DOI:
10.1039/D2RA04348C
(Paper)
RSC Adv., 2022,
12, 31186-31191
A “turn-on” ESIPT fluorescence probe of 2-(aminocarbonyl)phenylboronic acid for the selective detection of Cu(II)†
Received
14th July 2022
, Accepted 6th October 2022
First published on 31st October 2022
Abstract
Herein, we report a highly selective fluorescent probe for the detection of Cu(II). The detection mechanism relies on the Cu(II)-catalyzed oxidative hydroxylation of 2-(aminocarbonyl)phenylboronic acid into salicylamide, thus recovering the excited-state intramolecular proton transfer (ESIPT) effect and inducing more than 35-fold fluorescence enhancement. The simple structure and readily available fluorescent probe give a novel method for quantitatively detecting Cu(II) in the linear range of 0–22 μM, with a limit of detection down to 68 nM, and exhibiting high selectivity for Cu(II) over 16 other metal ions.
1. Introduction
As one of the critical heavy metal elements, the level of copper ion content significantly impacts the ecological environment and human health. Especially after biological enrichment, excessive Cu(II) causes metabolic and neurological disorders, leading to rheumatoid arthritis, Wilson's syndrome, Parkinson's disease, and Alzheimer's disease.1–3 Therefore, it is of great significance to develop an efficient detection assay for Cu(II) in clinical diagnosis and disease prevention.
In recent years, powerful assays for Cu(II) detection have been developed, including titrimetry,4 UV-Vis spectrophotometry,5 atomic absorption spectrophotometry (AAS),6 inductively coupled plasma-atomic emission spectroscopy (ICP-AES),7 ion selective electrode (ISE),8 and chemiluminescence.9 Among various analysis methods, the fluorescent probe is an appealing tool for Cu(II) detection owing to its simple operation, high sensitivity and in suit detection in living cells.10–12
Nowadays, several fluorescent probes for Cu(II) detection based on the sensing strategy of coordination or chemical reaction have been designed. However, based on the paramagnetic characteristics of Cu(II), the complex generated from the probe and Cu(II) ion usually leads to fluorescent quenching, which gives false-positive results due to the lower sensitivity and anti-interference property.13 Alternatively, reaction-based fluorescence probes exhibit higher sensitivity and selectivity owing to the fluorescent signal change related to the unique chemical reaction between analyte and probe molecular.14–16 Gratifying examples of fluorescence enhancement probes for Cu(II) have been reported based on distinct reactions, such as the Cu(II)-induced hydrolysis,17,18 ring-opening of the spirolactam,19,20 C–O bond cleavage,21–23 Cu(II)-mediated oxidation reaction of o-phenylenediamine,24–26 Cu(II)-catalyzed Heck coupling and click reaction.27–29 Among these probes, the boronic ester/acid-based fluorescence probe possesses excellent biocompatibility, which facilitates the in situ detection of intracellular Cu(II), and has been favored in recent years.30 However, the borate groups mostly bind with the fluorophore, such as coumarin,31 fluorescein,32 resorufin,33 benzotriazole34 by chemical modification, leading to challenges in synthetic procedure and high cost. Therefore, it is urgent to develop a new fluorescence probe of Cu(II) using arylboronic acid with a simple structure and low cost.
Salicylamide, the simplest derivative of salicylic acid, has long been used as an anti-bacterial and anti-viral drug.35,36 Notably, salicylamide has attracted considerable attention because of its excited-state intramolecular proton transfer (ESIPT) fluorescent property, which was first reported by Weller in the 1950s for salicylic acid.37 In general, molecules can exhibit ESIPT fluorescence if their structures incorporate an intramolecular hydrogen bonding interaction between a hydrogen bond donor (–OH and NH2) and a hydrogen bond acceptor (
N– and C
O), and the molecular structure of salicylamide fits this feature.38,39 Therefore, we envision that 2-(aminocarbonyl)phenylboronic acid could be utilized as a “turn-on” fluorescent probe for the detection of Cu(II), in which the boronic acid group acts as the recognition group of Cu(II) and hinders the ESIPT effect of the probe molecule. By employing the Cu(II) catalytic oxidative hydroxylation of the boronic acid group to the hydroxyl group, the ESIPT effect can be recovered owing to keto–enol tautomerism. The ESIPT process starts with the absorption of radiation from an enol cis conformer (1E) and into the excited state (1E*), this conformer undergoes ESIPT to form a keto tautomer (1K*) giving rise to an emission, leading to the fluorescent “turn on” (Scheme 1). Using widely distributed and cheap 2-(Aminocarbonyl)phenylboronic acid as a fluorescence probe, we provide a concise and efficient assay for quantitatively determining Cu(II).
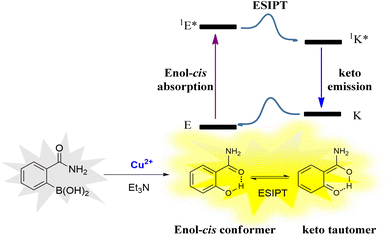 |
| Scheme 1 Detection mechanism of the probe and the ESIPT process of salicylamide. | |
2. Experimental section
2.1. Materials and methods
2-(Aminocarbonyl)phenylboronic acid, Et3N, Cu(OAc)2·H2O, EtOH, ACN, DMSO, DMF, KCl, NaCl, CaCl2, MgCl2·6H2O, Sr(NO3)2, AgNO3, ZnCl2, MnCl2·4H2O, CoCl2·6H2O, Pd(OAc)2, HgCl2, FeCl3, CrCl3, AlCl3, Pr(NO3)3·6H2O, and ceric ammonium nitrate were purchased from Shanghai Aladdin Bio-Chem Technology Co, Ltd. and used as received. The testing solvents were prepared with deionized water.
2.2. General instrumentation
Emission spectra were performed on a fluorescence spectrometer (F-4600). Error limits were estimated: λ (±1 nm), τ (±10%), and φ (±10%). 1H NMR (400 MHz) and 13C NMR (100 MHz) spectra were recorded on a Bruker Avance AV400 spectrometer (Bruker, Billerica, MA, USA) unless otherwise noted. The chemical shifts (δ) were quoted in parts per million from tetramethylsilane for 1H and DMSO-d6 for 13C spectroscopy. The pH values of the real water samples were recorded by applying B6337-02 benchtop pH/conductivity meters.
2.3. Fluorescence detection of Cu(II)
The experimental details of the fluorescent detection condition optimization are provided in the ESI.† As for sensitivity testing, 2-(aminocarbonyl)phenylboronic acid (100 μM), Et3N (150 μM) and the various concentrations of Cu(II) (0–96 μM) were added in a cuvette sequentially. After the above-mixed solutions reacted for 2.5 h, the fluorescence spectra were measured and recorded using a fluorescence spectrophotometer.
The limit of detection (LOD) is calculated using the following formula:
where
σ is the standard deviation of the blank signals (
n = 3).
2.4. Selectivity and anti-interference experiments
Various metal ion interferents (2 mM K+ and Na+, 1 mM Ca2+ and Mg2+, 50 μM Sr2+, Ag+, Zn2+, Mn2+, Co2+, Pd2+, Hg2+, Fe3+, Cr3+, Al3+, Pr3+, Ce4+), instead of Cu2+, were added to the fluorescent probe solution, and then 50 μM Cu2+ was mixed with this solution to explore the anti-interference capacity of the probe.
2.5. Fluorescence detection of Cu(II) in real water samples
Ganjiang River water was collected from the Ganjiang River, tap water was collected from a chemistry laboratory, and rainwater was collected from Ganzhou. Cu(II) was spiked into various water samples to obtain sample stock solutions with 5 mM of Cu(II). Real water samples were added to a sensing system containing 2-(aminocarbonyl)phenylboronic acid (100 μM) and Et3N (150 μM), resulting in a final solution with 20 μM of Cu(II) in the DMF solution. Then, their fluorescent spectra were measured and recorded by applying a fluorescence spectrometer. All average values were obtained from three parallel experiments (n = 3).
3. Results and discussion
To verify the design hypothesis, the fluorescence response of 2-(aminocarbonyl)phenylboronic acid to Cu(II) in various solvents was tested, as shown in Fig. 1a. The solutions of 2-(aminocarbonyl)phenylboronic acid alone exhibit weak fluorescence emission above 400 nm, while the maximum fluorescence enhancement could be observed in DMF upon the addition of Cu(II), indicating the relatively significant solvent effect in the sensing system. The effect of each component in the sensing system was investigated via a series of control experiments. As shown in Fig. 1b, we found that no fluorescence enhancement was produced regardless of discarding any of the components. Moreover, under ultraviolet irradiation at 365 nm, blue fluorescence could be observed with the naked eye in the complete detection system (inset of Fig. 1b). To gain insight into the proposed reaction mechanism, we confirmed the structure of the final fluorescent product in the millimolar level detection reaction as salicylamide by employing 1H NMR and 13C NMR (Fig. S1 and S2†). The aforementioned results clearly demonstrate that the reaction between Cu(II) and 2-(aminocarbonyl)phenylboronic acid leads to the oxidative hydroxylation of the boronic acid moiety in the presence of Et3N, resulting in ESIPT fluorescence.
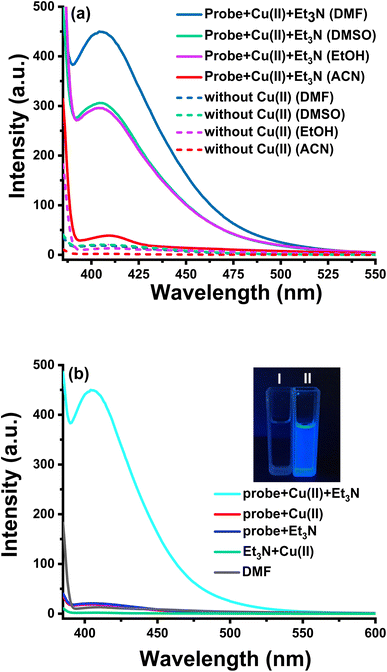 |
| Fig. 1 (a) Fluorescence emission spectra (λex = 365 nm) of the sensing system containing 100 μM 2-(aminocarbonyl)phenylboronic acid, 50 μM Et3N, with or without 50 μM Cu(II) in indicated solvents and (b) the emission spectra of the control experiments carried out in DMF under various conditions. Inset: photographs of 1 mM of 2-(aminocarbonyl)phenylboronic acid without Cu2+ (I) and with 0.5 mM of Cu2+ (II) under 365 nm UV illumination. | |
Alkaline conditions proved to be favorable for the Cu(II)-catalyzed nucleophilic substitution of boronic acid.40–42 The effect of the triethylamine concentration on the assay system was then examined. As shown in Fig. S3,† the fluorescence intensity at 410 nm dramatically increased along with the increasing concentration of Et3N (0–210 μM), and fluorescent intensity enhanced up to 13 times. Thus, the suitable concentration of triethylamine was chosen as 150 μM. For further investigation, we optimized detection conditions, such as the H2O/DMF ratio and incubation time. It can be found that the fluorescence intensity decreased when elevating the ratio of H2O/DMF and achieved the highest enhancement with the diluting solution as pure DMF at room temperature (25 °C) (Fig. S4†). Because the stock solution of Cu(II) was prepared in deionized water, the test solution contained a smidgen of water.
Incubation time is an important indicator of the response capability of the probe, so we examined the alteration of fluorescence at various incubation times. As shown in Fig. 2, no obvious fluorescence enhancement occurred in the absence of Cu(II), exhibiting the excellent stability of the probe in this system. After adding Cu(II), the fluorescence intensity of the sensing system increased gradually with an extended incubation time, reaching a steady state at 2.5 h. Consequently, we chose 2.5 h as the best incubation time for subsequent experiments.
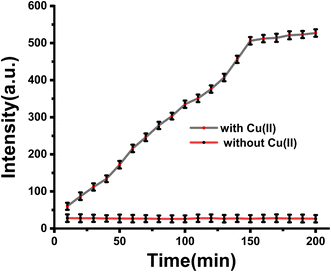 |
| Fig. 2 The fluorescence intensity of the system in the presence and absence of Cu(II) at the indicated time. The detection concentration is 100 μM for 2-(aminocarbonyl)phenyl boronic acid, 150 μM for triethylamine and 50 μM for Cu(II) in DMF. The fluorescence was monitored at 10 min intervals and collected at a wavelength of 410 nm. Each data point represents the average of the three trials. Error bars were calculated as standard deviations. | |
To test sensitivity, fluorescence spectra with different concentrations of Cu(II) were studied. When the concentration of Cu(II) was increased from 0 to 96 μM, the fluorescence emission intensity increased significantly and obtained the highest 35-fold fluorescence enhancement (Fig. 3a). A linear equation of F410 nm = 15.196 + 26.874 [Cu2+] (R2 = 0.9964) was obtained at a concentration range of 0–22 μM Cu(II), with a limit of detection (LOD) calculated as low as 68 nM based on LOD = 3σ/k (where σ is the standard deviation of blank measurement and k is the slope between the fluorescence intensity versus Cu(II) concentration) (Fig. 3b). As shown in Table S1,† the proposed assay is comparable to other previously reported fluorescence probes for monitoring Cu(II) (see Table S1† for details) and is much lower than the limit of Cu in drinking water (∼20 μM) set by the U.S. Environmental Protection Agency.43
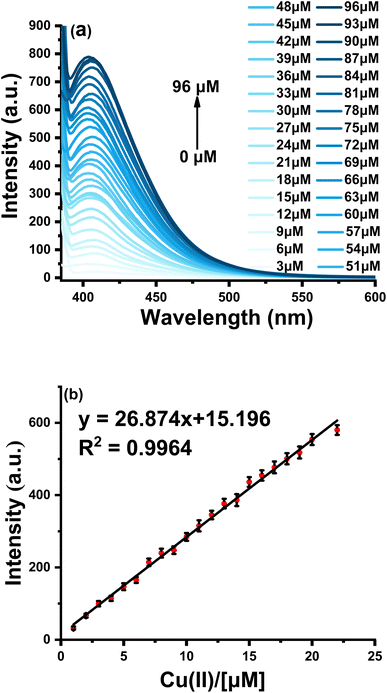 |
| Fig. 3 (a) Fluorescence responses of the sensing system to serial concentrations of Cu(II). The detection concentration is 100 μM for 2-(aminocarbonyl)phenyl boronic acid, 150 μM for triethylamine and the concentration of Cu(II) is in the range of 0–96 μM. (b) The linear correlations between fluorescence intensity and the concentration of Cu(II) (0–22 μM), λex = 365 nm. | |
Furthermore, we summarized the studies of Cu(II) sensors on applicability and analytical methods in LOD and linear range, as illustrated in Table 1. Compared with the reported results, our study exhibits higher sensitivity, lower detection limit, and wider linear range. Particularly, our analytical method showed some impressive advantages, such as operational simplicity, convenience and low cost.
Table 1 Comparison of the proposed method for Cu(II) detection with other previously reported assays
Entry |
Analytical method |
Reagents/detection technique |
Linear range (μM) |
LOD (μM) |
Ref. |
1 |
FAAS |
HNO3/deionized water |
— |
0.016 |
6 |
2 |
ICP-AES |
8-Hydroxyquinoline/C18 column |
— |
0.16 |
7 |
3 |
UV, NIR |
Carbon dots |
0.3–1.6 |
0.09 |
44 |
4 |
UV-vis absorption spectrum |
PNPs |
0–8 |
0.062 |
45 |
5 |
“Turn off” fluorescence probe |
NaClO4/reverse pulse polarography |
0–1 |
0.0467 |
46 |
6 |
Fluorescence probe |
AIE dots |
0.025–6 |
0.1 |
47 |
7 |
“Turn-on” fluorescent probe |
2-(Aminocarbonyl)phenylboronic acid |
0–22 |
0.068 |
This work |
High selectivity to detect objects over potentially competing species is an important indicator of the anti-interference capability of the probe. Competitive experiments on Cu(II) were carried out to evaluate the selectivity of the probe for Cu(II). As demonstrated in Fig. 4, only Cu(II) resulted in a remarkable fluorescence enhancement at 410 nm, whereas a range of common metal ions, including alkali and alkali-earth metal ions (K+, Na+, Ca2+, Mg2+, Sr2+), transition metal ions (Ag+, Zn2+, Mn2+, Co2+, Pd2+, Hg2+, Fe3+, Cr3+, Al3+) and rare-earth metal ions (Pr3+, Ce4+) merely caused slight enhancement in the fluorescence, even when K+, Na+, Mg2+ and Ca2+ were added at micromolar levels (Fig. 4). The results demonstrate the specific oxidative hydroxylation of the arylboronic acid catalyzed by Cu(II) and indicate the capability of the probe in the selective detection of Cu(II).
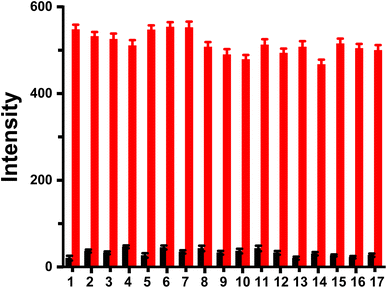 |
| Fig. 4 Fluorescence intensity changes of probe (100 μM) upon the addition of common metal ions: 1, none; 2, K+ (2 mM); 3, Na+ (2 mM); 4, Ca2+ (1 mM); 5, Mg2+ (1 mM); 6, Sr2+ (50 μM); 7, Ag+ (50 μM); 8, Zn2+ (50 μM); 9, Mn2+ (50 μM); 10, Co2+ (50 μM); 11, Pd2+ (50 μM); 12, Hg2+ (50 μM); 13, Fe3+ (50 μM); 14, Cr3+ (50 μM); 15, Al3+ (50 μM); 16, Pr3+ (50 μM); and 17, Ce4+ (50 μM). Black bars: probe treated with marked metal ions in the absence of Cu(II). Red bars: probe treated with the marked metal ions, followed by Cu(II) (50 μM). | |
Finally, a sensing system was applied to detect Cu(II) in real water samples. Tap water, Ganjiang river water and rainwater were collected and pretreated based on previous studies.48,49 When exogenous Cu(II) (20 μM) was added to these samples, the fluorescence intensity increased significantly. As shown in Table 2, the recovery rates are 94.50% and 95.50% with relative standard deviations of 0.93% and 0.76% for tap water and rainwater, respectively. These results suggest that the fluorescence probe has potential applicability for Cu(II) monitoring in real water samples.
Table 2 Study of the detection of Cu(II) in real water samplesa
Samples |
pH |
Added amount (μM) |
Found amount (μM) |
Std dev. (μM) |
RSD (%, n = 3) |
Recovery (%, n = 3) |
Experimental condition: Cu(II) (20 μM), the concentrations of 2-(aminocarbonyl)phenyl boronic acid (100 μM) and triethylamine (150 μM); incubation time: 2.5 h. The pH values of the water samples were determined using a pH meter. |
Tap water |
6.42 |
20.00 |
18.90 |
0.36 |
0.93 |
94.50 |
Ganjiang river water |
7.82 |
20.00 |
17.80 |
0.57 |
1.08 |
89.00 |
Rainwater |
5.83 |
20.00 |
19.10 |
0.33 |
0.76 |
95.50 |
4. Conclusions
In this study, we reported a simple structure of arylboronic acid as a “turn-on” fluorescence probe for the detection of Cu(II). The recognition mechanism is based on the Cu(II)-catalyzed oxidative hydroxylation of the arylboronic acid moiety to phenol, leading to the recovery of the ESIPT effect and switching on the fluorescent. The probe exhibited excellent sensitivity (detection limit of 68 nM) and high selectivity for Cu(II) over 16 other metal ions. The probe is also reliable for determining exogenous Cu(II) in real water samples from Ganzhou, demonstrating its potential applicability for the detection of Cu(II) in real-world scenarios.
Conflicts of interest
The authors declare that they have no known competing financial interests or personal relationships that could have influenced the work reported in this paper.
Acknowledgements
Financial support from the Jiangxi Provincial Natural Science Foundation (20202BABL213007, 20212BAB203013), Jiangxi Provincial Key Laboratory of Functional Molecular Materials Chemistry (20212BCD42018), National College Students' Innovation and Entrepreneurship Training Program (202110407006), the Youth Jinggang Scholars Program in Jiangxi Province is gratefully acknowledged.
References
- K. J. Barnham and A. I. Bush, Chem. Soc. Rev., 2014, 43, 6727 RSC.
- E. Gaggelli, H. Kozlowski, D. Valensin and G. Valensin, Chem. Rev., 2006, 106, 1995 CrossRef CAS PubMed.
- A. Lamboux, E. Couchonnal-Bedoya, O. Guillaud, C. Laurencin, L. Lion-François, A. Belmalih, E. Mintz, V. Brun, M. Bost, A. Lachaux and V. Balter, Metallomics, 2020, 12, 1781 CrossRef CAS.
- C. S. Yang, W. L. Lu and K. H. Lu, Chin. J. Anal. Chem., 2000, 28, 68 CAS.
- H. B. Zengin and R. Gürkan, Talanta, 2021, 224, 121789 CrossRef CAS.
- Y. R. Hou, J. L. Lu, Y. C. Fan, K. Abudusalamu, X.-D. Tang, Q. Q. Wei, J.-K. Guo and W. Zhao, Spectrosc. Spectral Anal., 2022, 42, 2102 Search PubMed.
- G. Abbasse, B. Ouddane and J. C. Fischer, J. Anal. At. Spectrom., 2002, 17, 1354 RSC.
- T. A. Ali, A. A. Abd-Elaal and G. G. Mohamed, Microchem. J., 2021, 160, 105693 CrossRef.
- M. Amjadi and Z. Abolghasemi-Fakhri, Sens. Actuators, B, 2018, 257, 629 CrossRef CAS.
- D. Udhayakumari, S. Naha and S. Velmathi, Anal. Methods, 2017, 9, 552 RSC.
- J. Zhao, Y. Y. Wang, W. L. Chen, G. S. Hao, J. P. Sun, Q. F. Shi, F. Tian and R. T. Ma, RSC Adv., 2022, 12, 3073 RSC.
- J. G. Huang, M. Liu, X. Q. Ma, Q. Dong, B. Ye, W. Wang and W. B. Zeng, RSC Adv., 2014, 4, 22964 RSC.
- Z. P. Li, Y. W. Zhang, H. Xia, Y. Mu and X. M. Liu, Chem. Commun., 2016, 52, 6613 RSC.
- L. Q. Li, M. H. Zheng, X. Y. Yan, H. Huang, S. X. Cao, K. M. Liu and J.-B. Liu, J. Photochem. Photobiol., A, 2022, 432, 114096 CrossRef.
- Q. X. Ye, S. F. Ren, H. Huang, G. G. Duan, K. M. Liu and J. B. Liu, ACS Omega, 2020, 5, 20698 CrossRef CAS PubMed.
- W. H. Wang, T.-L. Yung, S. S. Cheng, F. Chen, J. B. Liu, C.-H. Leung and D.-L. Ma, Sens. Actuators, B, 2020, 132, 128486 CrossRef.
- D. J. Zhu, Y. H. Luo, X. W. Yan, W. Xie, W. Cai and X. Zhong, RSC Adv., 2016, 6, 87110 RSC.
- R. B. An, D. T. Zhang, Y. Chen and Y. Z. Cui, Sens. Actuators, B, 2016, 222, 48 CrossRef CAS.
- M. Tian, H. He, B. B. Wang, X. Wang, Y. Liu and F. L. Jiang, Dyes Pigm., 2019, 165, 383 CrossRef CAS.
- K. Y. Liu, H. M. Shang, F. F. Meng, Y. Liu and W. Y. Lin, Talanta, 2016, 147, 193 CrossRef CAS PubMed.
- M. Taki, S. Iyoshi, A. Ojida, I. Hamachi and Y. Yamamoto, J. Am. Chem. Soc., 2010, 132, 5938 CrossRef CAS PubMed.
- Z. H. Shi, X. L. Tang, X. Y. Zhou, J. Cheng, Q. X. Han, J. A. Zhou, B. Wang, Y. F. Yang, W. S. Liu and D. C. Bai, Inorg. Chem., 2013, 52, 12668 CrossRef CAS.
- K. K. Yu, K. Li, J. T. Hou and X. Q. Yu, Tetrahedron Lett., 2013, 54, 5771 CrossRef CAS.
- X. M. Wu, Z. Q. Guo, Y. Z. Wu, S. Q. Zhu, T. D. James and W. H. Zhu, ACS Appl. Mater. Interfaces, 2013, 5, 12215 CrossRef CAS.
- Y. K. Jang, U. C. Nam, H. L. Kwon, I. H. Hwang and C. Kim, Dyes Pigm., 2013, 99, 6 CrossRef CAS.
- J. B. Li, Y. Zeng, Q. H. Hu, X. L. Yu, J. Guo and Z. Q. Pan, Dalton Trans., 2012, 41, 3623 RSC.
- Q. Y. Wu and E. V. Anslyn, J. Am. Chem. Soc., 2004, 126, 14682 CrossRef CAS.
- Z. J. Hu, J. W. Hu, Y. Cui, G. N. Wang, X. J. Zhang, K. Uvdalb and H. W. Gao, J. Mater. Chem. B, 2014, 2, 4467 RSC.
- Z. Zhang, Y. Zou and C. Q. Deng, RSC Adv., 2017, 7, 14742 RSC.
- K. M. K. Swamy, S.-K. Ko, S. K. Kwon, H. N. Lee, C. Mao, J.-M. Kim, K.-H. Lee, J. Kim, I. Shin and J. Yoon, Chem. Commun., 2008, 5915 RSC.
- J. M. V. Ngororabanga, C. Moyo, E. Hosten, N. Mama and Z. R. Tshentu, Anal. Methods, 2019, 11, 3857 RSC.
- M. Taki, K. Akaoka, K. Mitsui and Y. Yamamoto, Org. Biomol. Chem., 2014, 12, 4999 RSC.
- D. Maity, A. Raj, D. Karthigeyan, T. K. Kundu and T. Govindaraju, RSC Adv., 2013, 3, 16788 RSC.
- M. Y. Pei, H. H. Kong, A. Q. Tian, X. Liu, K. B. Zheng, Z. L. Ren and L. Wang, J. Mol. Struct., 2022, 1250, 131806 CrossRef CAS.
- R. A. Coburn, A. J. Batista, R. T. Evans and R. J. Genco, J. Med. Chem., 1981, 24, 1245 CrossRef CAS.
- J. M. Xu, J. Berastegui-Cabrera, H. Y. Chen, J. Pachón, J. Zhou and J. Sánchez-Céspedes, J. Med. Chem., 2020, 63, 3142 CrossRef CAS.
- A. Weller, Naturwissenschaften, 1955, 42, 175 CrossRef CAS.
- T. Nishiya, S. Yamauchi, N. Hirota, M. Baba and I. Hanazaki, J. Phys. Chem., 1986, 90, 5730 CrossRef CAS.
- J. Catalan, F. Toribio and A. U. Acuna, J. Phys. Chem., 1982, 86, 303 CrossRef CAS.
- D. M. T. Chan, K. L. Monaco, R. H. Li, D. Bonne, C. G. Clark and P. Y. S. Lam, Tetrahedron Lett., 2003, 44, 3863 CrossRef CAS.
- J. M. Xu, X. Y. Wang, C. W. Shao, D. Y. Su, G. L. Cheng and Y. F. Hu, Org. Lett., 2010, 12, 1964 CrossRef CAS.
- A. A. Atia and M. Kimura, Catalysts, 2020, 10, 1262 CrossRef CAS.
- Office of Water U.S.
Environmental Protection Agency, Edition of the Drinking Water Standards and Health Advisories, U.S. EPA Office of Science and Technology, Washington, D.C., 2012, EPA 822-S-12 001 Search PubMed.
- A. Salinas-Castillo, M. Ariza-Avidad, C. Pritz, M. Camprubí-Robles, B. Fernández, M. J. Ruedas-Rama, A. Megia-Fernández, A. Lapresta-Fernández, F. Santoyo-Gonzalez, A. Schrott-Fischer and L. F. Capitan-Vallvey, Chem. Commun., 2013, 49, 1103 RSC.
- S. G. Liu, N. Li, Y. Z. Fan, N. B. Li and H. Q. Luo, Sens. Actuators, B, 2017, 243, 634 CrossRef CAS.
- G. Z. Huang, C. Li, X. T. Han, S. O. Aderinto, K. S. Shen, S. S. Mao and H. L. Wu, Luminescence, 2018, 33, 660 CrossRef CAS.
- R. Jiang, N. Liu, F. Li, W. S. Fu, Y. Zhou and Y. Zhang, Polymers, 2018, 10, 786 CrossRef.
- A. R. Jacobson, S. Dousset, F. Andreux and P. C. Baveye, Environ. Sci. Technol., 2007, 41, 6343 CrossRef CAS PubMed.
- Y. D. Wang, J. H. Li, Y. Gao, Y. Yang, Y. Gao and Z. F. Xu, Hydrometallurgy, 2020, 191, 105220 CrossRef CAS.
|
This journal is © The Royal Society of Chemistry 2022 |
Click here to see how this site uses Cookies. View our privacy policy here.