMG signals detection and processing for on-line control of functional electrical stimulation, J. Electromyogr. Kinesiol., 2000, 10, 351–360, DOI:10.1016/S1050-6411(00)00026-2.
- J. D. Loeser, R. G. Black and A. Christman,
et al., Relief of pain by transcutaneous stimulation, J. Neurosurg., 1975, 42, 308–314, DOI:10.3171/jns.1975.42.3.0308.
- C. Antfolk, M. D'Alonzo and B. Rosén,
et al., Sensory feedback in upper limb prosthetics, Expert Rev. Med. Devices, 2013, 10(1), 45–54, DOI:10.1586/erd.12.68.
- A. Santos, E. Fortunato and R. Martins,
et al., E-Skin Piezoresistive Pressure Sensor Combining Laser Engraving and Shrinking Polymeric Films for Health Monitoring Applications, Adv. Mater. Interfaces, 2021, 8(21), 2100877, DOI:10.1002/admi.202100877.
- S. Y. Zhang, C. C. Lin and Z. Z. Xia,
et al., A Facile and Novel Design of Multifunctional Electronic Skin Based on Polydimethylsiloxane with Micropillars for Signal Monitoring, J. Mater. Chem. B, 2020, 8(36), 8315–8322, 10.1039/D0TB00954G.
- X. Q. Liao, W. T. Song and X. Y. Zhang,
et al., Directly printed wearable electronic sensing textiles towards human-machine interfaces, J. Mater. Chem. C, 2018, 6(47), 12841–12848, 10.1039/C8TC02655F.
- N. Yu, X. H. Zhan and S. N. Zhao,
et al., A Precise Dead Reckoning Algorithm Based on Bluetooth and Multiple Sensors, IEEE Internet Things J., 2018, 5(1), 336–351, DOI:10.1109/JIOT.2017.2784386.
- H. Liu and C. Zhao, Wearable electrochemical sensors for noninvasive monitoring of health—a perspective, Curr. Opin. Electrochem., 2020, 23, 42–46, DOI:10.1016/j.coelec.2020.03.008.
- Y. Liu, R. Bao and J. Tao,
et al., Recent progress in tactile sensors and their applications in intelligent systems, Sci. Bull., 2020, 65(1), 70–88, DOI:10.1016/j.scib.2019.10.021.
- S. Chun, W. Son and D. Wan,
et al., Water-Resistant and Skin-Adhesive Wearable Electronics Using Graphene Fabric Sensor with Octopus-Inspired Microsuckers, ACS Appl. Mater. Interfaces, 2019, 11(18), 16951–16957, DOI:10.1021/acsami.9b04206.
- J. Edwards, Wearables-Fashion With a Purpose: A New Generation of Wearable Devices Uses Signal Processing to Make Life Easier, Healthier, and More Secure, IEEE Signal Process. Mag., 2021, 38(2), 15–136, DOI:10.1109/MSP.2020.3044001.
- Y. F. Hu, T. Q. Huang and H. J. Zhang,
et al., Ultrasensitive and Wearable Carbon Hybrid Fiber Devices as Robust Intelligent Sensors, ACS Appl. Mater. Interfaces, 2021, 13(20), 23905–23914, DOI:10.1021/acsami.1c03615.
- P. Q. Wei, X. Yang and Z. M. Cao,
et al., Flexible and Stretchable Electronic Skin with High Durability and Shock Resistance via Embedded 3D Printing Technology for Human Activity Monitoring and Personal Healthcare, Adv. Mater. Technol., 2019, 4(9), 1900315, DOI:10.1002/admt.201900315.
- X. F. Zhao, C. Z. Hang and H. L. Lu,
et al., A skin-like sensor for intelligent Braille recognition, Nano Energy, 2020, 68, 104346, DOI:10.1016/j.nanoen.2019.104346.
- X. L. Huo and M. Ghovanloo, Tongue drive: A wireless tongue-operated means for people with severe disabilities to communicate their intentions, IEEE Commun. Mag., 2012, 50(10), 128–135, DOI:10.1109/MCOM.2012.6316786.
- S. Mishra, J. J. S. Norton and Y. Lee,
et al., Soft, conformal bioelectronics for a wireless human-wheelchair interface, Biosens. Bioelectron., 2017, 91(1), 796–803, DOI:10.1016/j.bios.2017.01.044.
- W. R. Thompson, Worldwide survey of fitness trends for 2016, ACSMs Health Fit. J, 2015, 19(6), 9–18, DOI:10.1249/FIT.0000000000000164.
- W. R. Thompson, Worldwide survey of fitness trends for 2017, ACSMs Health Fit. J., 2016, 20(6), 8–17, DOI:10.1249/fit.0000000000000252.
- W. R. Thompson, Worldwide survey of fitness trends for 2019, ACSMs Health Fit. J., 2018, 22(6), 10–17, DOI:10.1249/fit.0000000000000438.
- W. R. Thompson, Worldwide survey of fitness trends for 2020, ACSMs Health Fit. J., 2019, 23(6), 10–18, DOI:10.1249/fit.0000000000000526.
- K. H. Shelley, Photoplethysmography:
Beyond the Calculation of Arterial Oxygen Saturation and Heart Rate, Anesth. Analg., 2007, 105(6), S31–S36, DOI:10.1213/01.ane.0000269512.82836.c9.
- J. Allen, Photoplethysmography and its application in clinical physiological measurement, Physiol. Meas., 2007, 28(3), R1–R39, DOI:10.1088/0967-3334/28/3/R01.
- L. G. Lindberg, H. Ugnell and P. A. Oberg,
et al., Monitoring of respiratory and heart rates using a fibre-optic sensor, Med. Biol. Eng. Comput., 1992, 30(5), 533–537, DOI:10.1007/BF02457833.
- A. Shcherbina, C. M. Mattsson and D. Waggott,
et al., Accuracy in Wrist-Worn, Sensor-Based Measurements of Heart Rate and Energy Expenditure in a Diverse Cohort, J. Pers. Med., 2017, 7(2), 3, DOI:10.3390/jpm7020003.
- B. Bent, B. A. Goldstein and W. A. Kibbe,
et al., Investigating sources of inaccuracy in wearable optical heart rate sensors, NPJ Digit. Med., 2020, 3(1), 18, DOI:10.1038/s41746-020-0226-6.
- M. Etiwy, Z. Akhrass and L. Gillinov,
et al., Accuracy of wearable heart rate monitors in cardiac rehabilitation, Cardiovasc. Diagn. Ther., 2019, 9(3), 262–271, DOI:10.21037/cdt.2019.04.08.
- M. P. Wallen, S. R. Gomersall and S. E. Keating,
et al., Accuracy of Heart Rate Watches: Implications for Weight Management, PLoS Biol., 2016, 11(5), e0154420, DOI:10.1371/journal.pone.0154420.
- G. H. Lee, H. Kang and J. W. Chung,
et al., Stretchable PPG sensor with light polarization for physical activity-permissible monitoring, Sci. Adv., 2022, 8(15), eabm 3622, DOI:10.1126/sciadv.abm3622.
- P. J. Podrid, F. J. Venditti and P. A. Levine,
et al., The role of exercise testing in evaluation of arrhythmias, Am. J. Cardiol., 1988, 62(12), 24H–33H, DOI:10.1016/0002-9149(88)90337-2.
- B. X. Xu, A. Akhtar and Y. H. Liu,
et al., An Epidermal Stimulation and Sensing Platform for Sensorimotor Prosthetic Control, Management of Lower Back Exertion, and Electrical Muscle Activation, Adv. Mater., 2016, 28, 4462–4471, DOI:10.1002/adma.201504155.
- N. A. Maffiuletti, M. A. Minetto and D. Farina,
et al., Electrical stimulation for neuromuscular testing and training: state-of-the art and unresolved issues, Eur. J. Appl. Physiol., 2011, 111, 2391–2397, DOI:10.1007/s00421-011-2133-7.
- D.-K. Kwon and J.-M. Myoung, Wearable and Semitransparent Pressure-Sensitive Light-Emitting Sensor Based on Electrochemiluminescence, ACS Nano, 2020, 14(7), 8716–8723, DOI:10.1021/acsnano.0c03186.
- M. Bariya, Z. Shahpar and H. Park,
et al., Roll-to-Roll Gravure Printed Electrochemical Sensors for Wearable and Medical Devices, ACS Nano, 2018, 12(7), 6978–6987, DOI:10.1021/acsnano.8b02505.
- Y. Gao, H. Ota and E. W. Schaler,
et al., Wearable microfluidic diaphragm pressure sensor for health and tactile touch monitoring, Adv. Mater., 2017, 29(39), 1701985, DOI:10.1002/adma.201701985.
- S. Mishra, J. J. S. Norton and Y. Lee,
et al., Soft, conformal bioelectronics for a wireless human-wheelchair interface, Biosens. Bioelectron., 2017, 91, 796–803, DOI:10.1016/j.bios.2017.01.044.
- D. R. Seshadri, R. T. Li and J. E. Voos,
et al., Wearable sensors for monitoring the physiological and biochemical profile of the athlete, NPJ Digit. Med., 2019, 2(1), 72, DOI:10.1038/s41746-019-0150-9.
- S. Yao, P. Swetha and Y. Zhu,
et al., Nanomaterial-Enabled Wearable Sensors for Healthcare, Adv. Healthcare Mater., 2018, 7(1), 1700889, DOI:10.1002/adhm.201700889.
- H. Q. Zhang, R. Y. He and Y. Niu,
et al., Graphene-enabled wearable sensors for healthcare monitoring, Biosens. Bioelectron., 2021, 197, 113777, DOI:10.1016/j.bios.2021.113777.
Footnote |
† These authors contributed equally to this work. |
|
This journal is © The Royal Society of Chemistry 2022 |
Click here to see how this site uses Cookies. View our privacy policy here.