DOI:
10.1039/D2RA03488C
(Paper)
RSC Adv., 2022,
12, 19232-19239
Experimental and computational approaches to study the chlorination mechanism of pentlandite with ammonium chloride
Received
6th June 2022
, Accepted 27th June 2022
First published on 1st July 2022
Abstract
Pentlandite (Fe4.5Ni4.5S8) is the primary source for the metallurgical production of nickel worldwide, however it usually coexists with copper sulfide in nature. To develop an efficient and green process for the separation and extraction of valuable metals from the nickel sulfide concentrate, herein we conducted experimental studies and density functional theory (DFT) calculations to elucidate the chlorination mechanism of pentlandite using ammonium chloride (NH4Cl). First, low-temperature chlorination roasting experiments with NH4Cl were performed in which pentlandite was successfully converted into the corresponding metal chlorides (FeCl2 and NiCl2). Then, the chlorination product was analyzed via energy dispersive spectrometry to reveal the elemental distribution at the cross-section. Results reveal that Fe atoms in pentlandite underwent preferential chlorination to form a chloride layer, whereas Ni atoms remained at the center of the grain. Furthermore, density functional theory calculations were performed to investigate the chlorination mechanism of pentlandite by exploring two possible pathways, involving the adsorption of oxygen (O2), ammonium chloride (NH4Cl) and chlorine (Cl2) on both the (001) and (010) surfaces of pentlandite. Considering that the chlorination of pentlandite was achieved in air atmosphere, we first consider the direct chlorination of pentlandite by NH4Cl in the presence of oxygen. Dissociative oxygen adsorption was found to promote the chlorination process by providing oxygen sites for the dissociation of HCl, which is decomposed from NH4Cl, eventually leading to the formation of H2O and FeCl2 species. Alternatively, the reaction between pentlandite and Cl2 was proved to be feasible thermodynamically.
1. Introduction
Pentlandite, as an important source of nickel, is often used to extract valuable metals for metallurgy. It is usually coexists with copper sulfide in nature and this is called the nickel sulfide concentrate.1–4 Pyrometallurgical and hydrometallurgical routes have been applied in the industry to extract nickel from the nickel sulfide concentrate.5 Preactivation roasting–water leaching has proven to be a promising technique for comprehensively recovering valuable metals from the nickel sulfide concentrate.6–8 Recently, chlorinating roasting, an activated method for chlorinating metals to form metal chlorides, has exhibited high selectivity because of the different thermodynamic stabilities of each metal chloride and has transformed the valuable components of the nickel sulfide concentrate into water-soluble salts.9–11 Thus, selecting an appropriate chlorinating agent is necessary to achieve the high-efficiency extraction of valuable metals. Conventional chlorinating agents include gaseous chloride (Cl2 and HCl) and solid chloride.12–16 Although Cl2 and HCl exhibit excellent chlorinating performances, the high requirements for equipment and potential hazards restrict their application. Recently, ammonium chloride (NH4Cl) has shown advantages in extracting valuable metals from raw materials, such as copper and nickel sulfide ores, at a considerably low temperature while remaining active after decomposition.17–19 NH4Cl reacts with metal sulfides to produce corresponding metal ammonium chloride salts. When the roasting temperature exceeds 320 °C, these ammonium salts will be further decomposed into the corresponding metal chlorides.20
To promote the separation of valuable metals with high efficiency, the chlorination mechanism between the nickel sulfide concentrate and NH4Cl needs to be investigated. Products obtained from the chlorination process have been extensively investigated using experimental methods, such as X-ray diffraction (XRD), scanning electron microscopy (SEM), and energy-dispersive spectrometry (EDS).21–24 Rashid et al. proposed a technique for iron and copper recovery from copper smelter slag via heat treatment with NH4Cl.21 Mu et al. proposed a novel two-stage chlorination roasting of Ni–Cu matte using an NH4Cl additive to obtain a high water-leaching rate of nonferrous metals and minimize the formation of the water-soluble Fe salt.23 Previously, we compared several important features of different chlorination agents, such as their reactivity, economic feasibility and corrosivity, and selected NH4Cl as an additive while confirming the feasibility of chlorination roasting when using the nickel sulfide concentrate.25 Generally, the chlorination roasting process has been deemed an efficient technique for separating valuable metals, but few theoretical studies have focused on elucidating the chlorination mechanism.
Density functional theory (DFT) calculations were applied to elucidate the reaction mechanism between gases and solid surfaces at atomic scale.26,27 Pentlandite being the primary source for nickel extraction, some computational studies have attempted to elucidate the electronic structure of pentlandite. Mkhonto et al. modeled the interaction of O2 with the (110) surface of pentlandite (Fe5Ni4S8).28 They found that the molecular adsorption of O2 at different surface sites was spontaneous, with the Fe-top site being more favorable over both face-centered-cubic hollow and Ni-top sites. Lu et al. studied metal distribution in pentlandite, demonstrating that the metal distribution at tetrahedral sites plays a major role in structural stability.29 Lu et al. further investigated the catalytic activity in hydrogen evolution reaction of pentlandite, involving the (001) and (100) surfaces of pentlandite.30 Additionally, Anasthasiya et al. investigated the interaction between NH3 and the PbS(100) surface through DFT calculations.31 The calculated results indicate that the adsorption of NH3 over PbS is energetically favorable.
We previously studied the oxidation mechanism of complex metal sulfides such as chalcopyrite (CuFeS2) and pentlandite (Fe4.5Ni4.5S8), and elucidated the oxygen migration mechanism and the preferential oxidation of the iron sites using both DFT calculations and experimental studies.32,33 In a related recent work, we investigated NiS as the intermediate product of Ni3S2 oxidation, indicating that O2 can promote the chlorination of NiS using NH4Cl and that the generated Cl2 can promote chlorination process, thus yielding the corresponding metal chloride.34 This work is aimed at elucidating the chlorination mechanism of pentlandite by NH4Cl through both theoretical and experimental studies, providing a mechanistic understanding of pentlandite chlorination.
2. Methods
2.1 Experimental methods
The raw materials, obtained from Jinchuan Group Ltd. (China), have been analyzed in our previous work via X-ray fluorescence spectrometry (XRF-1800, SHiMADZU LIMITED, Japan).35 The sample was dried at 100 °C for 24 h and ground into a fine powder (∼200 mesh) using an agate mortar. Mineralogical characterization was performed using a tungsten filament scanning electron microscope, and the elemental distribution was characterized via energy-dispersive spectroscopy (EDS) analysis using an SU-1510.
2.2 Computational methods
All calculations were performed using Vienna ab initio simulation package (VASP).36,37 The electrons are completely transferred via quantum mechanics through the Kohn–Sham equation. Using the generalized gradient approximation of energy, the energy cutoff value of plane wave expansion is determined to be 400 eV.38–40 The k-point-mesh in the full wedge of Brillouin zone is sampled using a 3 × 3 × 1 grid according to the Monkhorst–Pack grid.41 Spin-polarized calculations were performed throughout this work. Transition states were calculated using the climbing image nudge elastic band (CI-NEB) method.42 For each optimized steady state, we calculate the frequency to ensure the accuracy of the transition state. The density of states (DOS) was performed to explain the electronic structure properties of (001) and (010) surfaces of pentlandite. Bader charge analysis were also performed to investigate the electronic properties.43 The lattice parameters of the bulk of pentlandite obtained herein agree well with those reported in previous literature.44 The space group of pentlandite is Fm
m.44 The (001) and (010) surfaces of pentlandite were considered based on a previous report: minerals mainly cleave along surfaces with large inter-plane spacing during the flotation; these surfaces are usually low-index surfaces with low surface energies. Our slab models for the (001) and (010) surfaces of pentlandite both contain seven atomic layers with a vacuum spacing of 15 Å, thus avoiding interactions between two periodically repeated slabs. The energy of molecules were calculated using a 10 × 10 × 10 Å cubic cell. The bond length of molecular O2 calculated using our approach is 1.23 Å, which agrees well with the experimental value of 1.21 Å.45
3. Results and discussion
3.1 General discussion on the chlorination of pentlandite
Fig. 1 shows a schematic of the chlorination mechanism of pentlandite by NH4Cl studied both experimentally and via DFT calculations. First, the chlorination roasting experiment of pentlandite with NH4Cl was performed in air atmosphere. Besides, the chlorination of pentlandite involves the chlorination agent (NH4Cl), atmospheric oxygen (O2), and possibly the chlorine (Cl2) formed during chlorination. To reveal the chlorination mechanism of pentlandite through theoretical studies, two possible pathways were considered. In path 1, NH4Cl reacts with pentlandite directly in the presence of oxygen. |
Fe4.5Ni4.5S8 + NH4Cl + O2 → FeCl2 + NiCl2 + NH3 + H2S
| (1) |
 |
| Fig. 1 Schematic of the experimental and DFT studies. | |
In path 2, the Cl2 generated during chlorination process reacts with pentlandite.
|
Fe4.5Ni4.5S8 + Cl2 + O2 → FeCl2 + NiCl2 + SO2
| (2) |
Thus, theoretical work examines the two pathways.
3.2 Elemental distribution during pentlandite chlorination
The chlorination roasting of the nickel sulfide concentrate was achieved using NH4Cl. As shown in Fig. 2, pentlandite and chlorinate form the main phases in the sulfide concentrates. The Fe2O3 phase appeared after a roasting time of 30 min under air atmosphere at 300 °C, which can be attributed to the preferential oxidation of Fe from pentlandite. The detailed reaction mechanism has been discussed in our previous work.33 The appearance of the NH4FeCl3 phase can be attributed to the reaction between the Fe-based sulfides and NH4Cl.
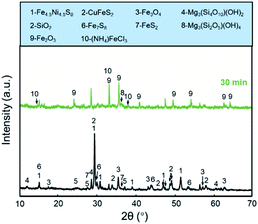 |
| Fig. 2 The XRD patterns for the nickel sulfide concentrate sample and the product obtained with NH4Cl at 300 °C for 30 min. | |
Fig. 3 shows the EDS-mapping results for Fe, Ni, O, Cl and S elements of the cross-section of a pentlandite particle after chlorination with NH4Cl at 300 °C. Fe and Cl were mainly found in the shell of the sample with Ni and S located in the core region. This suggests that Fe atoms in pentlandite were preferentially chloridized to form a chloride layer. Hence, combing the XRD and EDS results of the chloridized pentlandite grains reveals that the Fe species were chloridized to iron chloride before the chlorination of the Ni species.
 |
| Fig. 3 The EDS-mapping results for elements (Fe–Ni–Cl–S–O, Fe, Ni, Cl, S and O) of a pentlandite particle after chlorination roasting with NH4Cl at 300 °C in air for 30 min. | |
3.3 Chlorination mechanism of pentlandite with NH4Cl
Early experimental studies show that the chlorination of pentlandite by NH4Cl can only be achieved in the presence of molecular oxygen. Our calculations show that molecular NH3 arising from the sublimation of NH4Cl does not interact with the pentlandite surfaces. Therefore, it was predicted that NH4Cl would not directly interact with the pentlandite surfaces in the absence of oxygen. Thus, we considered the possibility of the pentlandite surfaces partial oxidation before its chlorination with NH4Cl.
3.3.1 The (001) surface. As shown in Fig. 4, oxygen atoms from dissociated O2 molecules were gradually added to the (001) surface of pentlandite. A monolayer (ML) coverage of adsorbates such as O on the surface is defined by the number of surface metal atoms. Here, the adsorption energy is also defined with respect to molecular O2: ½O2 (g) for atomic oxygen adsorption and O2 (g) for dissociation. The corresponding configurations are presented clearly in Fig. 4(a). Among the adsorption configurations, Fig. 4(i) and (ii) were mentioned in our previous work.33 In Fig. 4(iii), three oxygen atoms adsorb on the (001) surface with an adsorption energy of −8.79 eV. As there are eight surface metal atoms in the (001) surface of pentlandite, we considered oxygen-covered surfaces with up to four O atoms to attain a coverage of 0.5 ML with an adsorption energy of −11.09 eV (Fig. 4(iv)). In Fig. 4(b), the energies relative to molecular O2 in the gas phase were predicted to drop with increasing oxygen coverage and to follow a reasonably good linear relationship.
 |
| Fig. 4 (a) Top view for atomic oxygen adsorption on the (001) surface of pentlandite at different coverages and (b) correlation between the number of adsorbed O2 molecules and the adsorption energy (eV) on the (001) surface of pentlandite. ML signifies monolayer, which is defined by the number of surface metal atoms. | |
Based on the reaction between molecular O2 and the (001) surface of pentlandite, we further studied the reaction of pentlandite with NH4Cl. Fig. 5 shows the potential energy surface and the corresponding adsorption configurations. Among the adsorption configurations, Fig. 5(i) and (ii) were calculated in our previous work with molecular oxygen adsorbs on the Ni–Fe bridge site with an adsorption energy of −2.88 eV.33 Following O2 dissociation, NH4Cl is molecularly adsorbed on the (001) surface with an adsorption energy drop of ∼0.9 eV. Upon dissociation of the NH4Cl molecule on the (001) surface, O–H and Fe–Cl bonds are formed, whereas the NH3 molecule is adsorbed at the Fe top site. H2O is formed with added NH4Cl dissociation with a drop in energy by ∼1.01 eV, indicating that this process is thermodynamically favored. When H2O desorption from the surface, the energy increase for 0.29 eV, indicating that H2O desorption requires energy. After H2O desorption from the surface, a Cl atom can migrate to a neighboring Ni–Fe bridge site, forming an FeCl2 species. Finally, NH3 desorption also requires energy for ∼0.9 eV.
 |
| Fig. 5 (a) Potential energy profile and (b) corresponding adsorption configurations from side view and top view for NH4Cl adsorption on the (001) surface of pentlandite. | |
3.3.2 The (010) surface. For the (010) surface, on-surface adsorptions of O2 with coverages of 0.125, 0.25, 0.375 and 0.50 ML were studied. The coverages of 0.125 and 0.25 ML were discussed in our previous work, as shown in Fig. 6(i) and (ii).33 At 0.75 ML, an added oxygen atom can be adsorbed at the hollow site of Fe–Fe–Ni on the (010) surface, with the adsorption energy dropping to −5.99 eV. When oxygen coverage reaches 0.5 ML, the adsorption energy becomes more negative (−12.46 eV). The average adsorption energy of O2 decreases as the coverage increases from 0.125 to 0.5 ML. A similar trend was reported for oxygen adsorption on Pt and Pd surfaces.46–48 This indicates that the repulsive interaction between oxygen molecules builds up as coverage increases.
 |
| Fig. 6 (a) Top view for atomic oxygen adsorption on the (010) surface of pentlandite at different coverages and (b) correlation between the number of adsorbed O2 molecules and the adsorption energy (eV) on the (010) surface of pentlandite. | |
We further studied the interaction between NH4Cl and the (010) surface with adsorbed oxygen atoms. Fig. 7 presents the potential energy surface and the corresponding configurations. Among the configurations, Fig. 7(i) and (ii) were presented in our previous work.33 NH4Cl molecular adsorption drops by ∼0.9 eV compared with oxygen dissociative adsorption energy of −5.53 eV. The dissociation of NH4Cl leads to the adsorption being more negative, by ∼3.0 eV, with the formation of O–H and Fe–Cl bonds. H2O is formed with added NH4Cl dissociation with a drop in energy by ∼1.31 eV, indicating that this process is thermodynamically favored. After H2O desorption from the (010) surface, the relative energy increases by 0.53 eV, indicating that H2O desorption requires energy. NH3 desorption also requires an energy of ∼1.0 eV with the formation of an FeCl2 species. These results demonstrated that NH4Cl chlorination proceeds efficiently on the pentlandite surface when oxygen is preadsorbed in a dissociative manner.
 |
| Fig. 7 (a) Potential energy profile and (b) corresponding adsorption configurations for NH4Cl adsorption on the (010) surface of pentlandite from side view and top view. | |
3.4 Chlorination of pentlandite by the generated Cl2
3.4.1 The (001) surface. We first considered the adsorption of a single Cl atom on the (001) surface. As shown in Fig. 8, three stable adsorption configurations are located: the Ni hollow site, the Ni–Fe bridge site, and the Fe hollow site. The corresponding adsorption energies are −2.49, −2.95, and −2.72 eV, respectively, indicating that atomic chlorine adsorption at the Ni–Fe bridge site produces the most stable configuration, with the chlorine atom interacting with neighboring iron and nickel atoms. In practice, the adsorption configurations for the Cl atoms are similar to those for the O atoms, as both Cl and O atoms are more electronegative than S atoms. Pathway for atomic chlorine migration from the Ni hollow site to the Fe hollow site on the (001) surface is also shown in the figure. The energy barriers were calculated to be 0.78 and 0.45 eV, respectively, suggesting that the adsorbed Cl is easy to diffuse.
 |
| Fig. 8 (a) Potential energy profile and (b) corresponding adsorption configurations for the adsorption and diffusion of single chlorine atom on the (001) surface of pentlandite (top view). | |
Atomic chlorine adsorption energies at different coverages were investigated and the corresponding adsorption configurations were presented in Fig. 9. The adsorption energy decreases by ∼2 eV at a coverage of 0.25 ML. Furthermore, the adsorption energy drops to −8.11 eV because of the strong interaction between Cl atoms and the surface copper atoms when the coverage reaches 0.375 ML. When Cl coverage reaches 0.5 ML, the adsorption energy becomes more negative (−10.25 eV). A linear correlation was found between the number of adsorbed Cl2 molecules and the adsorption energy on the (001) surface of pentlandite (R2 = 0.99), indicating that there is almost no interaction between these adsorbates.
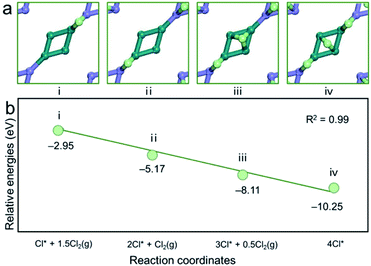 |
| Fig. 9 (a) Top view for atomic chlorine adsorption on the (001) surface of pentlandite at different coverages and (b) correlation between the number of adsorbed Cl2 molecules and the adsorption energy (eV) on the (001) surface of pentlandite. | |
3.4.2 The (010) surface. Atomic chlorine adsorption and diffusion on the (010) surface are presented in Fig. 10, where five different adsorption configurations were located. The most stable configuration is the Fe–Fe bridge site, where the chlorine atom interacts with two iron atoms with an adsorption energy of −3.04 eV. The chlorine atom can also adsorbed at the Ni–Fe bridge site with an adsorption energy of −3.02 eV, which approximates the Fe–Fe bridge site adsorption. The adsorption energies for the Fe hollow and Fe–Fe–Ni hollow sites are very similar, although the Fe hollow site is slightly more negative, by 0.01 eV, than the Fe–Fe–Ni hollow site. Thus, the chlorine atom prefers to interact with the iron atom over the nickel atom, leading to more stable adsorption configuration. Furthermore, the energy barriers for chlorine atom migration vary from 0.22 to 0.67 eV, indicating that chlorine atom is mobile on the (010) surface.
 |
| Fig. 10 (a) Potential energy profile and (b) corresponding adsorption configurations for the adsorption and diffusion of single chlorine atom on the (010) surface of pentlandite (top view). | |
As shown in Fig. 11, chlorine atoms from dissociated Cl2 molecules were gradually added to the (010) surface of pentlandite and the energies relative to molecular Cl2 in the gas phase were predicted to drop with increasing chlorine coverage. A reasonably good linear relationship is observed between these two quantities. The corresponding configurations are presented in Fig. 11(a). The adsorption energy drops by ∼3 eV at coverage of 0.25 ML. Furthermore, the adsorption energy drops to −8.84 eV because of the strong interaction between Cl atoms and the surface nickel atoms when the coverage reaches 0.375 ML. When Cl coverage reaches 0.50 ML, the adsorption energy becomes more negative (−11.08 eV).
 |
| Fig. 11 (a) Atomic chlorine adsorption on the (010) surface of pentlandite at different coverages and (b) correlation between the number of adsorbed Cl2 molecules and the adsorption energy (eV) on the (010) surface of pentlandite. | |
3.5 Electronic structure analysis
To provide further insight into the chlorination mechanism of pentlandite, we performed electronic structure analysis of the pentlandite surfaces. Total and partial DOS analyses of the (001) and (010) surfaces are presented in Fig. 12 and no band gap was predicted for both surfaces, suggesting that these surfaces have metallic character.
 |
| Fig. 12 Total and partial density of states for the (a) (001) and (b) (010) surfaces of pentlandite. | |
We further calculated the changes in Bader charges to examine possible electron transfer. As shown in Table 1, Bader charge analysis was performed for atomic chlorine adsorption on the (001) and (010) surfaces. Atomic chlorine was predicted carry more negative charges, suggesting that atomic chlorine serves as electron acceptor, leading to negatively charged. In addition, there is consistency between the amount of transferred charge and the adsorption energy.
Table 1 Changes in the Bader charges for atomic chlorine adsorption on the (001) and (010) surfaces
|
(001) |
(010) |
Ion |
Configuration |
Charge |
Configuration |
Charge |
Cl |
Fe hollow |
−0.51 |
Fe hollow |
−0.54 |
|
Ni hollow |
−0.50 |
Fe–Ni–Ni hollow |
−0.55 |
|
Ni–Fe bridge |
−0.56 |
Fe–Fe–Ni hollow |
−0.58 |
|
|
|
Fe–Fe bridge |
−0.54 |
|
|
|
Fe–Ni bridge |
−0.58 |
3.6 Discussion
An important issue for nickel extraction by chlorination is the separation of nickel chloride from other chlorides. Our EDS analysis reveals the distributions of Fe, Ni, O, S, and Cl after chlorination roasting and shows the preferential chlorination of Fe species in the chlorination roasting process. As pentlandite chlorination is a complex process, our calculations focused on the mechanism of the initial reactions of NH4Cl with the pentlandite surface to understand the preferential chlorination of the Fe species.
Based on our calculation results, the proposed chlorination mechanism of pentlandite with NH4Cl is shown in Fig. 13. First, oxygen adsorption on the two crystallographic planes of pentlandite was investigated by comparing molecular and dissociative adsorption at different adsorption sites in our previous work and at different surface coverages in this work.33 The computational results indicate that the interaction between O2 and pentlandite surfaces is favored thermodynamically and kinetically, revealing the significant role of molecular O2 during the chlorination roasting of pentlandite with NH4Cl. The preadsorbed oxygen atoms provides suitable sites for the dissociative adsorption of NH4Cl because of the formation of the considerably stronger O–H bond. This eventually leads to the formation of H2O on the partially oxidized pentlandite surfaces via hydrogen transfer. FeCl2 species is also generated because of the facile migration of Cl atoms.
 |
| Fig. 13 Schematic chlorination mechanism of pentlandite by NH4Cl. | |
In addition to the above discussed chlorination mechanism for pentlandite chlorination using NH4Cl, we investigated another possibility. We assume that molecular chlorine (Cl2), which was detected in the exhaust gas in some experimental studies, is formed during this process. The calculations for the reaction between Cl2 and pentlandite surfaces indicate that molecular Cl2 adsorption on both the (001) and (010) surfaces can be easily achieved. However, the interaction between Fe atoms of the pentlandite and chlorine is stronger than that of Ni atoms. The top layer of (010) surface contains more Fe atoms, and the adsorption energies are more negative than that of the (001) surface. Bader charge analysis further suggest that there is more charge transferred on the (010) surface. Therefore, the chlorination process of the (010) surface is more favorable than that of the (001) surface. Thus, our computational results are consistent with experimental results, enabling us to provide a mechanistic understanding of pentlandite chlorination.
4. Conclusions
In conclusion, our experimental results show that the Fe atoms migrate to the surface of pentlandite (Fe4.5Ni4.5S8) and are preferentially chloridized, forming the chloride layer. Our periodic DFT calculations on the chlorination of pentlandite reveal that two proposed pathways were feasible. First, the NH4Cl reacts with the pentlandite surfaces due to the presence of oxygen. The NH4Cl dissociative adsorption leads to the formation of FeCl2 species. Besides, the reaction between Fe4.5Ni4.5S8 and the generated Cl2 was favored thermodynamically, indicating that the chlorination can also be achieved by Cl2 adsorption. Considering the experimental studies and our computational results, two proposed mechanisms can contribute to the chlorination of pentlandite, and our studies provide important insights into the chlorination of pentlandite.
Conflicts of interest
There are no conflicts of interest to declare.
Acknowledgements
This work was jointly supported by Shanghai Sailing Program (No. 21YF1412900); the Shanghai Post-doctoral Excellence Program (No. 2021159); National Natural Science Foundation of China (No. 52004157); Steel Joint Research Foundation of National Natural Science Foundation of China – China Baowu Iron and Steel Group Co. Ltd. (No. U1860203); Shanghai Engineering Research Center of Green Remanufacture of Metal Parts (No. 19DZ2252900); National Basic Research Program of China (973 Program) (No. 2014CB643403); China Postdoctoral Science Foundation (No. 2019M661462) and CAS Interdisciplinary Innovation Team.
Notes and references
- V. Kirjavainen and K. Heiskanen, Miner. Eng., 2007, 20, 629–633 CrossRef CAS.
- S. J. Barnes, S. Staude, M. Le Vaillant, R. Pina and P. C. Lightfoot, Ore Geol. Rev., 2018, 101, 629–651 CrossRef.
- D. C. Peck and M. A. E. Huminicki, Ore Geol. Rev., 2016, 72, 269–298 CrossRef.
- X. Zeng, M. Xu and J. Li, Resour., Conserv. Recycl., 2018, 139, 188–193 CrossRef.
- R. Pandher and T. Utigard, Metall. Mater. Trans. B, 2010, 41, 780–789 CrossRef.
- W. Mu, F. Cui, Z. Huang, Y. Zhai, Q. Xu and S. Luo, J. Cleaner Prod., 2018, 177, 371–377 CrossRef CAS.
- D. Yu, T. A. Utigard and M. Barati, Metall. Mater. Trans. B, 2014, 45, 653–661 CrossRef CAS.
- G. Zhang, D. Luo, C. Deng, L. Lv, B. Liang and C. Li, J. Alloys Compd., 2018, 742, 504–511 CrossRef CAS.
- G. Zhu, R. Chi, W. Shi and Z. Xu, Miner. Eng., 2003, 16, 671–674 CrossRef CAS.
- F. J. Alvarez and A. E. Bohe, Ind. Eng. Chem. Res., 2008, 47, 8184–8191 CrossRef CAS.
- M. Zhang, G. Zhu, Y. Zhao and X. Feng, Hydrometallurgy, 2012, 129, 140–144 CrossRef.
- P. V. Aleksandrov, A. S. Medvedev, V. A. Imideev and D. O. Moskovskikh, Miner. Eng., 2019, 134, 37–53 CrossRef CAS.
- P. V. Aleksandrov, A. S. Medvedev, V. A. Imideev and D. O. Moskovskikh, Miner. Eng., 2019, 143, 106029–106035 CrossRef CAS.
- V. A. Imideev, P. V. Aleksandrov, A. S. Medvedev, O. V. Bazhenova and A. R. Khanapieva, Metallurgist, 2014, 58, 353–359 CrossRef CAS.
- M. Chakravortty and S. Srikanth, Thermochim. Acta, 2000, 362, 25–35 CrossRef CAS.
- F. Cui, W. Mu, Y. Zhai and X. Guo, Sep. Purif. Technol., 2020, 239, 116577–116590 CrossRef CAS.
- A. N. D'Yachenko and R. I. Kraidenko, Russ. J. Non-Ferrous Metals, 2010, 51, 377–381 CrossRef.
- A. A. Andreev, A. N. D’Yachenko and R. I. Kraidenko, Theor. Found. Chem. Eng., 2011, 45, 521–525 CrossRef CAS.
- P. Wu, L. Zhang, C. Lin, X. Xie, X. Yong, X. Wu, J. Zhou, H. Jia and P. Wei, Hydrometallurgy, 2020, 191, 105225–105235 CrossRef CAS.
- R. S. Zhu, J. H. Wang and M. C. Lin, J. Phys. Chem. C, 2007, 111, 13831–13838 CrossRef CAS.
- R. K. Nadirov, L. I. Syzdykova, A. K. Zhussupova and M. T. Usserbaev, Int. J. Miner. Process., 2013, 124, 145–149 CrossRef CAS.
- H. Zhu, J. Deng, J. Chen, R. Yu and X. Xing, J. Mater. Chem. A, 2014, 2, 3008–3014 RSC.
- F. Cui, W. Mu, S. Wang, H. Xin, H. Shen, Q. Xu, Y. Zhai and S. Luo, Sep. Purif. Technol., 2018, 195, 149–162 CrossRef CAS.
- X. Liu, Y. Feng, H. Li, Z. Yang and Z. Cai, Int. J. Miner., Metall. Mater., 2012, 19, 377–383 CrossRef CAS.
- C. Xu, H. Cheng, G. Li, C. Lu, X. Lu, X. Zou and Q. Xu, Int. J. Miner., Metall. Mater., 2017, 24, 377–385 CrossRef CAS.
- X. Chen, B. Yang, D. Tao and Y. Dai, Metall. Mater. Trans. B, 2010, 41, 137–145 CrossRef.
- H. Zhong, L. Wen, C. Zou, S. Zhang and C. Bai, Metall. Mater. Trans. B, 2015, 46, 2288–2295 CrossRef CAS.
- P. P. Mkhonto, H. R. Chauke and P. E. Ngoepe, Minerals, 2015, 5, 665–678 CrossRef CAS.
- L. Lu and S. Yu, Chem. Phys. Lett., 2019, 736, 136786–136793 CrossRef CAS.
- L. Lu and S. Yu, J. Colloid Interface Sci., 2021, 593, 116–124 CrossRef CAS PubMed.
- A. N. A. Anasthasiya, G. Kaur, T. V. Beatriceveena, V. Jayaraman and K. I. Gnanasekar, Appl. Surf. Sci., 2019, 495, 143605–143613 CrossRef.
- X. Xiong, X. Hua, Y. Zheng, X. Lu, S. Li, H. Cheng and Q. Xu, Appl. Surf. Sci., 2018, 427, 233–241 CrossRef CAS.
- X. Xiong, X. Lu, G. Li, H. Cheng, Q. Xu and S. Li, Phys. Chem. Chem. Phys., 2018, 20, 12791–12798 RSC.
- X. Xiong, G. Li, X. Lu, H. Cheng, Q. Xu and S. Li, Phys. Chem. Chem. Phys., 2020, 22, 4832–4839 RSC.
- G. Li, X. Zou, H. Cheng, S. Geng, X. Xiong, Q. Xu, Z. Zhou and X. Lu, Metall. Mater. Trans. B, 2020, 51, 2769–2784 CrossRef CAS.
- G. Kresse and J. Furthmüller, Comput. Mater. Sci., 1996, 6, 15–50 CrossRef CAS.
- G. Kresse and J. Furthmüller, Phys. Rev. B: Condens. Matter Mater. Phys., 1996, 54, 11169–11186 CrossRef CAS PubMed.
- G. Kresse and D. Joubert, Phys. Rev. B: Condens. Matter Mater. Phys., 1999, 59, 1758–1775 CrossRef CAS.
- J. Perdew, K. Burke and M. Ernzerhof, Phys. Rev. Lett., 1996, 77, 3865–3868 CrossRef CAS PubMed.
- P. E. Blochl, Phys. Rev. B: Condens. Matter Mater. Phys., 1994, 50, 17953–17979 CrossRef PubMed.
- H. J. Monkhorst and J. D. Pack, Phys. Rev. B: Solid State, 1976, 13, 5188–5192 CrossRef.
- G. Henkelman and H. Jónsson, J. Chem. Phys., 2000, 113, 9978–9985 CrossRef CAS.
- W. Tang, E. Sanville and G. Henkelman, J. Phys.: Condens. Matter, 2009, 21, 7 Search PubMed.
- V. T. Rajamani and C. T. Prewitt, Can. Mineral., 1973, 12, 178–187 Search PubMed.
- CRC Handbook of Chemistry and Physics, ed. D. R. Lide, CRC Press/Taylor and Francis, Boca Raton, FL, 2009 Search PubMed.
- Z. Gu and P. B. Balbuena, J. Phys. Chem. C, 2008, 112, 5057–5065 CrossRef CAS.
- W. Li, C. Stampfl and M. Scheffler, Phys. Rev. B: Condens. Matter Mater. Phys., 2002, 65, 075407–075426 CrossRef.
- M. Todorova, K. Reuter and M. Scheffler, J. Phys. Chem. B, 2004, 108, 14477–14483 CrossRef CAS.
|
This journal is © The Royal Society of Chemistry 2022 |
Click here to see how this site uses Cookies. View our privacy policy here.