DOI:
10.1039/D2RA04591E
(Paper)
RSC Adv., 2022,
12, 23704-23717
A comparative study of the mechanical stability, electronic, optical and photocatalytic properties of CsPbX3 (X = Cl, Br, I) by DFT calculations for optoelectronic applications
Received
23rd July 2022
, Accepted 5th August 2022
First published on 19th August 2022
Abstract
Organic free Cs-based perovskite materials are potential candidates for electronic and optoelectronic applications. A systematic comparative study of the mechanical, electronic, optical, and photocatalytic properties of CsPbX3 (X = Cl, Br, I) was conducted using density functional theory to compare the applicability of these materials in optoelectronic, photocatalytic, and photovoltaic (PV) devices. We calculated structural and elastic properties to determine the better agreement of damage-tolerance and electronic and optical responses for suitable device applications. Optimized lattice parameters and elastic constants showed excellent agreement with the experimental data whereas some properties were found to be much better than other theoretical reports. CsPbBr3 is thermodynamically more stable and more ductile compared to the other two perovskites. The hydrostatic pressure dependent mechanical stability showed that CsPbCl3 and CsPbBr3 sustained stability under low applied pressure, whereas the stability of CsPbI3 was very high. The electronic band gap calculations showed that CsPbCl3, CsPbBr3, and CsPbI3 are suitable for green, orange, and red emissions of optical spectra owing to the proper electronic band gaps. CsPbI3 can be shown as the best photocatalyst for the hydrogen evolution reaction and CsPbBr3 is the most stable photocatalyst due to its nearly balanced oxidation and reduction potentials, but CaPbCl3 is better for O2 production. The density of states and other optical properties have been reported in this study. Thus, our findings would be beneficial for experimental studies and can open a new window for efficient electronic, optoelectronic, and hydrogen production along with the biodegradation of polluted and waste materials.
1. Introduction
The demand for more efficient PV cells, optoelectronic and electronic devices is of great concern with the increasing world population under the worse conditions of insufficient energy crisis.1 With this increasing demand, researchers are extremely careful on a healthy ecosystem. A new kind of effective, eco-friendly, and renewable energy capturing technology has achieved much more interest among the world scientific community.2–11 The present photovoltaic market is dominated by Si-based solar cells12,13 but they do not work for long due to low efficiency and maintenance of the expensive purification processes.1 Besides, their less optical absorption, less carrier mobility, and indirect band type1 have made this rethinkable in the competitive race for the production of more functioning device, and the amorphous Si thin film technology is cost-effective and the fabrication process is simple. However, its low stability as well as lower photo conversion efficiency (PCE) are of great concern.14,15 Thus, researchers are eagerly concerned to find alternative low-cost, earth-abundant, and solution-processable solar cell materials. To this end, organic–inorganic halide perovskites meet these demands and are of great interest. In the 1990s, perovskite materials started their research journey extensively for the replacement of Si and multifunctional solar cell devices.
The perovskite solar cell was first reported by Miyasaka and co-workers in 2006 and they reported a PCE of ∼2.2%16,17 that reached 3.8% in 2009.18 In 2018, a research report on a PCE of 27.6% was published, which mentioned that a 30% plus PCE was possible by the end of 2020, but in 2020, the US National Renewable Energy Laboratory certified a perovskite solar cell with a max. PCE of 25.5%.19 Researchers found hope for mixed halide perovskites from a comparative study of different structures. Thus organic–inorganic lead halide perovskites solar cells have drawn incredible attention in the scientific community as alternative competitors to some inorganic photovoltaic materials, such as copper indium gallium sulfide and selenide (CIGS), cadmium telluride (CdTe), amorphous or single crystal silicon (a-Si or c-Si), iron disulphide (FeS2), and gallium arsenide (GaAs). Though multi-junction AlGaInP, AlGaAs, GaAs, GaInAs cells are certified as 47.1% efficient in the present research,20 all of these compounds are expensive due to the presence of scarcity elements, In, and Ga, and they are also toxic as they contain As and P. Hence, metal halide perovskites21–31 solar cells have a great possibility to replace the dominating Si-based solar cells by proper selection of atoms in a right place. Organic–inorganic halide perovskites with a formula of AMX3 (where, organic–inorganic cation A = CH3NH3+ or Cs+, etc.; inorganic cation M = Pb2+ or Sn2+, etc.; halide X = Cl−, Br−, I−)1,32 are highly33–36 efficient due to excellent absorption coefficient,37–39 carrier mobility, lengthy carrier life, and a suitable band gap.40–45 Due to low physical and chemical stabilities of organic–inorganic perovskites,1 inorganic perovskites have achieved tremendous research interest. Among them, Cs-based inorganic perovskites CsPbX3 (X = Cl, Br, I) are promising materials for next-generation advanced electronic, optoelectronic and photonic devices, and they possess temperature-dependent phases.46 The properties of CsPbX3 can be tuned by metal or mixing halides to enhance optoelectronic device applications such as LEDs, photo-detectors, and lasers.52–76 Among CsPbX3 (X = Cl, Br, I), CsPbCl3 has a wider band gap and better thermal stability due to the value of binding energy of 64 meV,47,48 which is preferable for the optomagnetic and optoelectronic,49 single,50 and multiphoton pumping-based laser devices.51. The cubic bulk CsPbBr3 has also temperature responses over the phase transition.77–79 Halide exchange and mixing can ensure more suitable properties tuning of CsPbBr3 for quantum dots due to high carrier transportability, reliable optoelectronic properties, and the enormous photoluminescence quantum yield, and greater constancy under humidity and thermal outbreaks.80,81 CsPbBr3 demonstrated a band gap of 2.30 eV,82 and cubic bulk CsPbI3 has a band gap of 1.73 eV.83 It is a potential candidate for room temperature Si-based thin film perovskite heterojunction solar cells.83,84 CsPbI3 is a highly efficient thermally stable solar cell due to its relatively high light absorption coefficient, and carrier mobility in the visible region.85 Organic–inorganic perovskites have a great tendency to dissolve under humidity at high temperature86 and are greatly sensitive to light and heat49 as well as the presence of toxic lead in these perovskites, which causes huge health issues to live creatures. It can be seen that CsPbX3 (X = Cl, Br, I) are direct band gap semiconductors that absorb visible and ultraviolet light. Hence, these compounds are used in photovoltaic and optoelectronic devices. It should be remembered that pressure plays an important role in tuning the physical properties of any materials.87–89 It treats phase transitions and is often reversible if the external conditions are the same in the complete cycle.90,91 In this sense the high-pressure studies often offer fundamental insight into the optoelectronic properties of perovskite materials.92–94 Perovskites are an advanced class of materials that have exhibited great potential in optoelectronic technologies thus all-inorganic perovskites have an extremely versatile crystal structure. Thus far, there are no such theoretical reports on the pressure-dependent comparative studies of the elastic, mechanical, electronic, optical, and photocatalytic properties of CsPbX3.
In this work, for the first time, a pressure-dependent comparative study of the mechanical, elastic properties and electronic, optical, and photocatalytic properties has been performed through first-principles calculations based on the density functional theory (DFT). In this study, we tried to find out more stable and efficient materials among them to make a prediction for the proper selection of perovskite materials for electronic, photocatalytic, and optoelectronic applications.
2. Methodology
The bulk perovskites of CsPbX3 (X = Cl, Br, I) exhibit cubic structure of space group Pmm (221), and the lattice constants are 5.734, 6.017, and 6.38, respectively (Table 1). To compare and analyze the data of the structural, mechanical, electronic, optical and photocatalytic properties, DFT calculations were performed using the CASTEP code in Materials Studio simulation software.95–100 Valance shell and ion core electrostatic interactions of ingredient atoms were investigated under Generalized Gradient Approximation (GGA) of Perdew–Burke–Ernzerhof (PBE) with norm-conserving pseudo-potentials treatment but the mechanical properties were calculated using ultrasoft pseudopotentials for the better agreement with experimental data. The photocatalytic properties were also calculated from the electronic band structure calculations. Initially, we chose the cubic structure to carry out the numerical operations.
Table 1 Calculated optimized lattice parameters, volumes and band gap values of CsPbCl3, CsPbBr3 and CsPbI3a
Materials |
Method |
Lattice parameters |
Band gap, Eg (eV) |
Bulk type |
a (Å) |
V (Å3) |
Refs: a = [102], b = [103], c = [104], d = [105], e = [32], f = [82], g = [106], h = [107], i = [108], j = [109], k = [110], l = [83], m = [119], n = [120], o = [121], p = [122], q = [123]. |
CsPbCl3 |
Calc. |
5.785 |
193 |
2.385 |
Cubic |
Theo. |
5.62a,5.743b |
2.168a, 2.202b |
|
5.7c, 5.7285d |
2.4c, 2.27d, 2.72i, 3.03o |
|
Expt. |
5.605q |
2.98m |
|
3.00p |
|
CsPbBr3 |
Calc. |
6.011 |
217 |
2.061 |
Cubic |
Theo. |
6.013b |
1.61a, 2.30f, 1.796b, 2.30e |
5.98h |
1.74g |
6.001g |
1.86h, 2.30o |
Expt. |
5.87a, 5.85e |
2.28i, 2.36m |
2.33p |
CsPbI3 |
Calc. |
6.432 |
266, 260j, 249k |
1.784 |
Cubic |
Theo. |
6.405b, 6.38j |
1.478a, 1.479b, 1.44j |
6.24a |
1.73l |
Expt. |
6.29k |
1.77m |
1.73n |
A primitive cell of CsPbCl3 consisting 5 atoms was formed with 1 Cs atom that occupied the Wyckoff site (0, 0, 0), 3 Pb atoms at the site of (0.5, 0.5, 0.5) and 1 Cl atom at (0, 0.5, 0.5) fractional coordinates101 as depicted in Fig. 1. The sets of valence shall are interacted as Cs: 5s2 5p6 6s1, Pb: 5s2 5p6 5d10 6s2 6p2, Cl: 3s2 3p5, Br: 4s2 4p5 and I: 5s2 5p5. All the crystals were optimized using the same software functional treatments. CsPbCl3 was simulated first by providing a lattice parameter as a = 5.734 (ref. 101) and the optimized structure with minimum formation enthalpy was confirmed with plane wave basic set k-points of 6 × 6 × 6 and cut-off energy of 520 eV with SCF tolerance 2 × 10−6 eV per atom, convergence tolerance energy of 2 × 10−5 eV per atom as well as maximum force and stress 0.05 eV Å−1and 0.10 GPa, respectively. The maximum atomic displacement was restricted up to 0.002 Å. We calculated the elastic, electronic, and optical properties for CsPbBr3 and CsPbI3 after replacing the Cl atom (100%) with Br and I atoms under the same conditions.
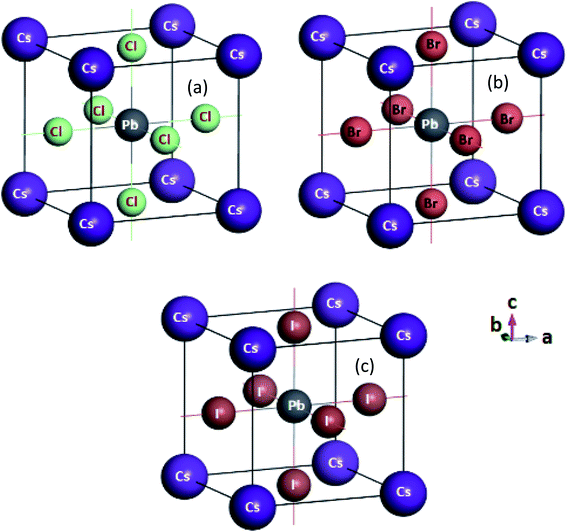 |
| Fig. 1 Cubic structure of (a) CsPbCl3 (b) CsPbBr3 and (c) CsPbI3 are depicted with atomic orientations. | |
3. Results and discussion
3.1 Structural properties
The three phases (cubic, tetragonal and orthorhombic) of CsPbCl3 were primarily chosen as the highest temperature phases of the cubic structure to carry out the numerical operations for high-temperature device applications. The optimized-cell structure of CsPbCl3 was determined and then the Cl atom was replaced by Br and I atoms, which were then optimized for CsPbBr3 and CsPbI3 perovskite structures. Fig. 1(a)–(c) represent the optimized crystal structures of CsPbCl3, CsPbBr3 and CsPbI3. The optimized cell parameters for the corresponding perovskites are represented in Table 1. From Table 1, it can be seen that after several k-points tests the optimized lattice parameter a = b = c of CsPbCl3 was 5.785 Å, which was elongated about 3.9% and 11.2% after the replacement of halide Cl atom by Br and I atoms and the obtained values were in a great agreement with other theoretical and experimental values.32,82,83,102–110,123 From the cell parameters and volumes of CsPbX3 (X= Cl, Br, I) results, a relation can be expressed as CsPbCl3 < CsPbBr3 < CsPbI3 (Table 1) with volume increments of 12.4% and 37.8% for CsPbBr3 and CsPbI3, respectively.
3.2 Mechanical properties
The mechanical properties of the cubic crystals of CsPbX3 were explicitly and implicitly calculated using three independent elastic tensors C11, C12 and C44. The calculated values are represented in Table 2 and shown graphically in Fig. 2(a) for the isostructural of CsPbCl3, CsPbBr3, and CsPbI3. Mechanical stability was justified under Born criteria for these crystals111 and it is clearly seen that all are mechanically stable because they satisfy the condition C11 + 2C12 > 0, C44 > 0 and C11 − C44 > 0 as well as another stability condition of C12 < B < C11.106 All of these in the bulk form are elastically anisotropic, confirmed by the relation 2C44 = C11 − C12 (ref. 112) and reconfirmed by non-zero Zener's anisotropy factor A.113,114 The C11 > C12 > C44 conditions show that the materials resist longitudinal deformation of more than shape deformation, where the largest value of C11 for CsPbBr3 offers more resistance to longitudinal deformation. Young's modulus (E) is an indication of the degree of resistance offered by a material to longitudinal tension.106 A comparative data set of E's (Table 2) could be an inspiration for conducting further research on CsPbBr3 for band gap tailoring and other purposes due to the comparatively high value of E. Pressure responses of CsPbX3 are depicted in Fig. 2(b) with B, G, E data. A comparative study of Cauchy pressure C12 − C44 (ref. 115) of the tabulated data shown in Table 1 confirms the brittle-like nature of CsPbCl3 > CsPbBr3 > CsPbI3, which can be reconfirmed from the values of Pugh's ratio B/G < 1.75 for all structures,116 which is shown in Fig. 2(c). It offers a completely new result from other researchers' findings,117,118 which may open a new opportunity to observe the material insight. CsPbBr3 offers a larger value of bulk modulus, whereas CsPbCl3 offers the least value, thus the compressibility of these perovskites can be ordered as CsPbBr3 < CsPbI3 < CsPbCl3.
Table 2 Calculated pressure independent (at 0 GPa) elastic constants Cij (GPa), elastic moduli B, G, E (GPa), Pugh's ratio B/G, Poisson's ratio σ, and machinability index μM = B/C44 and elastic anisotropy factor A, melting temperature Tm for CsPbCl3, CsPbBr3 and CsPbI3a
Materials |
C11 |
C12 |
C44 |
B |
G |
E |
B/G |
σ |
μM |
A |
Tm |
Ref: n = [115]. |
CsPbCl3 |
48.353, 52.941n |
7.881, 11.700n |
4.921, 4.800n |
21.372, 25.447n |
9.052, 9.027n |
23.799 |
2.361, 1.234n |
0.314 |
4.342 |
0.243 |
838.7 |
CsPbBr3 |
51.946 |
16.479 |
4.189 |
28.302 |
7.819 |
21.479 |
3.619 |
0.374 |
6.756 |
0.236 |
860.0 |
CsPbI3 |
39.323 |
6.754 |
5.352 |
17.61 |
8.522 |
22.015 |
1.811 |
0.292 |
3.289 |
0.328 |
785.4 |
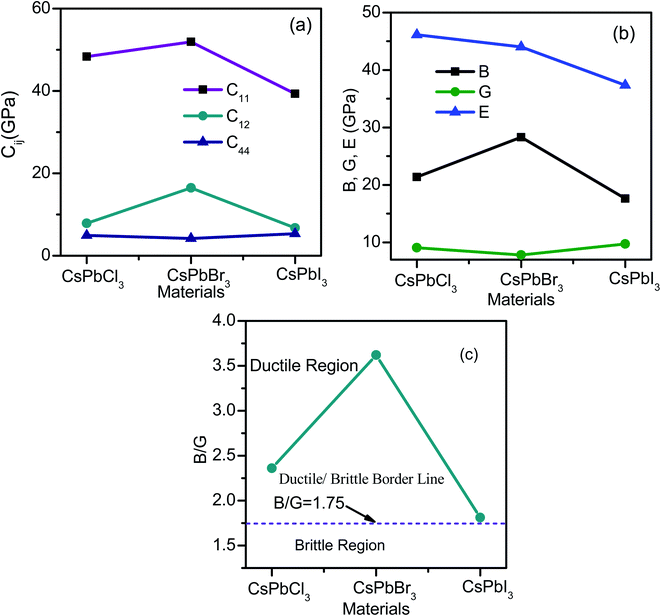 |
| Fig. 2 Elastic tensors Cij, modulus of elasticity B, G, and E are plotted in (a) and (b) respectively. Pugh's ratio is shown in (c) for CsPbX3. | |
The elastic and universal anisotropy factors A and AU were calculated using the following relations115,116 as
The values of Zener's anisotropy factor, A, for all structures were less than unity. Any deviation from unity confirms the elastic anisotropic property.117 For these materials, the order of anisotropy can be obtained as CsPbBr3 > CsPbI3 > CsPbCl3 (Table 2). The melting point temperatures (Tm) were calculated using the following empirical formula proposed by Fine et al.118
From Table 2, it is obvious that CsPbBr3 possesses the highest melting temperature due to the highest C11 and the comparison suggests that CsPbBr3 is a better choice for high-temperature efficiency and potential applications in comparison to the other two. The value of the machinability index indicates the suitability of the materials for industrial applications, whereas CsPbBr3 shows dominating nature over CsPbCl3 and CsPbI3 (Table 2) though its Hv value is the lowest one.
The calculated elastic constants are tabulated in Table 3. The values of the calculated elastic constants are positive and satisfy the Born stability criteria, which implies that the compounds are mechanically stable. The calculated elastic constants for CsPbCl3 are in good agreement with the available theoretical results reported in the literature.115 It should be noted that the mechanical instability is responsible for the negative values of C44 and B/C44, whereas stability can be satisfied by the value of C44 > 0. From Table 3 and Fig. 3(a), we observed that CsPbCl3 and CsPbBr3 lose mechanical stability at an applied pressure above 20 GPa and 25 Gpa, respectively, while CsPbI3 can tolerate more pressure and shows stability up to the hydrostatic pressure of about 75 GPa. The mechanical instability was confirmed from the negative value of C44 corresponding to the threshold pressures for individual perovskites. From the above discussion and the obtained results, the stability order of the three compounds under applied pressure can be followed as CsPbI3 > CsPbBr3 > CsPbCl3, but CsPbI3 remains stable under hydrostatic pressure approximately three times more than that of CsPbCl3 (Table 3 and Fig. 3(c)). The Poisson's ratio of the system was calculated using the following formula.
Table 3 Calculated pressure-dependent elastic constants Cij (GPa), elastic moduli B, G, E (GPa), Poisson's ratio σ, and machinability index μM = B/C44 and Pugh's ratio B/G for CsPbCl3, CsPbBr3 and CsPbI3
Materials |
P |
a (Å) |
V (Å3) |
C11 |
C12 |
C44 |
B |
G |
E |
σ |
B/C44 |
B/G |
CsPbCl3 |
0 |
5.734 |
188.526 |
48.353 |
7.881 |
4.921 |
21.372 |
9.052 |
23.796 |
0.314 |
4.343 |
2.361 |
5 |
5.446 |
161.53 |
97.069 |
16.589 |
4.065 |
43.416 |
12.44 |
34.068 |
0.369 |
10.68 |
3.489 |
10 |
5.271 |
146.485 |
145.49 |
29.864 |
2.916 |
68.406 |
14.79 |
41.382 |
0.399 |
23.458 |
4.625 |
15 |
5.151 |
136.698 |
175.01 |
27.907 |
1.684 |
76.941 |
16.6 |
46.453 |
0.399 |
45.689 |
4.635 |
20 |
5.052 |
128.959 |
214.4 |
36.143 |
0.483 |
95.561 |
18.37 |
51.796 |
0.409 |
197.85 |
5.201 |
25 |
4.972 |
122.947 |
249.95 |
43.191 |
−0.716 |
112.11 |
19.86 |
56.26 |
0.416 |
−156.58 |
5.644 |
30 |
4.903 |
117.891 |
281.84 |
47.262 |
−2.008 |
125.46 |
21.16 |
60.103 |
0.42 |
−62.477 |
5.928 |
CsPbBr3 |
0 |
6.014 |
217.613 |
51.946 |
16.479 |
4.189 |
28.301 |
7.819 |
21.478 |
0.373 |
6.756 |
3.619 |
5 |
5.663 |
181.623 |
90.465 |
13.995 |
3.612 |
39.485 |
11.56 |
31.601 |
0.366 |
10.931 |
3.415 |
10 |
5.473 |
163.947 |
125.74 |
14.119 |
2.887 |
51.327 |
14.35 |
39.39 |
0.372 |
17.778 |
3.575 |
15 |
5.339 |
152.189 |
173.83 |
28.697 |
2.141 |
77.074 |
16.91 |
47.262 |
0.397 |
35.999 |
4.558 |
20 |
5.235 |
143.502 |
211.2 |
35.276 |
1.285 |
93.918 |
19.04 |
53.501 |
0.405 |
73.087 |
4.932 |
25 |
5.151 |
136.732 |
248.17 |
43.089 |
0.334 |
111.45 |
20.89 |
58.971 |
0.411 |
333.68 |
5.336 |
30 |
5.079 |
131.056 |
283.31 |
49.763 |
−0.661 |
127.61 |
22.6 |
64.025 |
0.416 |
−193.06 |
5.646 |
CsPbI3 |
0 |
6.403 |
262.567 |
39.323 |
6.754 |
5.352 |
17.61 |
8.521 |
22.012 |
0.291 |
3.29 |
2.066 |
5 |
5.985 |
214.423 |
81.669 |
12.258 |
4.671 |
35.395 |
11.91 |
32.136 |
0.348 |
7.577 |
2.97 |
10 |
5.761 |
191.205 |
119.43 |
15.974 |
1.343 |
50.461 |
11.85 |
32.966 |
0.391 |
37.573 |
4.258 |
15 |
5.608 |
176.452 |
170.4 |
27.372 |
6.436 |
75.048 |
21.29 |
58.362 |
0.37 |
11.66 |
3.524 |
20 |
5.498 |
166.228 |
207.43 |
35.467 |
5.792 |
92.787 |
23.55 |
65.146 |
0.382 |
16.019 |
3.939 |
25 |
5.405 |
157.935 |
238.25 |
38.761 |
3.174 |
105.26 |
23.49 |
65.593 |
0.396 |
33.162 |
4.48 |
30 |
5.323 |
150.823 |
288.88 |
50.853 |
7.061 |
130.2 |
31.58 |
87.658 |
0.387 |
18.438 |
4.122 |
35 |
5.258 |
145.437 |
323.53 |
58.817 |
6.712 |
147.06 |
33.9 |
94.429 |
0.392 |
21.909 |
4.338 |
40 |
5.202 |
140.813 |
357.61 |
66.848 |
6.365 |
163.77 |
36.14 |
100.99 |
0.397 |
25.729 |
4.531 |
45 |
5.152 |
136.783 |
388.58 |
72.781 |
5.468 |
178.05 |
37.67 |
105.58 |
0.401 |
32.561 |
4.725 |
50 |
5.105 |
133.092 |
419.99 |
79.415 |
3.509 |
192.94 |
38 |
106.96 |
0.407 |
54.984 |
5.078 |
55 |
5.063 |
129.827 |
449.5 |
84.787 |
1.322 |
206.36 |
37.96 |
107.31 |
0.413 |
156.1 |
5.435 |
60 |
5.024 |
126.86 |
483.76 |
93.126 |
0.972 |
223.34 |
40.16 |
113.67 |
0.415 |
229.77 |
5.56 |
65 |
4.988 |
124.135 |
523.28 |
103.91 |
3.342 |
243.7 |
45.7 |
129.02 |
0.411 |
72.92 |
5.333 |
70 |
4.956 |
121.731 |
560.19 |
114.31 |
4.659 |
262.93 |
49.82 |
140.57 |
0.41 |
56.435 |
5.278 |
75 |
4.925 |
119.505 |
588.96 |
119.87 |
2.623 |
276.23 |
49.87 |
141.1 |
0.414 |
105.31 |
5.539 |
80 |
4.896 |
117.42 |
615 |
124.42 |
−0.964 |
287.95 |
47.96 |
136.32 |
0.421 |
−298.7 |
6.003 |
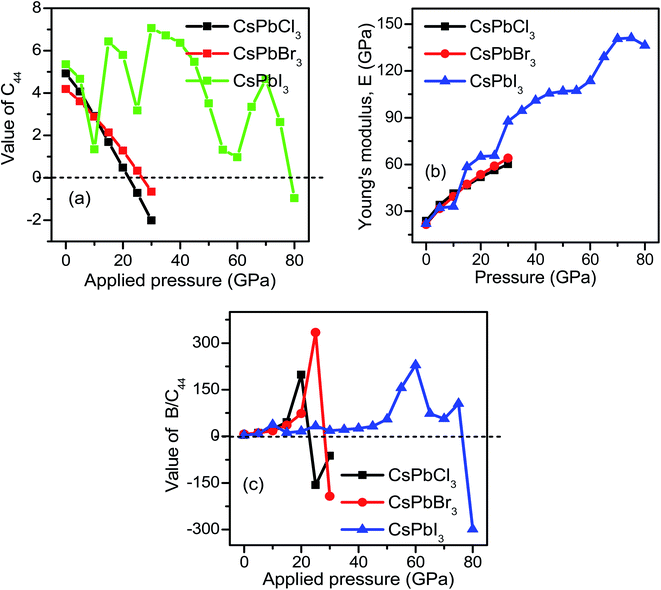 |
| Fig. 3 Pressure-dependent (a) elastic tensor C44, (b) Young's modulus (E), and (c) machinability index (μM = B/C44) are plotted for CsPbX3. | |
The calculated values are also included in Table 3. It is obvious that with the increase in hydrostatic pressure, the value of the Poisson's ratio σ increases for CsPbX3. It is well known that σ can separate crystalline solids as brittle and ductile at the borderline value of 0.26. From Table 3, it can be clearly evident that all the structures possessed values greater than 0.26, indicating that they must be quite ductile in nature. Among them, CsPbI3 is more ductile as it has more pressure tolerance. Bulk to shear modulus ratio (B/G) with the critical value of ductility of 1.75 served as the borderline between the ductile and brittle behaviors of crystalline solids. It also predicted a more ductile behavior of CsPbI3.
Young's modulus, E measures the stiffness against longitudinal deformation as well as thermal shock resistance of the materials. A larger value of E indicates a smaller thermal resistance. The thermal shock resistance order under different pressure conditions followed the order CsPbCl3 > CsPbBr3 > CsPbI3 (Fig. 3(b)).
3.3 Electronic properties
The energy band diagram with k-points in Brillouin zone sampling represents the energy band gap of a material. Fig. 4(a)–(c) represents the electronic band structures of CsPbX3. The energy differences between the top of the valence band (VB) and the bottom of the conduction band (CB) along the same point of momentum space (at the R point) for all structures provides evidence for the existence of a direct band gap. Electronic transition in the direct band gap material offers less momentum loss for carrier propagation as the phonon contributions are negligible for pair creation. From Fig. 4, it is clearly seen that the valence shells are extended from −5 eV up to 0 eV, where 0 eV energy levels (green lines) represent the Fermi levels (EF). The electronic shells above the EF form conduction bands, which have been extended from 0 eV up to 5 eV. From Fig. 4(a)–(c), it is seen that CsPbCl3, CsPbBr3 and CsPbI3 have direct band gaps with values of 2.385 eV, 2.061 eV and 1.784 eV, respectively, where CsPbCl3, CsPbBr3 and CsPbI3 show the carrier transitions for green, orange and red emissions of visible spectra, respectively. Solar irradiance on earth's surface is of maximum photon flux density for green wavelength and appreciably high enough for orange as well as red spectra of the visible region.105
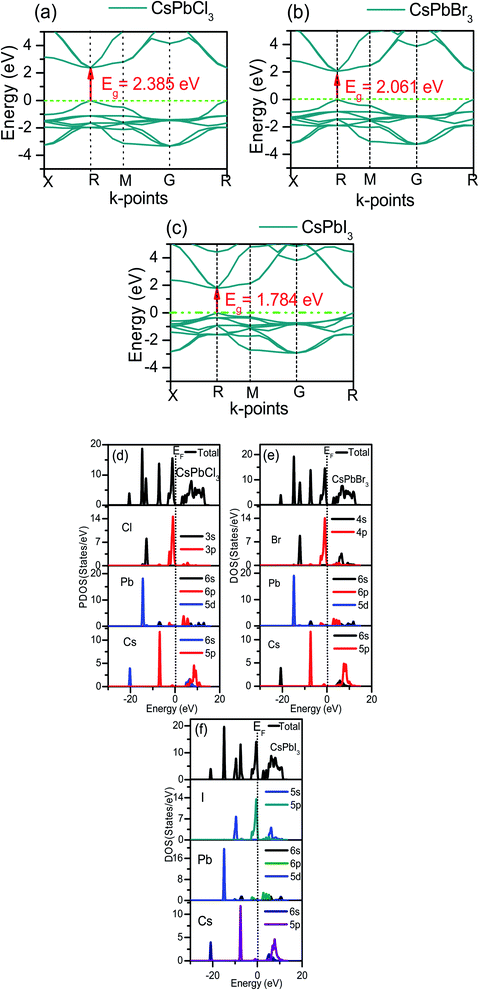 |
| Fig. 4 Energy band diagram with k-points for (a) CsPbCl3, (b) CsPbBr3 and (c) CsPbI3, and density of states (DOS) with corresponding energy of the ingredient atoms of (d) CsPbCl3, (e) CsPbBr3 and (f) CsPbI3 calculated in GGA-PBE treatments. | |
The obtained values of the band gap are listed in Table 1. By comparing the band gaps shown in Table 1, it is evident that the value of CsPbCl3 is closest to the theoretical reported values of 2.168 eV,102 2.202 eV,103 2.40 eV,104 and 2.27 eV (ref. 102) but it is (2.385 eV) smaller than the theoretical value of 3.03 eV (ref. 121) and experimental value of 3.0 eV.119,122
From Table 1 it is clearly seen that the calculated band gap of CsPbBr3 is 2.061 eV, whereas the band gaps of CsPbBr3 are reported as 1.796 eV,103 1.74 eV,106 2.30 eV,32,82,121 2.36 eV,119 2.33eV,122 1.86 eV (ref. 107) and 2.28 eV.108 Thus, our findings are in good agreement and very close to the other theoretical and experimental findings.32,82,103,106–108,121,122 Importantly, we were able to tune the band gap of CsPbBr3 to 2.061 eV, which is excellent suitable for optoelectronic and PV cell applications, whereas, our calculated Eg value for CsPbI3 was obtained to be 1.784 eV, which is in excellent agreement with the experimental value of 1.77 eV (ref. 119) and theoretical value of 1.73 eV (ref. 83 and 120) as well as more reliable than others theoretical observation of ∼1.478 eV,102 1.479 eV,103 1.44 eV (ref. 109) but very close to the experimental value (Table 1).83,102,103,109 It should be noted that the theoretical band gap obtained using PBE–GGA is a little bit underestimated compared to the experimental value, which could be due to the error in GGA.119–123 It can be seen that when Cl is partially replaced by Br and I atoms, the band gap decreases because of an increase in the atomic size. In all cases, the origin of some discrepancies with experimental values may arise from the drawbacks of the calculative process of the first principal calculations. From Fig. 4(a)–(c), it is explicitly clear that the top of the VB is well dispersive than the bottom of the CB, which indicates that the photo-generated holes possess lower effective mass. A lower carrier effective mass corresponds to higher carrier mobility. As such, the photo-generated holes in the VB can transfer more rapidly to the surface of CsPbX3 to participate in conduction and in photocatalytic reactions. This nature of the charge carriers for CsPbX3 systems supports the p-type conduction of CsPbCl3, CsPbBr3, and CsPbI3. The curvature of the valence band maxima shows a decrement with the replacement of Cl by Br and I. The hole mobility decreases with the insertion of Br and I in the place of Cl in CsPbCl3.
Atomic contributions to the band structure formation are shown in terms of density of states (DOS) for individual atoms and the total densities of states (TDOS) for all atoms are shown in Fig. 3(d–f) for CsPbX3 perovskites. From Fig. 4(d), it is evident that the DOS of CsPbCl3 can be divided into six major regions. The first region (Fig. 3(d)) −21.14 eV to −19.54 eV is mainly arising due to the Cs-6s orbital state, the second region −15.08 eV to −13.54 eV is due to the Pb-5d state, the third region −13.38 eV to −12.23 eV is due to Cl-3s, the fourth region −7.33 eV to −5.82 eV is due to the hybridization of Cs-5p and Pb-5d states and the fifth region lies between −3.16 eV to 0 eV below the EF level, which is called the top of the valence band and is originated from the 3p orbital of Cl atom. Whereas the conduction band that lies between 2.38 eV and13.24 eV, above the EF level, originated from the hybridization of the 6p orbital of Cs and 6p orbital of Pb and 3p orbital of Cl atoms. In a similar way, for CsPbBr3 the core VB originated from the mixing of Cs-3p, Pb-5d, Br-3s, Cs-5p, and Pb-5d and the top of the valence band originated from the 4p state of Br atom and the CB band arise from the hybridization of the 6p orbital of Cs and the 6p orbital of Pb and the 4p orbital of Br atoms (Fig. 4(e)). For the CsPbI3 system, the core VB originated from Cs-3p, Pb-5d, I-3s, Cs-5p, and Pb-5d and the top of the valence band originated from the 5p state of the I atom and CB occurred from the predominant hybridization of Cs-6p, Pb-6p and I-5p orbitals of Cs, Pb, I atoms (Fig. 4(f)). From the atomic contribution plots, it is clear that Pb and halogen atoms have a major contribution to the valence and conduction bands, which reduces the band gap energy. The s and p states of Pb and the p state of the halogens play a major role in the reduction of band gap energy as shown in Fig. 4(d)–(f). The order of decrement can be assigned as CsPbCl3 > CsPbBr3 > CsPbI3 (Fig. 5).
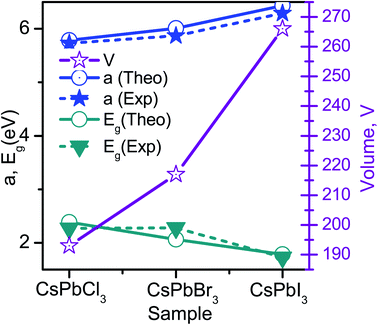 |
| Fig. 5 Comparative presentation of theoretical and experimental values of lattice parameters and band gap values for CsPbX3 (X = Cl, Br, I). | |
3.4 Optical properties
Many application fields, such as absorbers, reflectors, optical coatings, and different optoelectronic devices of materials are examined from their optical responses. In the presence of an external electromagnetic wave, the photonic interactions of the material can provide information on the properties and application predictions as a function of energy. These properties are analyzed for electronic transitions between occupied and empty states, band structures, bond types as well as internal structures of the materials according to their optical spectra. The frequency-dependent complex dielectric function is followed by the Kramers–Kronig transformation as ε(ω) = ε1(ω) + iε2(ω), which is also related to the representation of relative permittivity. The imaginary part of this complex function is,
where ω = 2πv is the frequency of the incident photon and e, û,
are the electronic charge, polarization vector of the incident field, and radius vector, respectively. Whereas, Ψkc and Ψkv are the wave functions of the conduction and valence bands at K, respectively. Using ε1 and ε2, other optical properties such as absorption coefficient α(ω), optical conductivity σ(ω), loss function L(ω), reflectivity R(ω) and refractive index n(ω) can be calculated118 using the following expressions.
The dielectric function is a fundamental parameter that is related to the charge-carrier regeneration rate of certain materials used in the solar cell.124,125 It gives a clear idea of the performance of optoelectronic devices.126 Higher dielectric constant values of the perovskite solar cells can lead to lower recombination rates. Fig. 6(a) shows the imaginary parts of the dielectric function of CsPbX3 perovskites. The real part of the dielectric function shows a higher value at low photon energy and then decreases rapidly with the increase of photon energy. It is well known that a perovskite material with such a higher value of the real part of the dielectric function exhibits a lower band gap,127 which is also seen in the electronic band structure. On the other hand, the values of the imaginary part of the dielectric function decrease to zero at a higher photon energy region. It should be noted that there are few peaks arising at 3.9, 7.8, 11.0 and 14.1 eV for CsPbCl3; 3.5, 7.3, 10.3 and 13.9 eV for CsPbBr3; and 3.0, 6.3, 8.5 and 13.9 eV for CsPbI3. The first peak is due to the generation of the electron–hole pairs for conduction. The 2nd and 3rd peaks are due to the interband optical transitions from the valence bands to the conduction bands. The fourth peak around 14.0 eV is due to the collecting excitons from the free carriers, which is the so-called plasma frequency and the peak is called the plasmon peak. These irregular photo responses of the materials cause the difference in band gaps among them.
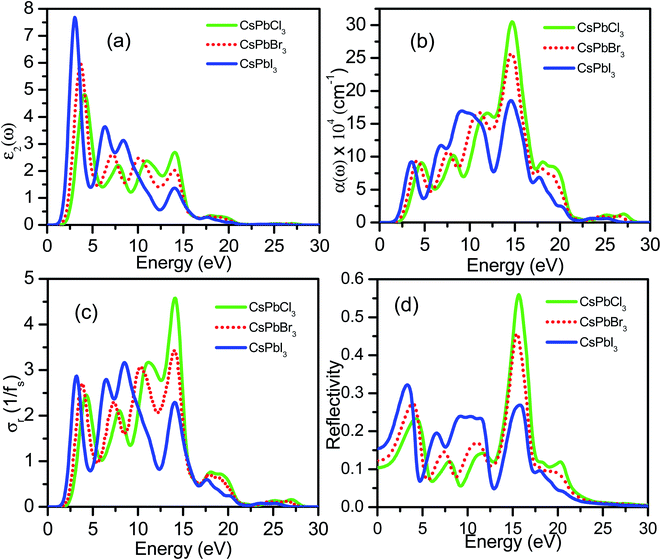 |
| Fig. 6 Optical responses in terms of dielectric functions (a), (b) absorption coefficients, (c) conductivity and (d) reflectivity of CsPbCl3, CsPbBr3 and CsPbI3 are shown with green, dotted red and blue lines, respectively. | |
It is well known that the performance of solar cells is determined by various optical properties, among them the absorption coefficient (α) is the crucial property. The variation of optical absorption of CsPbX3 perovskites can be seen in Fig. 6(b). The value of α of the I-based perovskite demonstrates its maximum intensity in the ultraviolet region compared to that of Br and Cl-based perovskites. The first peak of α offers an optical transition of electrons from Cl-3p of the valence band to Pb-6p of the conduction band at higher energy compared to that of the interband transitions of electrons in the case of CsPbBr3 and CsPbI3. The optical band gaps can be seen from the absorption peaks of corresponding materials as Eg (CsPbCl3)> Eg (CsPbBr3) > Eg (CsPbI3), which is coherent with the calculated values of Eg of CsPbX3 perovskites (Table 1). The highest absorption peaks for all materials occurred at the same energy of 14.6 eV (Fig. 6(b)).
Fig. 6(c) shows the real part of photoconductivity. From the figure, it is evident that if the absorption of photons enhances, the rate of photoconductivity tends to increase. Thereby, greater absorption and more photoconductivity are observed in the I-based perovskite compound relative to the Br and Cl-based perovskite compounds. The photoconductivity comparison of these materials shows some peaks over a wide range of energy spectra. The conductivity initiatives and the first peaks are ordered in terms of the energy as σ (CsPbI3) > σ (CsPbBr3) > σ (CsPbCl3). Photoconductivity increases at the energy edges 4.2, 7.8, 11.3 and 14.1 eV for CsPbCl3, 3.8, 7.3, 10.2 and 14.1 eV for CsPbBr3 and 3.0, 6.4, 8.3 and 14.1 eV for CsPbI3 as a result of photonic energy absorption, which is shown in Fig. 6(c).
Reflectivity is an important optical property that determines the surface nature of the materials for optoelectronic and solar cell applications.128 Fig. 6(d) shows the reflectivity spectra of CsPbX3 perovskites. From these data, it can be seen that the reflectivity is very low for all the studied cubic perovskite compounds; however, the reflectivity shows maximum for CsPbI3 and minimum for CsPbCl3 perovskites, at the optical range. Some peaks arise in each spectrum, which are the responses still true for infra-red and visible regions. In the visible region, the first peaks are observed at 4.2, 3.8 and 3.3 eV for CsPbCl3, CsPbBr3 and CsPbI3, respectively. Some other peaks are observed at 7.8, 11.5, 15.6, 20.4 eV for CsPbCl3, at 7.3, 10.9, 15.4 eV for CsPbBr3 and at 6.3, 9.0, 11.3, 15.8 eV for CsPbI3. From the comparison of reflectivity, it is clearly seen that CsPbI3 shows the most homogeneous response over a wide energy range in the ultraviolet region.
3.5 Redox potentials: photocatalytic activity
The photocatalytic activity of material depends highly on the suitable band gap, the recombination rate of carriers, and proper redox potentials. An idealization arises from a narrow band gap, low recombination rate with long-life carriers, and proper redox potentials of the compounds irradiated under visible light. The redox ability for visible light exposure can be calculated theoretically by aligning the CBM and VBM compared to the water reduction/oxidation potential level (Fig. 7). The positions of CBM and VBM can theoretically be predicted by employing the following equations,129
ECB = X − Ee + 0.5Eg and EVB = ECB + Eg |
where ECB and EVB are the CB and VB edge potentials, X is the absolute electronegativity of CsPbX3. Absolute or Mulliken electronegativity is numerically equal to the geometric mean of electronegativities of ingredient elements where the first ionization energy and the atomic electron affinity are well employed.130 Eg is the electronic band gap value and Ee is the energy of free electrons on the hydrogen scale (4.5 eV).
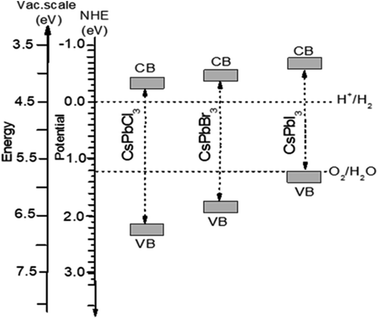 |
| Fig. 7 Band edge potentials of CsPbX3 (X = Cl, Br, I) for CBM and VBM estimated under GGA–PBE functions by applying the band structure and band gap values. | |
The calculated electronegativities, X are 5.56 eV, 5.17 eV, and 4.83 eV, whereas the electronic band gaps Eg are observed (in Fig. 4) as 2.385 eV, 2.061 eV and 1.784 eV for CsPbCl3, CsPbBr3 and CsPbI3 respectively. The CBM potentials occurred at −0.24 eV, −0.36 eV, −0.57 eV versus NHE and the VBM potentials were at 2.15 eV, 1.70 eV and 1.22 eV for CsPbCl3, CsPbBr3 and CsPbI3, respectively. By comparing these values, it is seen that the CBM-negative responses can be ordered as CsPbCl3 < CsPbBr3 < CsPbI3 to the H+/H2 level and the VBM can be ordered as CsPbCl3 > CsPbBr3 > CsPbI3 with positive to O2/H2O (1.23 eV). Analysis of these results provides explicit evidence that CsPbCl3 is one of the best candidates for its strong ability of water oxidation from the thermodynamics aspect generating O2, whereas CsPbI3 is the best candidate as an H2 producing agent due to its strong redox potential. In contrast, CsPbBr3 was found to be the best performer for the photocatalytic process in the visible region due to nearly equal effectiveness to both the oxidation and reduction processes.
4. Conclusions
The above mentioned properties investigation compares the applicability of these materials in optoelectronic, photocatalytic, and photovoltaic (PV) devices. We calculated structural, and elastic properties to determine the better agreement of damage-tolerance and electronic and optical responses for suitable device applications. The optimized lattice constant and elastic constant showed excellent agreement with the experimental data whereas some properties were found to be much better when compared to other theoretical reports. CsPbBr3 is thermally more stable but more brittle than the other two perovskites. From a comparative study, it is seen that CsPbCl3, CsPbBr3, and CsPbI3 are suitable for green, orange, and red emissions, respectively, of the optical spectra, owing to the proper electronic band gap. Theoretically, it was observed that CsPbI3 is the best photocatalyst for the hydrogen evolution reaction and CsPbBr3 is the most stable photocatalyst due to its nearly balanced oxidation and reduction potentials, but CaPbCl3 is better for O2 production. Thus, pressure can be employed to tune the properties of materials by adjusting their lattice parameters, electronic orbitals, crystal structure interatomic distances, and bonding patterns for efficient electronic and optoelectronic applications.
Author contributions
M. Aktary performed conceptualization, designed the study, interpreted the data, and wrote the manuscript. M. Kamruzzaman helped study, data analysis, and wrote the manuscript. R. Afrose Helped us to understand software, DFT calculations, data curation, writing-review, writing and editing the manuscript.
Conflicts of interest
The authors declare no competing financial interests.
Acknowledgements
The authors would like to thank the Department of Physics, Begum Rokeya University, Rangpur, Rangpur-5400 for supporting the computer facility. M. Aktary would like to dedicate this paper to her beloved father Hasinur Rahman for his kind and cordial supports during this research.
References
- A. Hagfeldt, G. Boschloo, L. Sun, L. Kloo and H. Pettersson, Chem. Rev., 2010, 110, 6595–6663 CrossRef CAS PubMed.
- J. Burschka, N. Pellet, S.-J. Moon, R. Humphry-Baker, P. Gao, M. K. Nazeeruddin and M. Grätzel, Nature, 2013, 499, 316 CrossRef CAS PubMed.
- M. Liu, M. B. Johnston and H. J. Snaith, Nature, 2013, 501, 395 CrossRef CAS PubMed.
- W. B. Xiao, M. M. Liu and C. Yan, J. Nanoelectron. Optoelectron., 2017, 12, 189–195 CrossRef CAS.
- H.-J. Park, K.-H. Lee, B. Kumar, K.-S. Shin, S.-W. Jeong and S.-W. Kim, J. Nanoelectron. Optoelectron., 2010, 5, 135–138 CrossRef CAS.
- A. Yella, H. W. Lee, H. N. Tsao, C. Y. Yi and A. K. Chandiran, Science, 2011, 334, 629 CrossRef CAS PubMed.
- N. J. Jeon, J. H. Noh, W. S. Yang, Y. C. Kim, S. Ryu, J. Seo and S. I. Seok, Nature, 2015, 517, 476 CrossRef CAS PubMed.
- M. M. Lee, J. Teuscher, T. Miyasaka, T. N. Murakami and H. J. Snaith, Science, 2012, 338, 643–647 CrossRef CAS PubMed.
- C. H. Chiang, M. K. Nazeeruddin, M. Gratzel and C. G. Wu, Energy Environ. Sci., 2017, 10, 808–817 RSC.
- T. R. Cook, D. K. Dogutan, S. Y. Reece, Y. Surendranath, T. S. Teets and D. G. Nocera, Chem. Rev., 2010, 110, 6474–6502 CrossRef CAS PubMed.
- J. H. Bang and P. V. Kamat, ACS Nano, 2009, 3, 1467–1476 CrossRef CAS PubMed.
- Z. Pan, K. Zhao, J. Wang, H. Zhang, Y. Feng and X. Zhong, ACS Nano, 2013, 7, 5215 CrossRef CAS PubMed.
- M. Jorgensen, K. Norrman and F. C. Krebs, Sol. Energy Mater. Sol. Cells, 2008, 92, 686–714 CrossRef.
- K. Yoshikawa, H. Kawasaki, W. Yoshida, T. Irie, K. Konishi, K. Nakano, T. Uto, D. Adachi, M. Kanematsu, H. Uzu and K. Yamamoto, Nat. Energy, 2017, 2, 17032 CrossRef CAS.
- M. D. Kelzenberg, S. W. Boettcher, J. A. Petykiewicz, D. B. Turner-Evans, M. C. Putnam, E. L. Warren, J. M. Spurgeon, R. M. Briggs, N. S. Lewis and H. A. Atwater, Nat. Mater., 2010, 9, 239–244 CrossRef CAS PubMed.
- A. Kojima, K. Teshima, T. Miyasaka and Y. Shirai, 210th ECS Meeting, ECS, 2006 Search PubMed.
- A. M. M. Tanveer Karim, M. K. R. Khan, M. S. Hossain, M. Kamruzzaman, M. Azizar Rahman and M. Mozibur Rahman, Mater. Sci. Eng., B, 2020, 259, 114599 CrossRef CAS.
- A. Kojima, K. Teshima, Y. Shirai and T. Miyasaka, J. Am. Chem. Soc., 2009, 131, 6050–6051 CrossRef CAS PubMed.
- M. Jošt, E. Köhnen, A. B. Morales-Vilches, B. Lipovšek, K. Jäger, B. Macco, A. Al-Ashouri, J. Krč, L. Korte, B. Rech, R. Schlatmann, M. Topič, B. Stannowski and S. Albrecht, Energy Environ. Sci., 2018, 11, 3511–3523 RSC.
- J. F. Geisz, R. M. France, K. L. Schulte, M. A. Steiner, A. G. Norman, H. L. Guthrey, M. R. Young, T. Song and T. Moriarty, Nat. Energy, 2020, 5, 326–335 CrossRef CAS.
- W. Zhang, G. E. Eperon and H. J. Snaith, Nat. Energy, 2016, 1, 16048 CrossRef CAS.
- A. Swarnkar, A. R. Marshall, E. M. Sanehira, B. D. Chernomordik, D. T. Moore, J. A. Christians, T. Chakrabarti and J. M. Luther, Science, 2016, 354, 92–95 CrossRef CAS PubMed.
- W. J. Yin, T. Shi and Y. Yan, Adv. Mater., 2014, 26, 4653–4658 CrossRef CAS PubMed.
- K. P. Marshall, M. Walker, R. I. Walton and R. A. Hatton, Nat. Energy, 2016, 1, 16178 CrossRef CAS.
- R. J. Sutton, G. E. Eperon, L. Miranda, E. S. Parrott, B. A. Kamino, J. B. Patel, M. T. Hörantner, M. B. Johnston, A. A. Haghighirad, D. T. Moore and H. J. Snaith, Adv. Energy Mater., 2016, 6, 1502458 CrossRef.
- J. B. Hoffman, A. L. Schleper and P. V. Kamat, J. Am. Chem. Soc., 2016, 138, 8603–8611 CrossRef CAS PubMed.
- L. Y. Huang and W. R. Lambrecht, Phys. Rev. B, 2016, 93, 195211 CrossRef.
- Q. A. Akkerman, M. Gandini, F. D. Stasio, P. Rastogi, F. Palazon, G. Bertoni, J. M. Ball, M. Prato, A. Petrozza and L. Manna, Nat. Energy, 2016, 2, 16194 CrossRef.
- G. E. Eperon, G. M. Paternò, R. J. Sutton, A. Zampetti, A. A. Haghighirad, F. Cacialli and H. J. Snaith, J. Mater. Chem., 2015, 3, 19688–19695 RSC.
- T. Krishnamoorthy, H. Ding, C. Yan, W. L. Leong, T. Baikie, Z. Zhang, M. Sherburne, S. Li, M. Asta, N. Mathews and S. G. Mhaisalkar, J. Mater. Chem., 2015, 3, 23829–23832 RSC.
- P. Ramasamy, D. H. Lim, B. Kim, S. H. Lee, M. S. Lee and J. S. Lee, Chem. Commun., 2016, 52, 2067–2070 RSC.
- P. Cottingham and R. L. Brutchey, Chem. Commun., 2016, 1–3 Search PubMed.
- D. P. Rai, Sandeep, A. Shankar, A. P. Sakhya, T. P. Sinha, B. Merabet, M. Musa Saad H.-E, R. Khenata, A. Boochani, S. Solaymani and R. K. Thapa, Mater. Chem. Phys., 2017, 186, 620–626 CrossRef CAS.
- Sandeep, D. P. Rai, A. Shankar, M. P. Ghimire, R. Khenata and R. K. Thapa, J. Magn. Magn. Mater., 2016, 417, 313–320 CrossRef CAS.
- Sandeep, D. P. Rai, A. Shankar, R. Khenata, M. P. Ghimire, R. K. Thapa and S. B. Omran, Int. J. Mod. Phys. B, 2016, 30(12), 1650078 CrossRef.
- Sandeep, D. P. Rai, A. Shankar, M. P. Ghimire, R. Khenata and R. K. Thapa, Phys. Scr., 2015, 90, 065803 CrossRef.
- J. Qiu, Y. Qiu, K. Yan, M. Zhong, C. Mu, H. Yan and S. Yang, Nanoscale, 2013, 5, 3245–3248 RSC.
- L. Lang, J. H. Yang, H. R. Liu, H. J. Xiang and X. G. Gong, Phys. Lett. A, 2014, 378, 290–293 CrossRef CAS.
- G. Murtaza and I. Ahmad, Phys. B, 2011, 406, 3222–3229 CrossRef CAS.
- H. Jing, R. Sa and G. Xu, Chem. Phys. Lett., 2019, 732, 136642 CrossRef CAS.
- Z. L. Ku, Y. G. Rong, M. Xu, T. F. Liu and H. W. Han, Sci. Rep., 2013, 3, 3132 CrossRef PubMed.
- H. S. Kim, C. R. Lee, J. H. Im, K. B. Lee, T. Moehl, A. Marchioro, S. J. Moon, R. Humphry-Baker, J. H. Yum, J. E. Moser, M. Grätzel and N. G. Park, Sci. Rep., 2012, 2, 591 CrossRef PubMed.
- H. J. Snaith, J. Phys. Chem. Lett., 2013, 4, 3623–3630 CrossRef CAS.
- C. Katan, N. Mercier and J. Even, Chem. Rev., 2019, 119, 3140–3192 CrossRef CAS PubMed.
- Z. K. Tan, R. S. Moghaddam, M. L. Lai, P. Docampo, R. Higler, F. Deschler, M. Price, A. Sadhanala, L. M. Pazos, D. Credgington, F. Hanusch, T. Bein, H. J. Snaith and R. H. Friend, Nat. Nanotechnol., 2014, 9, 46 Search PubMed.
- M. A. Fadla, B. Bentria, T. Dahame and A. Benghia, Phys. B, 2020, 585, 412118 CrossRef CAS.
- M. Baranowski, P. Plochocka, R. Su, L. Legrand, T. Barisien, F. Bernardot, Q. Xiong, C. Testelin and M. Chamarro, Photonics Res., 2020, 8, A50–A55 CrossRef CAS.
- G. Morello, M. De Giorgi, S. Kudera, L. Manna, R. Cingolani and M. Anni, J. Phys. Chem. C, 2007, 111, 5846–5849 CrossRef CAS.
- N. Pandey, A. Kumar and S. Chakrabarti, RSC Adv., 2019, 9, 29556 RSC.
- L. Protesescu, S. Yakunin, M. Bodnarchuk and F. Krieg, Nano Lett., 2015, 15, 3692–3696 CrossRef CAS PubMed.
- Y. Xu, Q. Chen, C. Zhang, R. Wang, H. Wu, X. Zhang, G. Xing, W. W. Yu, X. Wang, Y. Zhang and M. Xiao, J. Am. Chem. Soc., 2016, 138, 3761–3768 CrossRef CAS PubMed.
- Z. K. Tan, R. S. Moghaddam, M. L. Lai, P. Docampo, R. Higler, F. Deschler, M. Price, A. Sadhanala, L. M. Lazos, D. Credgington, F. Hanusch, T. Bein, H. J. Snaith and R. H. Friend, Nat. Nanotechnol., 2014, 9, 687–692 CrossRef CAS PubMed.
- H. Zhu, Y. Fu, F. Meng, X. Wu, Z. Gong, Q. Ding, M. V. Gustafsson, M. T. Trinh, S. Jin and X. Y. Zhu, Nat. Mater., 2015, 14, 636–642 CrossRef CAS PubMed.
- J. Deng, J. Li, Z. Yang and M. Wang, J. Mater. Chem. C, 2019, 7, 12415–12440 RSC.
- C. C. Stoumpos, C. D. Malliakas, J. A. Peters, Z. Liu, M. Sebastian, J. Im, T. C. Chasapis, A. C. Wibowo, D. Y. Chung and A. J. Freeman, et al., Cryst. Growth Des., 2013, 13, 2722–2727 CrossRef CAS.
- X. Li, Y. Tan, H. Lai, S. Li, Y. Chen, S. Li, P. Xu and J. Yang, ACS Appl. Mater. Interfaces, 2019, 11, 29746–29752 CrossRef CAS PubMed.
- H. Zhang, X. Liu, J. Dong, H. Yu, C. Zhou, B. Zhang, Y. Xu and W. Jie, Cryst. Growth Des., 2017, 17, 6426–6431 CrossRef CAS.
- D. N. Dirin, I. Cherniukh, S. Yakunin, Y. Shynkarenko and M. V. Kovalenko, Chem. Mater., 2016, 28, 8470–8474 CrossRef CAS PubMed.
- P. Ramasamy, D. H. Lim, B. Kim, S. H. Lee, M. S. Lee and J. S. Lee, Chem. Commun., 2016, 52, 2067–2070 RSC.
- Y. Lee, J. Kwon, E. Hwang, C. H. Ra, W. J. Yoo, J. H. Ahn, J. H. Park and J. H. Cho, Adv. Mater., 2015, 27, 41–46 CrossRef CAS PubMed.
- Y. Wang, Y. Zhang, Y. Lu, W. Xu, H. Mu, C. Chen, H. Qiao, J. Song, S. Li, B. Sun, Y. B. Cheng and Q. Bao, Adv. Opt. Mater., 2015, 3, 1389–1396 CrossRef CAS.
- W. Y. Nie, H. H. Tsai, R. Asadpour, J. C. Blancon, A. J. Neukirch, G. Gupta, J. J. Crochet, M. Chhowalla, S. Tretiak, M. A. Alam, H. L. Wang and A. D. Mohite, Science, 2015, 347, 522–525 CrossRef CAS PubMed.
- M. A. Green, A. Ho-Baillie and H. J. Snaith, Nat. Photonics, 2014, 8, 506–514 CrossRef CAS.
- G. Xing, N. Mathews, S. Sun, S. S. Lim, Y. M. Lam, M. Gratzel, S. Mhaisalkar and T. C. Sum, Science, 2013, 342, 344–347 CrossRef CAS PubMed.
- X. Li, F. Cao, D. Yu, J. Chen, Z. Sun, Y. Shen, Y. Zhu, L. Wang, Y. Wei, Y. Wu and H. Zeng, Small, 2017, 13, 1603996 CrossRef PubMed.
- X. Li, Y. Liu, X. Song, H. Wang, H. Gu and H. Zeng, Angew. Chem., Int. Ed. Engl., 2015, 54, 1759–1764 CrossRef CAS PubMed.
- J. Song, J. Li, X. Li, L. Xu, Y. Dong and H. Zeng, Adv. Mater., 2015, 27, 7162–7167 CrossRef CAS PubMed.
- J. Li, L. Xu, T. Wang, J. Song, J. Chen, J. Xue, Y. Dong, B. Cai, Q. Shan, B. Han and H. Zeng, Adv. Mater., 2017, 29, 1603885 CrossRef PubMed.
- K. Lin, J. Xing, L. N. Quan, F. P. G. de Arquer, X. Gong, J. Lu, L. Xie, W. Zhao, D. Zhang, C. Yan, W. Li, X. Liu, Y. Lu, J. Kirman, E. H. Sargent, Q. Xiong and Z. Wei, Nature, 2018, 562, 245–248 CrossRef CAS PubMed.
- Y. Wang, X. Li, J. Song, L. Xiao, H. Zeng and H. Sun, Adv. Mater., 2015, 27, 7101–7108 CrossRef CAS PubMed.
- Q. Zhang, R. Su, W. Du, X. Liu, L. Zhao, S. T. Ha and Q. Xiong, Small Methods, 2017, 1, 1700163 CrossRef.
- R. Su, C. Diederichs, J. Wang, T. C. H. Liew, J. Zhao, S. Liu, W. Xu, Z. Chen and Q. Xiong, Nano Lett., 2017, 17, 3982–3988 CrossRef CAS PubMed.
- R. J. Sutton, G. E. Eperon, L. Miranda, E. S. Parrott, B. A. Kamino, J. B. Patel, M. T. Hörantner, M. B. Johnston, A. A. Haghighirad, D. T. Moore and H. J. Snaith, Adv. Energy Mater., 2016, 6, 1502458 CrossRef.
- E. M. Senehira, A. R. Marshall, J. A. Christians, S. P. Harvey, P. N. Ciesielski, L. M. Wheeler, P. Schulz, L. Y. Lin, M. C. Beard and J. M. Luther, Sci. Adv., 2017, 3, 4204 CrossRef PubMed.
- P. Ramasamy, D. M. Lim, B. Kim, S. H. Lee, M. S. Lee and J. S. Lee, Chem. Commun., 2016, 52, 2067–2070 RSC.
- S. Wang, K. Wang, Z. Gu, Y. Wang, C. Huang, N. Yi, S. Xiao and Q. Song, Adv. Opt. Mater., 2017, 5, 1700023 CrossRef.
- H. M. Ghaithan, Z. A. Alahmed, S. M. H. Qaid, M. Hezam and A. S. Aldwayyan, ACS Omega, 2020, 5, 7468–7480 CrossRef CAS PubMed.
- T. G. Liashenko, E. D. Cherotchenko, A. P. Pushkarev, V. Pakštas, A. Naujokaitis, S. A. Khubezhov, R. G. Polozkov, K. B. Agapev, A. A. Zakhidov, I. A. Shelykh and S. V. Makarov, Phys. Chem. Chem. Phys., 2019, 21, 18930–18938 RSC.
- S. Plesko, R. Kind and J. Roos, J. Phys. Soc. Jpn., 1978, 45, 553–557 CrossRef CAS.
- F. Zhang, H. Zhong, C. Chen, X. Wu, X. Hu, H. Huang, J. Han, B. Zou and Y. Dong, ACS Nano, 2015, 9, 4533 CrossRef CAS PubMed.
- L. Protesescu, S. Yakunin, M. I. Bodnarchuk, F. Krieg, R. Caputo, C. H. Hendon, R. X. Yang, A. Walsh and M. V. Kovalenko, Nano Lett., 2015, 15, 3692 CrossRef CAS PubMed.
- O. Yu. Posudievsky, N. V. Konoshchuk, V. L. Karbivskyy, O. P. Boiko, V. G. Koshechko and V. D. Pokhodenk, Theor. Exp. Chem., 2017, 53, 235–243 CrossRef CAS.
- G. E. Eperon, G. M. Paterno, R. J. Sutton, A. Zampetti, A. A. Haghighirad, F. Cacialli and H. J. Snaith, J. Mater. Chem. A, 2015, 3, 19688–19695 RSC.
- J. A. Steele, H. Jin, I. Dovgaliuk, R. F. Berger, T. Braeckevelt, H. Yuan, C. Martin, E. Solano, K. Lejaeghere, S. M. J. Rogge, C. Notebaert, W. Vandezande, K. P. F. Janssen, B. Goderis, E. Debroye, Y. K. Wang, Y. Dong, D. Ma, M. Saidaminov, H. Tan, Z. Lu, V. Dyadkin, D. Chernyshov, V. V. Speybroeck, E. H. Sargent, J. Hofkens and M. B. J. Roeffaers, Science, 2019, 365, 679 CrossRef CAS PubMed.
- J. B. Hoffman, A. L. Schleper and P. V. Kamat, J. Am. Chem. Soc., 2016, 138, 8603–8611 CrossRef CAS PubMed.
- S. De Wolf, J. Holovsky, S. J. in Moon, P. Löper, B. Niesen, M. Ledinsky, F. J. Haug, J. H. Yum and C. Ballif, J. Phys. Chem. Lett., 2014, 5, 1035–1039 CrossRef CAS PubMed.
- G. Liu, L. Kong, W. Yang and H.-k. Mao, Mater. Today, 2019, 27, 91 CrossRef CAS.
- M. Coduri, T. B. Shiell, T. A. Strobel, A. Mahata, F. Cova, E. Mosconi, F. De Angelis and L. Malavasi, Mater. Adv., 2020, 1, 2840 RSC.
- M. I. Kholil and M. T. H. Bhuiyan, J. Phys. Chem. Solids, 2021, 154, 110083 CrossRef CAS.
- Y. Li, J. Liu, P. Zhang, J. Zhang, N. Xiao, L. Yu and P. Niu, J. Mater. Sci., 2020, 55, 14873 CrossRef CAS.
- J.-H. Lee, A. Jaffe, Y. Lin, H. I. Karunadasa and J. B. Neaton, ACS Energy Lett., 2020, 5, 2174 CrossRef CAS.
- O. V. Oyelade, O. K. Oyewole, D. O. Oyewole, S. A. Adeniji, R. Ichwani, D. M. Sanni and W. O. Soboyejo, Sci. Rep., 2020, 10, 7183 CrossRef CAS PubMed.
- S. Yalameha, P. Saeidi, Z. Nourbakhsh and A. V. A. Ramazani, J. Appl. Phys., 2020, 127, 085102 CrossRef CAS.
- R. Y. Alyoubi, B. M. Raffah, F. Hamioud and A. A. Mubarak, Mod. Phys. Lett. B, 2021, 35, 2150056 CrossRef CAS.
- S. Idrissi, H. Labrim, S. Ziti and L. Bahmad, J. Appl. Phys., 2020, 126(3), 190 CrossRef CAS.
- S. Idrissi, H. Labrim, S. Ziti and L. Bahmad, Phys. Lett. A, 2020, 384, 126453 CrossRef CAS.
- S. Idrissi, H. Labrim, S. Ziti and L. Bahmad, J. Supercond. Novel Magn., 2020, 33, 3087–3095 CrossRef.
- P. Hohenberg and W. Kohn, Phys. Rev. B, 1964, 136, 864 CrossRef.
- W. Kohn and L. J. Sham, Phys. Rev. A, 1965, 140, 1133 CrossRef.
- S. J. Clark, M. D. Segall, C. J. Pickard, P. J. Hasnip, M. I. Probert, K. Refson and M. C. Payne, Z. Kristallogr., 2005, 220, 567 CAS.
- J. Hutton, R. J. Nelmes, G. M. Meyer and V. R. Eiriksson, J. Phys. C Solid State Phys., 1979, 12, 5393–5410 CrossRef CAS.
- M. Ahmad, G. Rehman, L. Ali, M. Shafiq, R. Iqbal, R. Ahmad, T. Khan, S. Jalali-Asadabadi, M. Maqbool and I. Ahmad, J. Alloys Compd., 2017, 705, 828–839 CrossRef CAS.
- M. Roknuzzaman, K. Ostrikov, H. Wang, A. Du and T. Tesfamichael, Sci. Rep., 2017, 7, 14025 CrossRef PubMed.
- S. Idrissi a, S. Ziti b, H. Labrim c and L. Bahmad, Mater. Sci. Semicond. Process., 2021, 122, 105484 CrossRef.
- M. A. Ghebouli, B. Ghebouli and M. Fatmi, Phys. B, 2011, 406, 1837–1843 CrossRef CAS.
- A. Najim, B. Hartiti, H. Absike, H. J. T. Nkuissi, H. Labrim, S. Fadili, P. Thevenin and M. Ertugrul, Mater. Sci. Semicond. Process., 2022, 141, 106442 CrossRef CAS.
- H. Joshi, R. K. Thapa, A. Laref, W. Sukkabot, L. Pachuau, L. Vanchhawng, P. Grima-Gallardofgh, M. Musa Saad H-E and D. P. Rai, Surf. Interfaces, 2022, 30, 101829 CrossRef CAS.
- Z. Zhao, M. Zhong, W. Zhou, Y. Peng, Y. Yin, D. Tang and B. Zou, J. Phys. Chem. C, 2019, 123, 25349–25358 CrossRef CAS.
- L. K. Gao and Y. L. Tang, Theoretical Study on the Carrier Mobility and Optical Properties of CsPbI3 by DFT, ACS Omega, 2021, 6(17), 11545–11555 CrossRef CAS PubMed.
- D. M. Trots and S. V. Myagkota, J. Phys. Chem. Solids, 2008, 69, 2520–2526 CrossRef CAS.
- M. Born, Math. Proc. Cambridge Philos. Soc., 1940, 36, 160 CrossRef CAS.
- J. M. J. den Toonder, J. A. W. van Dommelen and F. P. T. Baaijens, Modell. Simul. Mater. Sci. Eng., 1999, 7, 909 CrossRef CAS.
- M. H. K. Rubel, M. A. Hadi, M. M. Rahaman, M. S. Ali, M. Aftabuzzaman, R. Parvin, A. K. M. A. Islam and N. Kumada, Comput. Mater. Sci., 2017, 138, 160 CrossRef CAS.
- M. Roknuzzaman, M. A. Hadi, M. A. Ali, M. M. Hossain, N. Jahan, M. M. Uddin, J. A. Alarco and K. Ostrikov, J. Alloys Compd., 2017, 727, 616 CrossRef CAS.
- M. A. Hadi, M. N. Islam and M. H. Babu, Z. Naturforsch., 2019, 74, 71–81 CrossRef CAS.
- D. G. Pettifor, Mater. Sci. Technol., 1992, 8, 345 CrossRef CAS.
- S. F. Pugh, Philos. Mag., 1954, 45, 823 CAS.
- M. E. Fine, L. D. Brown and H. L. Marcus, Scr. Metall., 1984, 18, 951 CrossRef CAS.
- T. Paul, B. K. Chatterjee, S. Maiti, S. Sarkar, N. Besra, B. K. Das, K. J. Panigrahi, S. Thakur1, U. K. Ghorai and K. K. Chattopadhyay, J. Mater. Chem. C, 2018, 6, 3322–3333 RSC.
- G. E. Eperon, S. D. Stranks, C. Menelaou, M. B. Johnston, L. M. Herz and H. J. Snaith, Energy Environ. Sci., 2014, 7, 982–988 RSC.
- Li Lang, Y.-Yu Zhang, P. Xu, S. Chen, H. J. Xiang and X. G. Gong, Phys. Rev. B, 2015, 92, 075102 CrossRef.
- K. Heidrich, W. Schafer, M. Schreiber, J. Sochtig, G. Trendel and J. Treusch, Phys. Rev. B: Condens. Matter, 1981, 24, 5642 CrossRef CAS.
- R. L. Moreira and A. Dias, J. Phys. Chem. Solids, 2007, 68, 1617 CrossRef CAS.
- J. Islam and A. K. M. A. Hossain, Sci. Rep., 2020, 10, 14542 CrossRef PubMed.
- X. Liu, B. Xie, C. Duan, Z. Wang, B. Fan, K. Zhang, B. Lin, F. J. M. Colberts, W. Ma, R. A. J. Janssen, F. Huang and Y. Cao, J. Mater. Chem. A, 2018, 6, 395 RSC.
- S. Saha, T. P. Sinha and A. Mookerjee, Phys. Rev. B, 2000, 62, 8828 CrossRef CAS.
- M. I. Kholil, M. T. H. Bhuiyan, M. A. Rahman, M. S. Ali and M. Aftabuzzaman, RSC Adv., 2021, 11, 2405 RSC.
- Y. Saeed, B. Amin, H. Khalil, F. Rehman, H. Ali, M. I. Khan, A. Mahmood and M. Shafiq, RSC Adv., 2020, 10, 17444 RSC.
- Y. Gong, H. Yu and X. Quan, Int. J. Photoenergy, 2014, 2014, 5 CrossRef.
- J. Liu, S. Chen, Q. Liu, Y. Zhu and J. Zhang, Chem. Phys. Lett., 2013, 572, 101–105 CrossRef CAS.
|
This journal is © The Royal Society of Chemistry 2022 |
Click here to see how this site uses Cookies. View our privacy policy here.