DOI:
10.1039/D2RA03947H
(Paper)
RSC Adv., 2022,
12, 26620-26629
A novel sensor for the selective monitoring of trace ytterbium ions using an agarose-based optical membrane
Received
27th June 2022
, Accepted 24th August 2022
First published on 20th September 2022
Abstract
A novel highly selective sensitive optical sensor was prepared via the chemical immobilization of β-2-hydroxybenzyl-5-bromo-2-hydroxyazastyrene (HBBHAS) on an epoxy-activated agarose membrane pieces. The absorbance variation of the immobilized azastyrene film on agarose upon the addition of 1.5 × 10−5 M aqueous solutions of La3+, Y3+, Al3+, Sc3+, Sm3+, Eu3+, Lu3+, Fe3+, Ce3+, Cr3+, S2O32−, Tb3+, Mn2+ and KIO3 revealed substantially higher changes for the Yb3+ ion compared to the other considered ions. Thus, using HBBHAS as an appropriate ionophore, a selective optical sensor for Yb3+ was prepared via its chemical immobilization on a transparent agarose membrane. The effects of pH, reagent concentration, and time duration of the reaction of immobilizing the reagent were examined. A distinct change in the maximum absorbance of the reagent was established on contact of the sensing membrane with Yb3+ ions at pH = 4.25. For the membrane sensor, a linear relationship was observed between the variation in membrane absorbance (ΔA) at 424 nm and Yb3+ concentrations in the range of 4.75 × 10−5 to 6.20 × 10−10 M with a detection limit of 1.9 × 10−10 M for Yb3+. The effects of some potentially interfering ions on the assessment of Yb3+ were analyzed, and no substantial interference was found. The sensor showed a short response time and decent durability with no reagent leaching. The recovery of Yb3+ ions from the sensor material was performed using 0.3 M HNO3 and its response was reversible and reproducible with RSD ≥ 1.95%. This study reports a non-toxic, economical, stable, accurate, easy-to-use, and novel optical sensor material to assess Yb3+ in synthetic and environmental water samples.
Introduction
Ytterbium, a soft silvery metal, is a member of the lanthanide family. Monazite, gadolinite and xenotime are minerals that contain it. This element is employed in some steels and is occasionally linked with yttrium or other related attributes. A mixture of seven stable isotopes makes up natural ytterbium. It has a beautiful silvery shine and is a soft, malleable, and ductile element. Ion-exchange and solvent methodologies advanced in the late 20th century have facilitated the separation of ytterbium from other rare earth elements. The techniques and applications in routine Yb determination remain limited. Since ytterbium is quite unstable, it must be maintained in sealed containers to avoid exposure to air and moisture. Ytterbium components can induce skin and eye irritation and are teratogenic. Although earlier investigations appear to show that the threat is minimal, all ytterbium compounds must be recognized as very poisonous. Metallic ytterbium dust is flammable and explosive. As Yb3+ is an industrially essential element and there are only a few sensors for this target, it is critical to establish a sensitive and selective method for assessing it.1–4
Although ytterbium has low toxicity, intravenous injections of its soluble salts induce liver and spleen damage. Ytterbium compounds are discharged into the environment in a variety of ways, mostly by the petroleum industry. They can also be emitted into the environment when domestic objects are discarded. Ytterbium progressively accumulates in soil and water, leading to increasing quantities in human and animal tissue and soil particles in the long run. Commercial fiber lasers and fiber amplifiers are built using it.5,6 In zero-expansion conductors, gallium, ytterbium, and germanium (YbGaGe) are applied as electrical components.7
Ytterbium and other lanthanide oxides are extensively employed in the manufacture of optical glasses, optical fibers, gasoline-cracking catalysts, polishing products, and carbon arcs as well as in the iron and steel industries to remove sulphur, carbon, and other electronegative components.8 Cathodic stripping voltammetry,9 luminescence spectroscopy,10 spectrophotometry,11,12 near-infrared spectroscopy,13,14 ICP-MS,15,16 HPLC-ICP-MS,17 thermal ionization mass spectrometry,18 ICP-AES,19–21 neutron activation analysis, isotope dilution mass spectrometry, X-ray fluorescence spectrometry,22–24 and electrothermal atomic absorption spectrometry25 are typical techniques for detecting Yb3+ ions in solutions at low levels. However, almost all of them are slow since they require various stages for sample preparation and are costly.
As a result, we are interested in developing a highly sensitive Yb3+ sensor that is both simple and rapid for analyzing Yb3+ ions in various samples. A variety of lanthanide group metal sensors have recently been reported.26–35
Optical sensors have shown to be quite useful for analyzing several metal ions.36–42 They are simple, quick, and affordable, and they can reliably respond to an extensive diversity of concentrations. According to a literature survey, there are just two reports on Yb3+ sensors. The first one was constructed on 2,5-bis(5-tert-butyl-benzoxazol-2-yl)thiophene (BBT) as a membrane carrier on PVC.43 This sensor has a reasonably narrow working range (10−6 to 10−2 M) and a micro-level detection limit. The second technique was established via the complexation of N,N-bis(salicylidene)1,2-bis(2-aminophenylthio)ethane embedded with Yb3+ onto mesoporous silica by a direct anchoring method for the adsorption and recovery of Yb3+ from aqueous samples.44
Owing to the crucial significance of Yb3+ in industry, the lack of good assessment techniques for this metal, and the urgent need for a sensitive Yb3+ sensor for the rapid monitoring of sub-micromolar Yb3+, a highly sensitive Yb3+ sensor for the estimation of Yb3+ ions in several samples is required.
Optical sensors have lately sparked a lot of attention because of their numerous applications in environmental analysis, process control, and clinical investigation.45 Immobilizing ionophores on transparent membranes is a fundamental step in the fabrication of optical sensors. Optical sensors are made with a variety of support materials, including sol–gel glass and plasticizers as well as lipophilic, ionic, hydrophilic, and molecularly imprinted polymers. Ionophores play a crucial role in optical sensor development. Because of their propensity to form stable complexes with metal ions, Schiff base ligands are broadly used as ionophores in membrane sensors. They possess great selectivity, stability, and sensitivity for a specified ion.46,47
Covalently immobilized dyes, unlike physically entrapped or immobilized dyes, suffer from hysteresis or leaching and have long lifetimes.48 Agarose, a non-toxic gel, is very hydrophilic in the pH range of 0.0–14. Our lab has been using transparent agarose membranes to facilitate the development of covalently immobilized optical sensors for monitoring pH. Agarose membranes can be easily created and activated by forming chemical connections with nucleophilic group-containing indicators.49–53
Spectrophotometric studies of complexes are preferred because of their low operating costs and simplicity. In our earlier reports, some optical sensors for Ag(I),36 Zn(II),37 Cr(III),38 and Co(II)39 evaluation in terms of covalent immobilization of coloured complexes in a suitable membrane were introduced. The goal of the present study is to successfully immobilize β-2-hydroxybenzyl-5-bromo-2-hydroxyazastyrene on an agarose membrane film to construct a novel optical sensor for Yb3+ determination. The sensor response time and pH value were optimized. The influence of several parameters such as pH, base matrix, and reagent concentration was optimized. The main objective of this article is to design a highly sensitive, selective, and stable Yb3+ sensor that can be used for the analysis of Yb3+ ions in real samples.
Experimental
Apparatus
A Jenway 3505 pH meter 9 V-AC power was employed to record the pH values. The Yb3+ ion evaluation was conducted by ICP-AES (PerkinElmer, Germany, 8300). Spectroscopic assessments were performed using a JASCO V-670 UV-Vis spectrophotometer. The thin films were positioned in a quartz cuvette and all absorption investigations were accomplished at 25 ± 2 °C in a batch mode.
Materials and devices
All reagents utilized in this investigation were of analytical grade. The Merck Chemical Company provided epichlorohydrin and other chemicals. Double-distilled water was used to prepare a CH3COOH/CH3COONa buffer test solution (0.05 M). By adding 0.5 M HCl or NaOH solutions dropwise, the pH was set.54 Synthesis and purification of the synthesized reagent (β-2-hydroxybenzyl-5-bromo-2-hydroxyazastyrene) were performed as per our previous report.55 To hold the agarose membranes within the spectrophotometer quartz cells for absorbance assessment, a home-made polyacrylamide holder was used.
Preparing and activating agarose membranes
By dissolving agarose powder in boiling water, a 10 mL solution of 5.0% (w/v) agarose was prepared. The hot solution was poured gently and pressed between two glass plates of 20 × 20 cm with a gap of 0.2 mm until it cooled down to 25 ± 2.0 °C. Then, the obtained transparent and thin membrane was cut into 3 × 1 cm sections and preserved under 50% methanol solution.49,50 The epichlorohydrin method was utilized for epoxy activation.51–53 After adding 0.8 mL 6.0% epichlorohydrin and 3.2 mL 2.0 M NaOH solutions to about 10 agarose membranes pieces, the solution in a beaker (25 mL) was diluted to 10 mL with water. In a water circulating bath, the blend was heated to 40 °C with agitation for 2.0 h. After cooling, the epoxy-activated membranes were completely rinsed with water of 4.0 °C using a glass filter. Then, the activated membranes were left for a longer time prior to immobilizing the reagent.49–53
Preparing the immobilized optical sensor
For immobilization, the agarose membrane was combined with a 5 × 10−3 M alkaline HBBHAS solution, as reported elsewhere. To remove any non-bound HBBHAS, the yellow membranes were fully washed with 50% methanol on a glass filter, soaked in methanol (50%) overnight, and rinsed vigorously with water. Consequently, the membranes were prepared and fixed by a polyacrylamide holder in a cell. Then, the cell was typically employed to assess the absorbance. All the assessments for the agarose membranes were done within an aqueous medium.
Measurement procedure
The sensor was positioned in a 1.0 cm quartz cell and a 3.0 mL acetate buffer solution (pH 4.25) containing a defined Yb3+ concentration was added. Its absorbance was estimated at 424 nm after 3.0 min. Detailed structural and morphological characterizations of the agarose-based optical membrane are presented in Scheme 1. The calibration curve was obtained by plotting the absorbance of a series of Yb3+ standard solutions at various concentrations. The Yb3+ concentration that existed in the sample was assessed using the calibration curve. The optode was regenerated for 5.0 min in a 0.3 M HNO3 solution and was ready to use. Scheme 1 presents both the immobilized optical sensor preparation and its measurement procedure.
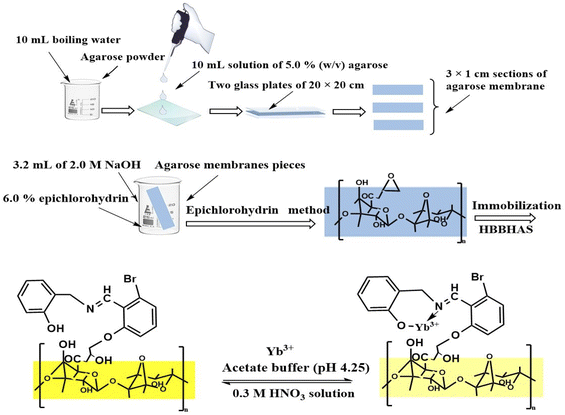 |
| Scheme 1 The immobilized optical sensor preparation and its measurement procedure. | |
Interferents
The influences of common coexisting ions on the recovery of Yb3+ were evaluated by spiking suitable quantities of the respective ions into solutions with 1 × 10−7 M Yb3+ ions, which was done according to a published approach after determining the optimal conditions. The tolerance limit was defined as the concentration of added ions that produced less than ±5.0% relative error. The Yb3+ recovery was almost the same in the presence of other species within the calculated tolerance limits.
Accuracy and precision
To establish the fabricated optode's precision and accuracy, solutions with three different concentrations of Yb3+ were prepared. The assay method was performed in six replicates, and the relative standard deviation (RSD) was calculated as a percentage within the same day to assess the repeatability (intra-assay) and over five days to estimate the intermediate precision (inter-assay).
Procedure for the ytterbium determination of samples
A column with the intensely basic anion exchange resin Dowex 1 × 8 in the Cl− form, 200 mesh, was applied. The resin was conditioned via successive washing with 1.0 M HCl, 1.0 M NaOH, 1.0 M HCl and 0.2 M EDTA disodium salt (Na2H2Y), until no Cl− ions could be detected in the effluent. The resin was placed in a column of 6.0 mm diameter and 7.0 cm height. An adequate aliquot of a rare earth mixture from the sample dissolution and later elimination of the matrix elements56 was evaporated to dryness and turned into respective anionic complexes with EDTA. It was dissolved at a volume of 200 μL 5 × 10−3 M Na2H2Y, pH = 4.25, and sowing was carried out to the top of the column. Elution was performed with 5 × 10−3 M Na2H2Y at a flow rate of 1.0 mL min−1.
Fractions of 1.5 mL were collected and analyzed after removing organic matter with an HCl/HNO3 mixture via an extractive absorptiometric procedure. In the current working conditions, Yb3+ is present in the first 37 mL of elution.
Determination of Yb(III) in soil and sediments
First, a sample (1.0 g) was weighed in a polytetrafluoroethylene (PTFE) beaker, followed by the addition of 5.0 mL 75% HClO4 and 10 mL 50% hydrofluoric acid (HF). Furthermore, the sample was heated to incipient dryness in a sand bath, and acid attack with HClO4 and HF (1 + 2) was performed three times to achieve silicate matrix digestion. The resulting samples were moved into flasks and diluted with 10 mL 2.5% NaOH and distilled water to 50 mL (pH ∼ 4.25). The next step was the evaluation of the ytterbium ion concentration in the samples using the absorbance variance of these solutions.
Results and discussion
HBBHAS immobilization over agarose membranes
Immobilizing HBBHAS on an agarose film altered its optical properties to some extent. A red shift was observed in the absorbance maximum of HBBHAS from 337 nm in acetonitrile solution to around 424 nm over the immobilized sample (Fig. 1), caused by the immobilized reagent's flatter structure compared to that of its soluble analog. To obtain sufficient reagent loading across the agarose membrane, the effect of the pH of the solution reaction on the maximum absorbance of the membrane was examined at 424 nm. The highest HBBHAS immobilization was attained within the pH range of 9.5–10.5. Investigations were conducted on the impacts of the HBBHAS concentration in the solution on the HBBHAS immobilization over the membrane. By increasing the HBBHAS concentration from 1 × 10−6 to 7 × 10−3 M, a steady increase in membrane absorbance could be observed. Any increase in load, conversely, might reduce the membrane's transmittance. Hence, the subsequent investigations were performed using the membrane sensors prepared in a HBBHAS concentration of 5 × 10−3 M with maximum absorbance.
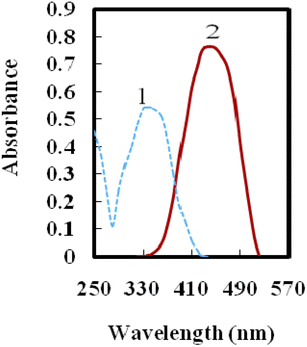 |
| Fig. 1 Curves 1 and 2 represent HBBHAS in acetonitrile solution (1.5 × 10−5 M) and after immobilization on an agarose membrane, respectively. In curve 2, a red shift was found after immobilization on the agarose membrane. | |
Optimization of the Yb3+ optical sensor
The influences of several parameters such as pH, response time, temperature, lifetime and regeneration were optimized to achieve highly selective determination.
Effect of pH on the sensor reaction
The fabricated membrane sensor's response characteristics were highly reliant on pH. Variation in pH altered the absorbance of the complexed and free species of the immobilized HBBHAS within the pH range of 3.0–11, which was used to evaluate the effects of pH absorbance variance (ΔA) prior to and after Yb3+ addition. Fig. 2 indicates that on altering the pH from 4.0 to about 4.5, the change in absorbance rapidly increased; conversely, at pH values greater than 6.5, it decreased. A pH of 4.25 was regarded as optimal and utilized for the test solutions. There is a possibility of the hydrolysis of Yb3+ in higher pH values.
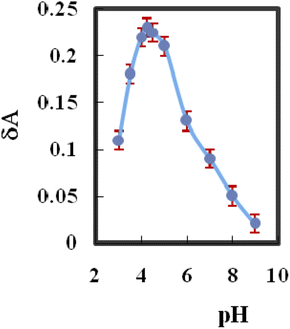 |
| Fig. 2 Effect of pH on the absorbance change between the complexed and free types of the immobilized HBBHAS (10−7 M, Yb3+). | |
The sensor response time
To calculate the Yb3+ sensor's response time, the absorbance was plotted as a function of time with 10−7 M Yb3+ concentration at 424 nm. As shown in Fig. 3, in about 60 s, the absorbance achieves a steady-state signal of 98%. Generally, the response time was reduced by increasing the analyte concentration, and it changed between 45 and 60 s. This could be attributed to the fact that by increasing the concentration of the analyte, its diffusion rate may be increased in the membrane phase.49,59,60
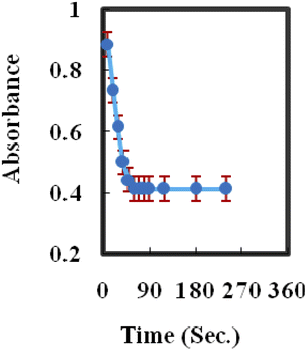 |
| Fig. 3 A characteristic response plot of the sensor at 424 nm as a function of time for [Yb3+] (10−7 M). | |
Effect of temperature on the sensor reaction
The influence of temperature on the sensing performance was investigated. The absorption spectra were recorded at different temperatures from 25 to 70 °C at 424 nm. As the temperature of the Yb3+ sample increases, the absorbance difference at 424 nm decreases due to thermal quenching related to the increase in the ion lattice vibration61 and decrease in the formation of the complex with the membrane. Increasing the temperature to ≥75 °C has no effect on absorbance, indicating that no complex formation occurs between Yb3+ and HBBHAS. The optimum temperature to achieve highly sensitive and selective results was 25 ± 2.0 °C.
The sensor regeneration
Multiple usage cycles of an optical sensor are desired, wherein the sensor is simply regenerated and gives reproducible responses. SCN−, EDTA, HNO3 and HCl solutions with different concentrations were employed to regenerate the membrane sensor and desorb Yb3+ from it. A 0.3 M HNO3 solution was used to efficiently remove any adsorbed Yb3+ from the membrane, returning its absorbance (ΔA ∼ 0) to its primary value in <2.0 min.
Lifetime
The membrane sensor's lifetime was examined by storing the membranes for two months in water.49 Before and after this period, the membranes' mean absorbances at 424 nm were 0.710 (±0.020) and 0.703 (±0.028), respectively, indicating the high stability of the obtained result. As a result, the membranes are found to stable for at least two months. Furthermore, no indication of signal drift or HBBHAS leaching was revealed across the membrane's many applications.
Preliminary studies
Absorbance variations following the absorbance readings at the maximum wavelength of immobilized HBBHAS on an agarose membrane in a spectrometer quartz cell were recorded in our preliminary investigations. They occur by adding 1.5 × 10−5 M aqueous solutions of Tl+, Zn2+, Al3+, Ca2+, Co2+, CrO42−, Ni2+, Cu2+, H3BO3, Mg2+, Na+, Pb2+, S2O32−, Sn2+, Mn2+, Ce3+, Ag+, Ba2+, Cd2+, Cr3+, Fe3+, La3+, Y3+, Al3+, Sc3+, Sm3+, Tb3+, Eu3+, Lu3+, Fe3+, Ce3+, Cr3+, S2O32−, Mn2+ and KIO3 followed by equilibration at pH 4.25. It is worth noting that the greatest variation is associated with Yb3+; nonetheless, minor changes in the maximum absorbance were detected (when studying the other ions) by increasing the concentration of the relevant ions. Due to the relatively high selectivity of HBBHAS for Yb3+ and based on its absorbance change, it was expected that the stated compound HBBHAS has a high selectivity for Yb3+.
It is possible that the immobilized HBBHAS had a flatter structural conformation than its soluble analog. To acquire further information regarding the conformational alterations in HBBHAS after complexing with the Yb3+ ion, the free HBBHAS molecular structure and that of its 1
:
1 complex with Yb3+ were enhanced using HyperChem 7.0 on a Pentium IV personal computer.57 The 6-31G* basis set was applied at the controlled Hartree–Fock (RHF) theory level to optimize the free ligand structure. Then, using the enhanced structure of HBBHAS, the structure of its 1
:
1 complex with Yb3+ was presented. Fig. 4 shows the optimal structures of the HBBHAS molecules as roughly planar; however, the structure was collected slightly with respect to the complex to provide the least conformational energy. The HBBHAS molecule acts as a bidentate ligand in the optimized complex structure and creates covalent and coordinate bonds with Yb3+ over its oxygen and one nitrogen donating groups, respectively. Considering the relatively high selectivity of the HBBHAS molecule for Yb3+ and spectrophotometry results, the biggest variation in absorbance was observed for Yb3+ compared to that for the other studied ions. Moreover, it is expected that HBBHAS acts as an appropriate sensing structure to fabricate an optical sensor for Yb3+ ions. The spectral features of this optical sensor reveal an absorbance maximum at 424 nm. It is found that by incrementing Yb3+ concentration, caused by the formation of the complex, the membrane absorbance at 424 nm is reduced. There was no detectable spectral change during the titration, which is characteristic of an absorption technique involving a robust complex establishment.58,59
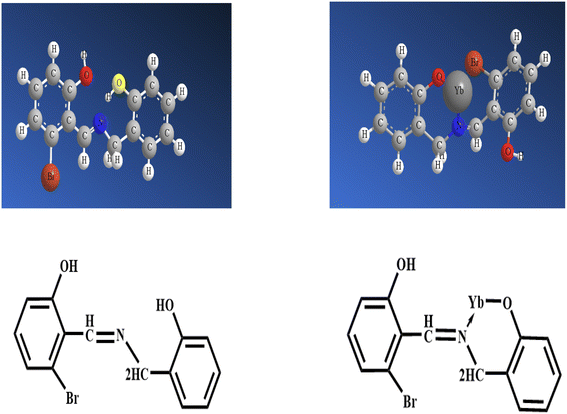 |
| Fig. 4 The optimized structures of the HBBHAS molecule and its Yb complex. | |
Selectivity
The developed sensor system's selectivity was examined in the presence of a series of different ions (cations and anions) under the optimized conditions. Interference was considered only when it caused a change in absorbance greater than ±5.0%. The impacts of several possibly interfering ions on the performance of the membrane sensor response were examined. All of the previously investigated ions, particularly lanthanide ions with concentrations at least 100 times that of the Yb3+ ion, were shown to have no significant influence on the analytical signal. The resultant relative errors are defined as RE (%) = [(A − Ao)/Ao] × 100. The selectivity of the obtained Yb3+ optical membrane sensor was investigated by recording the absorbance of Yb3+ ions at a fixed concentration (1.0 × 10−7 M; pH = 4.25) before (Ao) and after (A) adding some possibly interfering ions, at concentrations over 100 times that of the analyte ion. Based on the data, it is evident that the relative error is less than ±2.75% for all the considered metal ions, which is regarded as tolerable.49,59,60
Calibration curve of the sensor
Under the optimal conditions, to prepare the calibration curve for Yb3+, absorbance change values were plotted as a function of the analyte concentration at a time of 60 s, followed by the solution contact with the sensing phase. The film optode absorption signals were introduced to various Yb3+ concentrations in the range of 6.20 × 10−10 to 4.75 × 10−5 M. The calibration curve is linear up to the limit where the Yb3+ concentration is 6.20 × 10−5 M, with an R2 value of 0.9988 defined by the following equation: |
ΔA = −0.062pYb + 0.631
| (1) |
where pYb represents −log[Yb3+]. Based on the methodologies set by the International Union of Pure and Applied Chemistry (IUPAC), the detection and quantification limits were correspondingly defined as the sample concentration that afforded a signal identical to the blank signal and three times the standard deviation, which were 1.9 × 10−10 and 5.76 × 10−10 M, respectively, which are low enough to adequately monitor Yb3+ within environmental specimens.
Reproducibility and short-term stability
The reproducibility of the optode and its repeatability were demonstrated. To evaluate the optode reproducibility, six optodes were prepared, and solutions of 5.0 × 10−7 M and 2.0 × 10−6 M Yb3+ ions were measured seven times by the optodes. The RSD% values were calculated to be 2.1 (for 5.0 × 10−7 M) and 1.6 (for 2.0 × 10−6 M). To evaluate the repeatability of the optode, Yb3+ ion solutions with concentrations of 5.0 × 107 M and 2.0 × 10−6 M were measured seven times by the optode. The results show RSD% values of 2.25 (for 2.0 × 10−6 M) and 1.75 (for 5.0 × 10−7 M), respectively. In order to assess the short-term stability of the optical sensor, the membrane was placed in a sample solution containing 2.0 × 10−6 M Yb3+ and the absorption was assessed for 48 h (once every 4.0 hours, and repeating each measurement 5 times). The RSD% was equal to 1.45. This experiment reported that the optode had good stability and reproducibility.
The intra-day and inter-day precision and accuracy analytical results show that the present approach is highly repeatable and reproducible, with coefficients of variation ranging between 1.15% and 1.75%.
Analytical applications
The Yb3+ membrane sensor has been successfully applied in the spectrophotometric titration of Yb3+ (10 mL, 2.5 × 10−6 M) with a standard EDTA (1.0 × 10−4 M with pH 10) solution, and the results are provided in Fig. 5. As can be seen, the membrane sensor may be used to assess the amount of Yb3+ ions present.
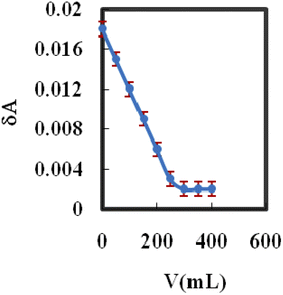 |
| Fig. 5 Spectrophotometric titration curve of 10 mL Yb3+ ion solution (2.5 × 10−6 M) with EDTA solution (1.0 × 10−2 M) using the proposed membrane sensor. | |
To demonstrate the usefulness of the proposed membrane, a set of samples comprising several natural water, soil and sediment samples containing different foreign ions were analyzed. Due to its high selectivity, the impurities in the samples tested have no effect. The ICP-AES data (Tables 1 and 2) reveal a respectable consistency within the assessed values using the introduced technique.19 The accuracy was assessed by comparing the results with those obtained using ICP-AES.19 Applying the paired t-test and F-test,62 no significant difference at the 95% confidence level was observed. The agarose membrane has a long lifetime and could be used several times to determine Yb3 ions in environmental water, soil and sediment samples.60
Table 1 Ytterbium determination in water samples (results based on triplicate measurements)
Sample |
Yb added M × 10−6 |
Proposed method |
ICP-AES19 |
t-Testb |
F-Testc |
Yb founda |
Recovery% |
Yb founda |
Recovery% |
Mean ± relative standard deviation (n = 6). Tabulated t-value for five degrees of freedom at P (0.95) is 2.57. Tabulated F-value at P (0.95) is 5.05. Gave no test for Yb3+. |
Tap waterd |
0.0 |
n.d. |
|
n.d. |
|
|
|
7.5 |
7.4 ± 0.31 |
98.67 |
7.7 ± 1.67 |
102.67 |
1.34 |
2.99 |
15 |
15.3 ± 0.65 |
102.00 |
14.5 ± 0.45 |
96.67 |
1.95 |
3.09 |
30 |
30.5 ± 0.38 |
101.67 |
29.4 ± 0.29 |
98.00 |
1.66 |
3.81 |
Ground waterd |
0.0 |
n.d. |
|
n.d. |
|
|
|
1.0 |
0.99 ± 0.33 |
99.00 |
1.02 ± 1.66 |
102.00 |
1.47 |
2.87 |
5.0 |
5.03 ± 0.65 |
100.60 |
4.85 ± 0.67 |
97.00 |
1.85 |
2.35 |
10 |
9.85 ± 0.41 |
98.50 |
10.30 ± 0.52 |
103.00 |
1.14 |
3.23 |
Sea waterd |
0.0 |
n.d. |
|
n.d. |
|
|
|
25 |
24.5 ± 0.32 |
102.08 |
26.9 ± 0.29 |
107.60 |
1.13 |
2.35 |
75 |
76.5 ± 0.48 |
102.00 |
74.2 ± 0.63 |
98.93 |
1.39 |
2.75 |
150 |
146.5 ± 0.66 |
97.67 |
158.5 ± 0.54 |
105.67 |
1.57 |
3.22 |
Lake waterd |
0.0 |
n.d. |
|
n.d. |
|
|
|
35 |
35.8 ± 0.57 |
102.29 |
34.6 ± 1.66 |
98.85 |
1.37 |
3.82 |
70 |
72.1 ± 0.31 |
103.00 |
67.4 ± 1.48 |
96.29 |
1.56 |
3.19 |
210 |
215.6 ± 0.38 |
102.67 |
202.0 ± 1.74 |
96.19 |
1.95 |
3.44 |
Polluted waterd |
0.0 |
n.d. |
|
n.d. |
|
|
|
20 |
20.5 ± 0.36 |
102.50 |
19.1 ± 1.84 |
95.50 |
1.95 |
3.54 |
80 |
82.2 ± 0.42 |
102.75 |
78.0 ± 1.43 |
97.50 |
1.76 |
3.85 |
160 |
162.2 ± 0.21 |
101.38 |
153.8 ± 1.29 |
96.13 |
1.81 |
2.68 |
Table 2 Ytterbium determination in soil samples (results based on triplicate measurements)
Samples |
Added ng g−1 |
Founda ng g−1 |
t-Testb |
F-Valuec |
Optode |
ICP-AES19 |
Average of six determinations. Tabulated t-value for five degrees of freedom at P (0.95) is 2.57. Tabulated F-value at P (0.95) is 5.05. |
Raase El-Bar soil |
|
42.8 ± 0.2 |
42.0 ± 0.6 |
|
|
50 |
93.3 ± 0.4 |
91.4 ± 0.9 |
1.27 |
|
100 |
141.7 ± 0.5 |
144.4 ± 0.8 |
|
3.56 |
150 |
194.5 ± 0.6 |
190.5 ± 0.7 |
1.49 |
|
Enshas soil |
|
56.2 ± 0.3 |
54.5 ± 0.9 |
|
|
40 |
95.6 ± 0.5 |
96.2 ± 1.0 |
|
3.81 |
80 |
138.2 ± 0.7 |
132.6 ± 1.1 |
1.05 |
|
120 |
178.7 ± 0.4 |
177.3 ± 1.0 |
|
2.87 |
Gamassa sediment |
|
68.6 ± 0.2 |
67.4 ± 1.4 |
|
|
60 |
130.0 ± 0.4 |
125.4 ± 0.9 |
|
3.63 |
120 |
185.8 ± 0.3 |
189.2 ± 0.8 |
1.24 |
|
180 |
252.2 ± 0.6 |
243.7 ± 1.2 |
|
2.78 |
To test the reliability of the proposed procedure, the proposed method was employed for the direct assessment of Yb3+ ions in binary mixtures, and the results are listed in Table 3. Defined concentrations of selected ions were mixed with 1 × 10−6 M Yb3+ ions and a buffer solution of pH 4.25 and added to the cell containing the sensor after each absorbance measurement. The recovery of Yb3+ ions is equivalent, as revealed in Table 3. The results obtained were compared with those obtained from ICP-AES11 analysis and were found to be in good agreement. The findings show that the Yb3+ membrane sensor can be used to monitor Yb3+ directly in real samples.
Table 3 Determination of Yb3+ ions in binary mixtures
Yb3+ (M) |
Mn+ (M) added |
Yb3+ (M) founda |
Recovery (%) |
Average of six determinations. |
1 × 10−6 |
Na+ (8 × 10−4) |
1.015 × 10−6 |
101.5 ± 0.4 |
K+ (5 × 10−4) |
0.984 × 10−6 |
98.4 ± 0.3 |
Ca2+ (4 × 10−3) |
1.022 × 10−6 |
102.2 ± 0.5 |
Ce3+ (6 × 10−4) |
1.016 × 10−6 |
101.6 ± 0.2 |
Th4+ (5 × 10−4) |
0.988 × 10−6 |
98.8 ± 0.4 |
La3+ (4 × 10−4) |
0.979 × 10−6 |
97.9 ± 0.5 |
Lu3+ (3 × 10−4) |
0.992 × 10−6 |
99.2 ± 0.4 |
Mg2+ (2 × 10−3) |
1.015 × 10−6 |
101.5 ± 0.3 |
Sm3+ (3 × 10−4) |
1.025 × 10−6 |
102.5 ± 0.3 |
Eu3+ (2 × 10−4) |
1.030 × 10−6 |
103.0 ± 0.2 |
Y3+ (1 × 10−4) |
0.975 × 10−6 |
97.5 ± 0.4 |
Co2+ (1 × 10−4) |
0.971 × 10−6 |
97.1 ± 0.2 |
The HBBHAS reagent introduces satisfactory sensitivity for the spectrophotometric assessment of rare earth ions, due to its great selectivity for Yb3+. Synthetic binary mixtures of Yb3+ and La3+ comprising various proportions of both substances were solved with excellent results (Table 3). This is due to the fact that the Yb3+ ion is selectively extracted with respect to La3+.
It is also possible to solve ternary mixtures of Yb3+, La3+ and Ce3+, with the results shown in Table 4. The lanthanide assessments in geological and other samples do not need a preceding separation step, even though determination techniques such as ICP63 are employed. These separation procedures are time-consuming processes. Methods such as that of Crock et al.56 based on ion-exchange chromatography by sequential acid elution can be applied for the initial separation of lanthanides from the matrix metals (this leads to a relative error of 3.0% in individual Yb assessment).
Table 4 Determination of Yb3+ in ternary mixtures
Sample |
% composition (w/w) molar relation |
Yb3+ founda |
% relative error |
Average of six determinations. |
1 |
Yb3+ |
33.3 |
32.6 (n = 6) |
|
La3+ |
33.3 |
s = 0.141 |
+2.0 |
Ce3+ |
33.3 |
CLα=0.05 = 32.6 ± 0.05 |
|
Yb3+ |
50.0 |
49.2 (n = 6) |
|
2 |
La3+ |
49.0 |
s = 0.023 |
−1.5 |
Ce3+ |
1.0 |
CLα=0.05 = 49.2 ± 0.02 |
|
3 |
Yb3+ |
5.0 |
4.7 (n = 6) |
|
La3+ |
45.0 |
s = 0.23 |
−5.2 |
Ce3+ |
50.0 |
CLα=0.05 = 4.7 ± 0.19 |
|
To solve multi-element lanthanide samples, ion-exchange chromatography was used. Mincxewsky and Dybcxynski64 examined the possibility of separating anionic complexes of rare earth elements with some organic ligands using anionic resins. This is a rapid and effective technique. According to their results, the elution order is Lu < Yb < Tm < Y < Ho < La < Ce < Tb < Pr < Gd < Pm < Eu.
Their experiment revealed that the separation of Yb3+ from La3+ is possible by the approach reported here (% relative error < 2.0%). Yb3+ was separated from Y3+ with an error of 3.0%. The elution order obtained by Mincxewsky was confirmed, which guarantees the quantitative separation of Yb3+ from the rest of the lanthanides. Table 5 presents the results obtained in the processing of synthetic samples and other materials.
Table 5 Determination of Yb3+ with HBBHAS in multi-element samples
Samples |
% composition (w/w) |
Yb(III) founda |
% Relative error |
Molar relation |
Synthetic samples. |
Yb3+ |
50.0 |
49.8 (n = 6) |
−0.40 |
I* other REE |
50.0 |
s = 0.032 |
|
|
CLα=0.05 = 49.8 ± 0.03 |
Yb3+ |
10.0 |
10.1 (n = 6) |
+1.00 |
2* other REE |
90.0 |
s = 0.136 |
|
|
CLα=0.05 = 10.1 ± 0.11 |
Yb3+ |
50.0 |
51.6 (a = 6) |
+3.20 |
3* other REE |
50.0 |
s = 0.20 |
|
|
CLα=0.05 = 51.6 ± 0.16 |
Magnet alloy |
25.0 |
24.9 (n = 6) |
−0.40 |
4* Other REE |
75.0 |
s = 0.070 |
|
|
CLα=0.05 = 25.0 ± 0.07 |
Perovskite57 5 Ba Na1/2 Yb1/2 Te O6 type Yb |
9.4 |
9.45 (n = 6) |
+0.53 |
s = 0.044 |
CLα=0.05 = 9.45 ± 0.045 |
Perovskite58 6 Ba, Yb Ru IrO9 type Yb |
16.9 |
17.1 (n = 6) |
+1.18 |
s = 0.136 |
CLα=0.05 = 17.1 ± 0.014 |
The performance of the proposed method was assessed by calculating the t-value (for accuracy) and F-value (for precision) compared with the ICP-AES method. The mean values were obtained using the Student's t- and F-tests at 95% confidence limits for five degrees of freedom.62 The results show that the calculated values did not exceed the theoretical values. The wider range of determination, higher accuracy, increased stability and lower time consumption show the advantages of the proposed method over the other method.
Conclusions
In summary, immobilizing β-2-hydroxybenzyl-5-bromo-2-hydroxyazastyrene on an agarose membrane led to an optical sensor with better selectivity to assess Yb3+. The introduced sensor provided desirable optical features, such as great selectivity, reproducibility, adequate lifetime, fast regeneration, easy and cheap fabrication, and easy handling. The membrane reacts to Yb3+ by reducing the sensing phase absorbance. Using a 0.3 M HNO3 solution, the sensor can be readily regenerated, resulting in a longer lifetime with multiple applications and making it an alternative technique for the environmental monitoring of Yb3+ over a range of 4.75 × 10−5 to 6.20 × 10−10 M. It was demonstrated that the data from ICP-AES11 were in accordance with the corresponding results of the proposed sensor.
Author contributions
Hesham El-Feky: conceptualization, data curation, investigation, methodology, visualization, validation, writing – original draft, writing – review & editing. Alaa Amin: conceptualization, methodology, data curation, investigation, supervision, validation, writing – original draft, writing – review & editing. Nader Hassan: conceptualization, investigation, methodology, validation, writing – original draft, writing – review & editing.
Conflicts of interest
The authors declare that they have no conflict of interest.
Acknowledgements
The authors would like to acknowledge the financial support from the Department of Chemistry, Faculty of Science, Benha University, and for providing instrumental facilities.
References
- M. R. Ganjali, L. Naji, T. Poursaberi, M. Shamsipur and S. Haghgood, Anal. Chim. Acta, 2003, 475, 59–66 CrossRef CAS.
- M. R. Ganjali, P. Norouzi, A. Tamaddon and M. Adib, Sens. Actuators, B, 2006, 114, 855–860 CrossRef CAS.
- H. A. Zamani, G. Rajabzadeh and M. R. Ganjali, Talanta, 2007, 72, 1093–1099 CrossRef CAS PubMed.
- A. K. Singh, A. K. Jain and S. Mehtab, Anal. Chim. Acta, 2007, 597, 322–330 CrossRef CAS.
- V. Gapontsev, IPG photonics debuts high-power fiber-pigtailed laser systems, Electro Manuf., 2002, 15, p. 3 Search PubMed.
- D. Woods, Netw. Comput., 2002, 13(17), 63–65 Search PubMed.
- M. Freemantle, Chem. Eng. News, 2003, 81, 6–15 CrossRef.
- O. R. Kirk and F. D. Othmer, Encyclopedia of Chemical Technology, Wiley, New York, 1982, vol. 19, p. 851 Search PubMed.
- M. Mlakar, Electroanalysis, 2003, 15, 27–32 CrossRef CAS.
- O. D. Stashkiv, V. O. Vasylechko, R. V. Gamernyk, G. V. Gryshchouk, A. V. Zelinskiy and L. B. Koliada, Mol. Cryst. Liq. Cryst., 2021, 719, 124–139 CrossRef CAS.
- A. Hrdlicka, J. Havel, C. Moreno and M. Valiente, Anal. Sci., 1991, 7, 925–929 CrossRef CAS.
- E. Kasprzycka, A. N. C. Neto, V. A. Trush, O. L. Malta, L. Jerzykiewicz, V. M. Amirkhanov, J. Legendziewicz and P. Gawryszewsk, Spectrochim. Acta, Part A, 2022, 274, 121072 CrossRef CAS PubMed.
- L. Wang, X. Yang, M. Yuan, Z. Yang, K. Han, H. Wang and X. Xu, Opt. Mater., 2022, 123, 111823 CrossRef CAS.
- A. D. Fedichkina, D. S. Koshelev, A. A. Vashchenko, L. O. Tcelykh, A. S. Goloveshkin, V. E. Gontcharenko, E. V. Latipov, A. V. Medvedko, S. Z. Vatsadze, A. S. Burlov and V. V. Utochnikova, J. Lumin., 2022, 244, 118702 CrossRef CAS.
- R. S. Houk, V. A. Fassel, G. D. Reach and H. J. Svec, Anal. Chem., 1980, 52, 2283–2289 CrossRef CAS.
- F. O. Leme, L. C. Lima, R. Papai, N. Akiba, B. L. Batista and I. Gaubeur, J. Anal. At. Spectrom., 2018, 33, 2000–2007 RSC.
- V. H. Nguyen, M. Ramzan, D. Kifle and G. Wibetoeb, J. Anal. At. Spectrom., 2020, 35, 2594–2599 RSC.
- Y. Mizutani, H. Hidaka and S. Yoneda, Geochem. J., 2020, 54, 381–391 CrossRef CAS.
- A. Mazzucotelli, F. De Paz, E. Magi and R. Frache, Anal. Sci., 1992, 8, 189–193 CrossRef CAS.
- B. Cai, B. Hu, H. Xiong, Z. Liao, L. Mao and Z. Jiang, Talanta, 2001, 55, 85–91 CrossRef CAS.
- H. Sereshti, M. Kermani, M. Karimi and S. Samadi, Clean: Soil, Air, Water, 2014, 42, 1089–1097 CAS.
- A. Masada, N. Nomura and T. Tanaka, Geochim. Cosmochim. Acta, 1973, 37, 239–248 CrossRef.
- S. F. Marsh, Anal. Chem., 1967, 39, 641–648 CrossRef CAS.
- D. H. Cornell, Pure Appl. Chem., 1993, 65, 2453–2464 CrossRef.
- E. C. Lima, F. J. Krug, J. A. Nobrega and A. R. A. Nogueira, Talanta, 1998, 47, 613–623 CrossRef CAS.
- H. A. Zamani, M. R. Ganjali, P. Norouzi, A. Tadjarodi and E. Shahsavani, Mater. Sci. Eng., C, 2008, 28, 1489–1494 CrossRef CAS.
- H. A. Zamani, M. R. Ganjali, P. Norouzi and S. Meghdadi, Anal. Lett., 2008, 41, 902–916 CrossRef CAS.
- H. A. Zamani, G. Rajabzadeh, M. R. Ganjali and P. Nourozi, Anal. Chim. Acta, 2007, 598, 51–57 CrossRef CAS PubMed.
- H. A. Zamani, G. Rajabzadeh and M. R. Ganjali, J. Braz. Chem. Soc., 2006, 17, 1297–1303 CAS.
- H. A. Zamani, M. R. Ganjali, P. Norouzi and M. Adib, Sens. Lett., 2007, 5, 522–527 CrossRef CAS.
- H. A. Zamani, M. R. Ganjali, P. Nourozi and S. Meghdadi, J. Appl. Electrochem., 2007, 37, 853–859 CrossRef CAS.
- H. Behmadi, H. A. Zamani, M. R. Ganjali and P. Norouzi, Electrochim. Acta, 2007, 53, 1870–1876 CrossRef CAS.
- H. A. Zamani and M. Mohaddeszadeh, Anal. Lett., 2008, 41, 2710–2726 CrossRef CAS.
- M. R. Ganjali, P. Norouzi, B. Akabri-Adergani, S. Riahi and B. Larijani, Anal. Lett., 2007, 40, 1923–1938 CrossRef CAS.
- M. R. Abedi, H. A. Zamani, M. R. Ganjali and P. Norouzi, Sens. Lett., 2007, 5, 516–521 CrossRef CAS.
- H. H. El-Feky, A. M. Askar and A. S. Amin, RSC Adv., 2021, 11, 35300–35310 RSC.
- A. S. Amin, S. El-Bahy and H. H. El-Feky, Anal. Biochem., 2022, 643, 114579 CrossRef CAS PubMed.
- H. H. El-Feky, S. M. El-Bahy, A. M. E. Hassan and A. S. Amin, Int. J. Environ. Anal. Chem., 2021, 1–18 CrossRef.
- H. H. El-Feky, A. S. Amin and E. M. I. Moustafa, RSC Adv., 2022, 12, 18431–18440 RSC.
- Z. Al-Mallah and A. S. Amin, J. Ind. Eng. Chem., 2018, 63, 281–287 CrossRef CAS.
- J. C. Munoz-Cesar, C. Torres-Torres, J. Moreno-Valenzuela, D. Torres-Torres, G. Urriolagoitia-Sosa and M. Trejo-Valdez, Meas. Sci. Technol., 2013, 24, 035603 CrossRef CAS.
- A. S. Amin, Sens. Actuators, B, 2015, 221, 1342–1347 CrossRef CAS.
- M. Ebrahimi and H. A. Zamani, Anal. Lett., 2009, 42, 1041–1055 CrossRef CAS.
- M. S. Salman, M. N. Hasan, K. T. Kubra and M. M. Hasan, Microchem. J., 2021, 162, 105868 CrossRef CAS.
- M. H. Wu, J. L. Lin, J. Wang, Z. Cui and Z. Cui, Biomed. Microdevices, 2009, 11, 265–273 CrossRef PubMed.
- S. Dong, M. Luo, G. Peng and W. Cheng, Sens. Actuators, B, 2008, 129, 94–98 CrossRef CAS.
- Y. Egawa, R. Hayashida and J. Anazi, Anal. Sci., 2006, 22, 1117–1119 CrossRef CAS.
- H. Hisamoto, Y. Manabe, H. Yanai, H. Tohma, T. Yamada and K. Suzuki, Anal. Chem., 1998, 70, 1255–1261 CrossRef CAS PubMed.
- P. Hashemi and M. M. Abolghasemi, Sens. Actuators, B, 2006, 115, 49–53 CrossRef CAS.
- R. Heydari, M. Hosseini, A. Amraei and A. Mohammadzadeh, Mater. Sci. Eng., C, 2016, 61, 333–337 CrossRef CAS PubMed.
- P. Hashemi, M. M. Abolghasemi, K. Alizadeh and R. Afzari Zarjani, Sens. Actuators, B, 2008, 129, 332–338 CrossRef CAS.
- P. Hashemi, M. Hosseini, K. Zargoosh and K. Alizadeh, Sens. Actuators, B, 2011, 153, 24–28 CrossRef CAS.
- K. Alizadeh, R. Parooi, P. Hashemi, B. Rezaei and M. R. Ganjali, J. Hazard. Mater., 2011, 186, 1794–1800 CrossRef CAS PubMed.
- J. A. Dean, Analytical Chemistry Handbook, McGraw-Hill, New York, 1995, pp. 14.30–14.34 Search PubMed.
- M. E. Moustafa, E. M. Mabrouk, H. A. Dessouki and A. S. Amin, Microchem. J., 1991, 44, 311–317 CrossRef CAS.
- J. C. Crock, F. E. Lichte, G. O. Riddle and C. L. Beech, Talanta, 1986, 33, 601–608 CrossRef CAS PubMed.
- Hyperchem, Release 7.0, Hypercube, Inc., Gainesville, 2002 Search PubMed.
- P. Hashemi, M. M. Abolghasemi, K. Alizadeh and A. Zarjani, Sens. Actuators, B, 2008, 129, 332–338 CrossRef CAS.
- K. Alizadeh, B. Rezaei and E. Khazaeli, Sens. Actuators, B, 2014, 193, 267–272 CrossRef CAS.
- K. Alizadeh, R. Parooi, P. Hashemi, B. Rezaei and M. R. Ganjali, J. Hazard. Mater., 2011, 186, 1794–1800 CrossRef CAS.
- K. Saidi, W. Chaabani and M. Dammak, RSC Adv., 2021, 11, 30926–30936 RSC.
- J. N. Miller and J. C. Miller, Statistics and Chemometrics for Analytical Chemistry, Prentice-Hall, London, 5th edn, 2005 Search PubMed.
- Z. Sulcek, I. Rubeska, V. Sixta and T. Pauket, At. Spectrosc., 1989, 10, 4–9 CAS.
- J. Mincxewsky and R. Dybcxynski, J. Chromatogr., 1962, 7, 98–103 CrossRef.
|
This journal is © The Royal Society of Chemistry 2022 |