DOI:
10.1039/D2RA02946D
(Paper)
RSC Adv., 2022,
12, 24607-24613
Functionalised electrospun membranes (TETA-PVC) for the removal of lead(II) from water†
Received
10th May 2022
, Accepted 16th August 2022
First published on 30th August 2022
Abstract
Driven by the need for delivering sustainable water purification solutions for the removal of heavy metals from water, electrospun PVC membranes were functionalised with triethylenetetramine (TETA) and were used to remove lead(II) ions selectively from water. The membranes were characterised and their adsorption behavior towards the removal of lead from water was investigated. The incorporation of TETA on the membrane's surface significantly improved the removal efficiency of lead(II) up to 99.8% in 30 minutes and under ambient conditions, with the lowest concentration of 50 ppm. The adsorption mechanism was investigated and kinetic data showed a better correlation with the pseudo-second-order model. Similarly, the equilibrium data best fitted with the Langmuir adsorption isotherm model with a relatively high maximum adsorption capacity of 1250 mg g−1 for lead(II) ions, larger than recently reported adsorption capacities for similar membranes. The functionalised membrane also showed high selectivity to lead(II) in a mixed solution containing lead(II), mercury(II), cadmium(II), arsenic(III), copper(II), and zinc(II). The functionalised membrane was regenerated, where desorption of lead(II) was achieved, under mildly acidic conditions. The removal efficiency of the regenerated membrane after six cycles of adsorption/desorption was maintained at a high level of 98%. The proposed design offers a simple yet effective, sustainable, and environmentally friendly solution for water treatment.
Introduction
The increased burden on the world's freshwater resources has severely impacted the social and economic well-being of many communities. This problem is likely to be exacerbated in the future due to projected population growth, climate change, urbanization, growth in irrigated agriculture, and economic development.1 Water pollution has been a major environmental concern worldwide limiting access to clean water. Toxic contaminants, particularly heavy metals, are transferred from various sources through runoff into water bodies, deteriorating the quality of available freshwater and threatening the aquatic and terrestrial environments.2–4 Heavy metals are among the hazardous pollutants, due to their high toxicity even at low concentrations,5 persistence in nature, and potential to bio-accumulate in food chains.6–8 Lead (Pb) is considered the second highest toxic heavy metal due to its persistence and non-biodegradability in nature.9 It is mostly generated from natural and anthropogenic sources such as batteries, paint, cosmetics, ceramic glazes, toys, plumbing systems, and pipes.10–12 Lead is abundantly found in nature possessing unique features, such as softness, malleability, ductility, low melting point, and resistance to corrosion, which resulted in its extensive use in different industries.13–15 However, exposure to lead is known to cause several health problems such as coma, cancer, renal failure, and could also damage the central nervous system leading to permanent cognitive impairment.12,16 The US Environmental Potential Agency (US EPA) indicated that the maximum allowable level of lead in drinking water is 15 μg L−1.16–18 Unfortunately, lead is still found at levels much higher than that in water bodies. Therefore, the efficient removal of lead from aqueous solutions is crucial for the protection of human health and the environment.
Various technologies have emerged for the removal of Pb(II) ions from water. However, the adsorption process is one of the most efficient methods with high and relatively better performance than the conventional water treatment methods, due to its simple operation, high adsorption capacity, wide applicability, and flexibility in the design.19,20 Different adsorbents, including activated carbon,21 zeolites,22 silica,23 and activated alumni24 have been reported for removing Pb(II) ions from water. The reported adsorbents showed high adsorption capacities, however, the high cost of some of the adsorbents, their relatively low efficiency, and low recyclability limit their use for water treatment.19,20 This implies the need to develop sustainable (green, low cost, and highly efficient) adsorbents. Membrane technology is regarded as a promising treatment method due to its high recovery rate of heavy metals from aqueous solutions,25 however, membrane fouling by which the solute blocks the pores of the membrane reduces its performance and weakens the designed system.26 To overcome this problem, altering the membrane's surface to enhance its adsorptive properties has been considered by introducing functional groups (carboxyl, amino, and thiol) on the surface.27–29 Among the employed functional groups, amine-functionalised membranes are highly recommended due to their interaction with aqueous heavy metals that may result in their efficient removal from water.30,31
Recently, researchers have shifted their interest to polymeric nanofibrous membranes for the removal of heavy metals from water.32–34 Nanofibrous membranes are known for their high surface area to volume ratio which makes them suitable candidates for extracting pollutants from aqueous solutions.35,36 To date, different fabrication techniques have been employed to produce polymeric nanofibrous membranes, with electrospinning as one of the most suitable methods for the production of continuous nanofibers.37–39 Surface modification strategies of fabricated membranes have been extensively explored to enhance their adsorption capacity and efficiency by altering the physical and/or chemical properties of the membrane.40
In this study, we are reporting the development of a functionalised electrospun membrane with a high adsorption capacity for aqueous Pb(II). Polyvinyl chloride (PVC) was employed for the fabrication of the membrane and the resulting PVC membrane was functionalised with triethylenetetramine (TETA). TETA is an organic polyamine ligand known to bind metal ions (such as copper,41,42 zinc, iron, and nickel),43 forming metal–organic complexes. Hence, the formation of a metal–organic complex between TETA and Pb(II) metal ions in water was investigated before modifying the surface of the PVC membrane. The proposed mechanism of the metal chelation is represented in Fig. 1 based on the literature.41 Moreover, XPS analyses proved the formation of covalent bonds between NH2 in TETA and lead(II) ions in water.44
 |
| Fig. 1 The proposed binding motif of TETA and Pb(II). | |
Surface modification of adsorbent (PVC) with TETA is a simple (one step) and scalable, yet effective approach to improve the performance of the adsorbent and achieve a higher adsorption capacity of lead(II) in water.30,39 This was confirmed in this study as indicated by the high adsorption capacity (qmax. 1250 mg g−1) of TETA-PVC towards Pb(II) ions relative to other reported studies as shown in Table 1.
Table 1 Comparison of maximum lead(II) adsorption capacity qmax (mg g−1) of TETA-PVC with previously reported functionalised electrospun membranes
Material |
Modification |
Adsorption capacity |
Ref. |
Polyacrylonitrile (PAN)/polyaniline (PANI)-nylon |
Diethylenetriamine (DETA) |
960 mg g−1 |
45 |
Chitosan/Polyvinyl alcohol (PVA)/polyether sulfone (PES) |
Fe3O4 nanoparticles |
525.8 mg g−1 |
46 |
Poly methacrylic acid (PMA)/cellulose acetate |
— |
146.2 mg g−1 |
47 |
Polyvinyl chloride (PVC) |
Triethylenetetramine (TETA) |
1250 mg g−1 |
This study |
Results and discussion
The formation of the mononuclear complex (TETA; Pb) was investigated in solution and was followed by modifying the PVC surface of the electrospun membrane with TETA ligand. The adsorption parameters of aqueous Pb(II) by the functionalised membrane were investigated and reported in this study.
Formation of TETA-lead(II) complex
UV-visible absorption study. TETA-Pb(II) complex formation in water was detected by UV-Vis Spectroscopy. The absorption spectra of the TETA, Pb(II), and their mixture in solution are presented in Fig. 2. A hypsochromic shift of the sharp peak of the TETA-Pb(II) spectrum from 208 to 194 nm along with a hyperchromic shift (an enhancement of the peak's absorbance at 194 nm) were observed relative to the uncomplexed Pb(II) spectrum. The change in the position and intensity of the absorption band indicates the formation of the metal–organic complex ([TETA/Pb(II)]).48 Additionally, a new peak at 250 nm was obtained consistent with the metal to ligand charge transfer. The formation of the two isosbestic points at 210 nm and 230 nm (see Fig. 2*) further confirms the formation of the metal–organic complex.49,50
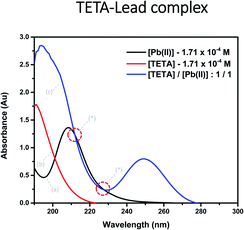 |
| Fig. 2 Absorption spectra of aqueous (a) lead(II) acetate trihydrate, (b) TETA, and (c) [TETA/Pb(II)] complex within the UV-visible region. | |
Binding mechanism of lead(II) ion and triethylenetetramine (TETA). A titration experiment of Pb(II) metal ion with TETA was carried out to investigate the binding association and stoichiometry of the resulting metal–organic complex in water. An aqueous solution of Pb(OAC)2 (1.71 × 10−4 M) in water was titrated with increasing concentrations of TETA ranging from 6.84 × 10−6 M to 1.71 × 10−4 M and was analysed by UV-Vis spectroscopy (Fig. 3a). Upon the addition of TETA to the lead solution, a maximum absorption peak was observed at a wavelength of 250 nm.
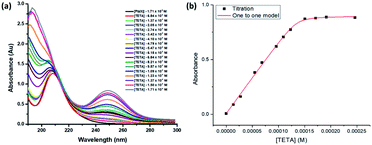 |
| Fig. 3 (a) UV-visible absorption spectra of 1.71 × 10−4 M solution of Pb(OAC)2 in H2O titrated with increasing concentrations of TETA, (b) binding isotherm of the complex formation of TETA–Pb(II) in water at a wavelength of 250 nm fitted to 1 : 1 binding model. | |
Furthermore, an isosbestic point is obtained at 218 nm, which shows that both species were at equilibrium at this wavelength,51 hence, indicating the formation of a metal–organic complex in solution.
The stoichiometry (metal: ligand) and the binding constants were calculated by fitting the experimental data, (obtained by the titration) to different stoichiometric equations. Fitting the experimental data with the 1
:
1 binding model was in good agreement (R2 = 0.998) (Fig. 3b). The binding constant (Ka) was found to be 1.15 ± 0.6 × 106 M−1, which is relatively high given that the study is done in water.52 This indicates the formation of a strong and stable complex TETA-Pb(II) in water.53–56
Characterisation of functionalised membranes
To achieve an efficient large-scale water treatment technology for the removal and recovery of lead(II) metal ions, the organic ligand was suspended on a solid support. Electrospun membranes have been considered as the solid support and the adsorbent in this study due to exhibiting high porosity and surface area, making them unique candidates for water purification.27 The functionalisation of the membranes, with the organic ligand, was optimized by increasing the concertation of TETA on the surface of the electrospun PVC membrane (Section 3 in ESI†). Adsorption experiments indicated that TETA-PVC of ratio w/v 1
:
3 resulted in the highest removal efficiency for lead(II) ions in water.
FTIR and TGA curve analyses. Functionalisation of PVC membrane with TETA was confirmed using FTIR, where observing N–H functional groups on the surface of the functionalised PVC membrane is expected. As shown in Fig. S3,† new bands at 1500–1750 cm−1 and 3250–3500 cm−1 corresponding to primary and secondary amines stretching vibrations, respectively, were detected after the functionalisation of the membrane.57,58 Thermogravimetric measurements of PVC and TETA-PVC nanofibers were also performed and presented in Fig. S3.† Fibers of the PVC membrane decompose in the range of 220–240 °C, consistent with previously reported studies.59 The functionalised membrane exhibited lower thermal stability, where the thermal decomposition starts at 180 °C due to the decomposition of the organic ligands at the surface.
SEM–EDX measurements. The PVC and TETA-PVC membranes were analysed using a scanning electron microscope (SEM) and energy dispersive X-ray (EDX). The distribution of fibers in the electrospun membrane was studied by SEM. Fibers of the PVC membrane were observed to be uniformly distributed. The morphology of the PVC fibers changes upon its functionalisation with TETA as indicated in the SEM images. Moreover, the presence of nitrogen from the amine groups of the ligand was detected on the membrane's surface by EDX spectra (Fig. S5f†). The latter is a convenient qualitative validation of the modification of the membrane.60Pore size, membrane thickness, and water contact angle measurements. The average pore diameters of the membranes were measured using a capillary flow porometer. The average pore diameter of the TETA-PVC membrane was found to be 3.2 μm, lower than that of the pristine PVC membrane (3.6 μm) (Fig. S6†). It has been reported in previous studies that the thickness of the electrospun nanofibrous membrane is inversely proportional to its pore size.61 This was confirmed in our results where the thickness of the functionalised membrane was greater than that of the PVC membrane (144 nm TETA-PVC >105 nm PVC) (Table S1†).
The water contact angles (WCA) of PVC and TETA-PVC membranes were measured by an optical tensiometer. As per the literature, pure PVC membrane is relatively hydrophobic with the WCA of above 90°;62 however, upon introducing the TETA linker to the surface, the membrane became more hydrophilic due to the presence of polar amine groups in the organic ligand.63 This was verified by water contact angle measurements by which it decreased from 135° for the pristine PVC membrane to ∼81° for the TETA-PVC membrane (Fig. S8†). The surface modification of PVC membrane with a hydrophilic TETA decreased the contact angle to less than 90°. The improvement of surface hydrophilicity reduces the repulsive forces between the surface and adsorbate in solution;64 thus, enhancing the adsorption capacity for the removal of Pb(II) ions from water.
Lead(II) removal efficiency from water
The removal of Pb(II) ions from water was examined based on increasing PVC to TETA ratios (w/v ratio) and the results are presented in Table S2.† TETA-PVC membrane of ratio 1
:
3 resulted in the highest percentage removal of Pb(II) ions from water, indicating that it is the optimum ratio of PVC (g) to TETA (mL) (see Fig. 4). The removal efficiency was enhanced due to the metal: ligand interaction in the solution.
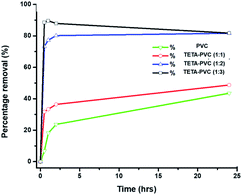 |
| Fig. 4 Percentage removal of [Pb(II)] = 150 mg L−1 from water at room temperature within 24 h, using PVC, TETA-PVC 1 : 1, TETA-PVC 1 : 2, TETA-PVC 1 : 3 membranes. | |
Hence, TETA-PVC with a 1
:
3 w/v ratio was used throughout the adsorption study. SEM analysis was carried out on TETA-PVC after lead(II) ions were adsorbed on its surface. The formation of hexagon-shaped structures on the surface of the TETA-PVC membrane was observed in SEM images (Fig. 5d). However, the pristine membrane did not form any structure after the removal of lead(II) from water. This confirms the formation of a metal–organic complex on the surface of the functionalised membrane. Moreover, the EDX peaks are attributed to lead on the newly formed shapes, supporting the adsorption of Pb(II) onto the functionalised membrane's surface (Fig. S9†).
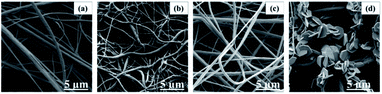 |
| Fig. 5 SEM images of (a): PVC; (b): TETA-PVC, (c): PVC/Pb, and (d): TETA-PVC-/Pb membrane. | |
Adsorption kinetics. The kinetic mechanism controlling the adsorption process of lead(II) by TETA-PVC was investigated to determine the optimum contact time and understand the adsorption process. Kinetic experiments were carried out by exposing the membrane to different contact times with Pb(II) aqueous solution; starting with 10 to 1440 min with initial Pb(II) concentration of 150 mg L−1. Kinetic experiments were carried out at neutral pH (pH 7) and room temperature. Obtained results were analysed with two kinetic models, pseudo-first-order and pseudo-second-order. The rate order constant K1 and K2, qe (quantity adsorbed) values, and the corresponding linear regression correlation coefficients values R2 were calculated and reported in Table S3†.35 The pseudo-second-order equation plot shows a high correlation coefficient (R2 = 0.99) and good compliance with the proposed pseudo-second-order equation. Whereas, the correlation coefficient (R2 = 0.22) obtained by applying the pseudo-first-order equation to the experimental data was very low.The basic assumption of the pseudo-second-order model is that the adsorption process is mainly via chemical adsorption. This suggests that Pb(II) ions form a chemical bond, likely a coordination bond, with the potential amine functional groups of the functionalised PVC membrane. These potential binding sites are the amines of TETA.65,66
Adsorption isotherms for lead(II) removal from water. To gain a better understanding of the interaction between the adsorbate (Pb(II)) and the adsorbent (TETA-PVC), adsorption equilibrium experiments were performed with different concentrations of Pb(II) ions ranging from 600 to 1200 mg L−1 being treated with the 10 mg of TETA-PVC. The experimental data were fitted into two adsorption isotherm models, Langmuir (eqn. (S5)†) and Freundlich (eqn. (S7)†) models.67–69 Adsorption behavior followed the Langmuir model with a correlation coefficient of R2 > 0.99, whereas the Freundlich model showed a lower correlation factor R2 of 0.89. This indicates that the sorption of Pb(II) ions on the TETA-PVC membrane is a monolayer chemical sorption process.70 The maximum adsorption capacity (qmax) and Langmuir constant (KL) were determined from the slope and intercept of the linear plot of Ce/qe vs. Ce (Fig. 6b), and were found to be 1250 mg L−1 and 40.9 × 10−2 L mg−1, respectively. Additionally, the separation factor (RL) was calculated (eqn. (S6)†) to be between 0 and 1, hence the adsorption mechanism of lead ions into the PVC membrane is favorable (Table S4†).71 However, it can be noted that the correlation coefficient of Freundlich is relatively acceptable showing that the adsorption process could be described by both Langmuir and Freundlich models. This elucidates that chemical interaction between the surface of the membrane and the metal could have occurred.32
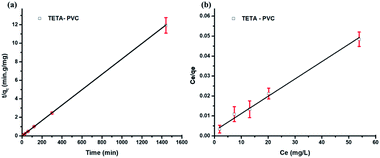 |
| Fig. 6 (a) The pseudo-second-order kinetic plot for the adsorption of ([Pb(II)] = 150 mg L−1) on a 10 mg TETA-PVC membrane (b) Langmuir isotherm plot for the adsorption of Pb(II) onto TETA-PVC membrane. | |
Effect of competing metal ions. The prepared membrane exhibits a high adsorption capacity towards aqueous Pb(II) ions; however, industrial wastewater includes different metal cations that could affect the uptake of lead by the TETA-PVC membrane.72 Therefore, it is crucial to study the effect of co-existing cations in solution on the adsorption capacity of the membrane. To evaluate the selectivity of pristine PVC and TETA-PVC membranes, Hg(II) and Cd(II) were also added to the aqueous solution of Pb(II). The effect of Hg(II) and Cd(II) on Pb(II) removal efficiency by PVC and TETA-PVC membranes is illustrated in Fig. 7.
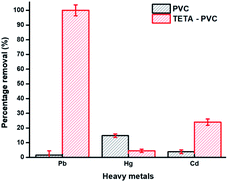 |
| Fig. 7 Removal efficiency of 10 mg of PVC and TETA-PVC membranes in a tertiary system of Pb(II), Hg(II), and Cd(II) ([M] = 150 mg L−1 each). | |
As can be observed, in the tertiary solution of metal ions, the removal percentage of the pristine PVC membrane towards all three metals is relatively low (less than 20%), with the highest removal percentage towards Hg(II) ions (16.5%). Whereas in the presence of the three different cations, the removal of lead(II) by the TETA-PVC membrane was enhanced (≈97%) relative to the removal of lead in the mono-ionic system (≈88%). The selectivity of metal ions for the PVC membrane was in the order of Hg(II) > Cd(II) > Pb(II), as for the TETA-PVC membrane was in the order of Pb(II) > Cd(II) > Hg(II). Similarly, the selectivity of Pb(II) ions by PVC and TETA-PVC membranes was also tested in a mixed aqueous solution composed of six heavy metals (Hg(II), Cd(II), As(III), Zn(II), Cu(II), and Pb(II)). The results showed that TETA-PVC is highly selective for Pb(II) (see Fig. S13†). This confirms the strong interaction of Pb(II) and TETA on the membrane's surface. The electronegativity of heavy metals affects the selectivity adsorption behavior of the adsorbent. Previous studies showed that heavy metal cations with high electronegativity can easily replace cations with lower electronegativity.73 As such, results show that Pb(II) is strongly adsorbed by TETA on the electrospun membrane due to its high electronegativity compared to the present cations. Thus, the TETA-PVC membrane showed high selectivity towards Pb(II) ions. Moreover, the coordination sphere and hydration radius along with other factors, contribute to the high selectivity of TETA-PVC towards Pb(II).73–75
Regeneration of TETA-PVC. The reusability of the functionalised membrane was assessed to reflect the sustainability of the developed adsorbent. As such, six successive adsorption–desorption cycles were performed at the initial metal ion concentration of 700 mg L−1. At the end of each adsorption experiment, the saturated membrane was regenerated in an acidic medium, using 0.1 M p-toluene sulfonic acid (pH = 5.5), where the acidic media is expected to weaken the coordination bond between TETA-PVC and Pb(II) ions.76 The obtained results revealed that the amine functional group of the adsorbent (i.e. –NH2) in the acidic medium became protonated and the adsorbed metal ions on these groups were replaced by H+ ions releasing Pb(II) in solution.77,78 The performance of the regenerated TETA-PVC nanofiber membrane in each cycle is presented in Fig. 8a.
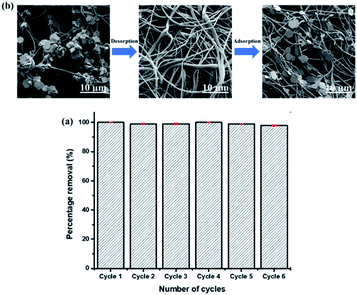 |
| Fig. 8 (a) Reusability of TETA-PVC for the adsorbed amount of Pb(II) ([Pb(II)] = 700 mg L−1), (b) SEM images of the TETA-PVC before and after the adsorption of [Pb(II)] = 700 mg L−1, and after the regeneration. | |
The removal efficiency of Pb(II) by TETA-PVC membrane at a contact time of 30 minutes remained almost constant at around 100% up to 6 cycles. SEM images of the TETA-PVC adsorbent show that after desorption of Pb(II) the hexagon-shaped structures, of the metal–organic complex, disappeared from the surface of the nanofibers of the membrane (Fig. 8b). Additionally, lead was not detected by EDX (Fig. S14†). After six cycles, the membrane maintained a 98% removal efficiency revealing its great potential as a reusable environmentally friendly adsorbent. It is important to note that the above experiments were performed on a wet membrane; however, it was also tested on a membrane that was dried after each cycle and the results obtained were the same for both membranes (wet and dry ones).
Conclusions
In this study, the development of functionalised polymeric PVC electrospun nanofibers as an adsorbent for the removal of lead(II) metal ions in water under ambient conditions has been investigated. Polyvinyl chloride (PVC) membrane was fabricated by electrospinning technique and then functionalised by introducing an amine-based functional group (TETA) onto its surface, to enhance its adsorptive capacity towards metals. Structural and chemical analyses were performed on the membranes to indicate that the grafting of TETA on the PVC membrane has been accomplished. A comparison between the pristine and functionalised membrane with TETA was studied and results showed that lead(II) recovery was enhanced from 30% to 99% in 30 minutes. The effects of contact time, initial concentration of the metal ion (with the lowest concentration of 50 ppm), and presence of different ions in solution on the adsorption properties of the membranes were reported. The results showed that adsorption followed the Langmuir isotherm model with a maximum adsorption capacity of 1250 mg L−1, higher than recently reported studies using membrane, as well as a pseudo-second-order kinetic model. TETA-PVC membrane also showed high selectivity to Pb(II) in a mixed ionic system including Hg(II), Cd(II), As(III), Zn(II), and Cu(II) ions. Moreover, the performance of the membrane persisted after six cycles maintaining a high removal efficiency. Thus, the presented work offers several advancements in nanotechnology for the sustainable and effective removal of Pb(II) from water.
Conflicts of interest
There are no conflicts to declare.
Acknowledgements
R. A. B. acknowledges the K. Shair Central Research Science Laboratory (CRSL) at AUB for providing the facilities to carry out this work.
Notes and references
- J. S. Famiglietti, Nat. Clim. Change, 2014, 4, 945–948 CrossRef.
- J. J. Huang, J. Hydrol., 2015, 11 Search PubMed.
- S. De Gisi, G. Lofrano, M. Grassi and M. Notarnicola, Sustain. Mater. Technol., 2016, 9, 10–40 CAS.
- A. R. Rezaei, Z. Ismail, M. H. Niksokhan, M. A. Dayarian, A. H. Ramli and S. M. Shirazi, Water, 2019, 11, 1415 CrossRef.
- N. Herawati, S. Suzuki, K. Hayashi and I. F. Rivai, Bull. Environ. Contam. Toxicol., 2000, 64, 33–39 CrossRef CAS PubMed.
- D. Santos, R. Vieira, A. Luzio and L. Félix, in Advances in Molecular Toxicology, ed. J. C. Fishbein and J. M. Heilman, Elsevier, 2018, vol. 12, pp. 151–179 Search PubMed.
- V. Masindi and K. L. Muedi, Environmental Contamination by Heavy Metals, IntechOpen, 2018 Search PubMed.
- H. Ali, E. Khan and I. Ilahi, J. Chem., 2019, 2019, 1–14 Search PubMed.
- J. Briffa, E. Sinagra and R. Blundell, Heliyon, 2020, 6, e04691 CrossRef PubMed.
- A. Sigel, H. Sigel and R. K. O. Sigel, Lead: Its Effects on Environment and Health, Walter de Gruyter, Berlin, Germany, 2017, vol. 17 Search PubMed.
- S. Tiwari, I. Tripathi and H. Tiwari, Int. J. Emerg. Res. Manag. Technol., 2013, 2278–9359 Search PubMed.
- World Health Organization, Preventing disease through healthy environments: exposure to lead: a major public health concern, 2nd edn, 2021, World Health Organization, https://apps.who.int/iris/handle/10665/346775, Licence: CC BY-NC-SA 3.0 IGO.
- J. Verma, S. Kumari and A. Dhasmana, Int. J. Innov. Res. Technol., 2020, 7, 673–676 Search PubMed.
- M. Boldyrev, Science, 2018, 1, 1–23 CrossRef.
- N. Corda and M. S. Kini, Sep. Sci. Technol., 2020, 55, 2679–2698 CrossRef CAS.
- F. A. Shammala, Insights Org. Inorg. Chem., 2020, 1, 1–10 Search PubMed.
- Y. Manawi, G. McKay, N. Ismail, A. Kayvani Fard, V. Kochkodan and M. A. Atieh, Chem. Eng. J., 2018, 352, 828–836 CrossRef CAS.
- J. H. Redmon, K. E. Levine, A. M. Aceituno, K. Litzenberger and J. M. Gibson, Environ. Res., 2020, 183, 109126 CrossRef CAS.
- A. A. Siyal, M. R. Shamsuddin, M. I. Khan, N. E. Rabat, M. Zulfiqar, Z. Man, J. Siame and K. A. Azizli, J. Environ. Manage., 2018, 224, 327–339 CrossRef CAS.
- A. Fegousse, A. El Gaidoumi, Y. Miyah, R. El Mountassir and A. Lahrichi, J. Chem., 2019, 2019, 1–11 CrossRef.
- M. Ullah, R. Nazir, M. Khan, W. Khan, M. Shah, S. G. Afridi and A. Zada, Soil Water Res., 2019, 15(2020), 30–37 CrossRef.
- M. Irannajad and H. Kamran Haghighi, Environ. Process., 2021, 8, 7–35 CrossRef CAS.
- M. Manyangadze, N. M. H. Chikuruwo, T. B. Narsaiah, Ch. S. Chakra, G. Charis, G. Danha and T. A. Mamvura, Heliyon, 2020, 6, e05309 CrossRef.
- E. Szatyłowicz and I. Skoczko, J. Ecol. Eng., 2018, 19, 61–67 CrossRef.
- A. Nqombolo, A. Mpupa, R. M. Moutloali and P. N. Nomngongo, Wastewater Treatment Using Membrane Technology, IntechOpen, 2018 Search PubMed.
- J. Zhang, F. Zhou, S. Li, Y. Wan and J. Luo, J. Membr. Sci., 2021, 635, 119451 CrossRef CAS.
- B. M. Thamer, A. Aldalbahi, M. Moydeen A, M. Rahaman and M. H. El-Newehy, Polymers, 2021, 13, 20 CrossRef CAS PubMed.
- F. Zhu, Y.-M. Zheng, B.-G. Zhang and Y.-R. Dai, J. Hazard. Mater., 2021, 401, 123608 CrossRef CAS.
- F. Yang and P. Yang, Macromol. Rapid Commun., 2022, 43, 2100669 CrossRef CAS.
- A. Almasian, G. chizari fard, M. Parvinzadeh Gashti, M. Mirjalili and Z. Mokhtari-Shourijeh, Desalin. Water Treat., 2015, 57, 10333–10348 CrossRef.
- B. M. Thamer, A. Aldalbahi, M. Moydeen A, A. M. Al-Enizi, H. El-Hamshary and M. H. El-Newehy, Sci. Rep., 2019, 9, 19467 CrossRef.
- T. S. Vo, M. M. Hossain, H. M. Jeong and K. Kim, Nano Convergence, 2020, 7, 36 CrossRef CAS.
- S. A. A. N. Nasreen, S. Sundarrajan, S. A. S. Nizar, R. Balamurugan and S. Ramakrishna, Membranes, 2013, 3, 266–284 CrossRef.
- S. R. Zahabi, S. A. Hosseini Ravandi and A. Allafchian, J. Water Health, 2016, 14, 630–639 CrossRef.
- Y. Chen and L. Jiang, Appl. Water Sci., 2021, 11, 51 CrossRef CAS.
- D. M. Warsinger, S. Chakraborty, E. W. Tow, M. H. Plumlee, C. Bellona, S. Loutatidou, L. Karimi, A. M. Mikelonis, A. Achilli, A. Ghassemi, L. P. Padhye, S. A. Snyder, S. Curcio, C. D. Vecitis, H. A. Arafat and J. H. Lienhard, Prog. Polym. Sci., 2018, 81, 209–237 CrossRef CAS.
- M. S. Islam, B. C. Ang, A. Andriyana and A. M. Afifi, SN Appl. Sci., 2019, 1, 1248 CrossRef.
- L. Li, R. Hashaikeh and H. A. Arafat, J. Membr. Sci., 2013, 436, 57–67 CrossRef CAS.
- O. Makanjuola, I. Janajreh and R. Hashaikeh, Desalination, 2018, 436, 98–106 CrossRef CAS.
- M. Manyangadze, N. H. M. Chikuruwo, T. B. Narsaiah, C. S. Chakra, M. Radhakumari and G. Danha, South Afr. J. Chem. Eng., 2020, 31, 25–32 CrossRef.
- J. I. Lachowicz, A.-H. Emwas, G. R. Delpiano, A. Salis, M. Piludu, L. Jaremko and M. Jaremko, Adv. Mater. Interfaces, 2020, 7, 2000544 CrossRef CAS.
- J. Lu, Mol. Cancer Ther., 2010, 9, 2458–2467 CrossRef CAS.
- F. S. Hoseinian, B. Rezai, M. Safari, D. Deglon and E. Kowsari, Hydrometallurgy, 2021, 202, 105609 CrossRef CAS.
- S. Gu, L. Wang, X. Mao, L. Yang and C. Wang, Materials, 2018, 11, 514 CrossRef PubMed.
- A. Almasian, M. Giahi, Gh. Chizari Fard, S. A. Dehdast and L. Maleknia, Chem. Eng. J., 2018, 351, 1166–1178 CrossRef CAS.
- S. Koushkbaghi, A. Zakialamdari, M. Pishnamazi, H. F. Ramandi, M. Aliabadi and M. Irani, Chem. Eng. J., 2018, 337, 169–182 CrossRef CAS.
- L. Zang, R. Lin, T. Dou, L. Wang, J. Ma and L. Sun, Nanoscale Adv, 2019, 1, 389–394 RSC.
- K. Jomová, L. Hudecova, P. Lauro, M. Simunkova, S. H. Alwasel, I. M. Alhazza and M. Valko, Molecules, 2019, 24, 4335 CrossRef PubMed.
- T. Huyen Vu, N. Serradji, M. Seydou, É. Brémond and N.-T. Ha-Duong, J. Inorg. Biochem., 2020, 203, 110864 CrossRef CAS PubMed.
- D. Humelnicu, A. Pui, C. Malutan, Th. Malutan and I. Humelnicu, J. Saudi Chem. Soc., 2020, 24, 451–460 CrossRef CAS.
- A. Trabolsi, M. Elhabiri, M. Urbani, J. L. Delgado de la Cruz, F. Ajamaa, N. Solladié, A.-M. Albrecht-Gary and J.-F. Nierengarten, Chem. Commun., 2005, 5736 RSC.
- F. Zapata, A. Caballero, A. Espinosa, A. Tárraga and P. Molina, J. Org. Chem., 2009, 74, 4787–4796 CrossRef CAS.
- K. N. Houk, A. G. Leach, S. P. Kim and X. Zhang, Angew. Chem., Int. Ed., 2003, 42, 4872–4897 CrossRef CAS PubMed.
- G. Tircsó, Z. Kovács and A. D. Sherry, Inorg. Chem., 2006, 45, 9269–9280 CrossRef PubMed.
- R. Delgado, S. Quintino, M. Teixeira and A. Zhang, J. Chem. Soc. Dalton Trans., 1997, 55–64 RSC.
- E. C. Hulme and M. A. Trevethick, Br. J. Pharmacol., 2010, 161, 1219–1237 CrossRef CAS.
- J. Yu, W. Xiong, J. Zhu, J. Chen and R. Chi, Clean Technol. Environ. Policy, 2017, 19, 517–525 CrossRef CAS.
- M. Faisal, A. Z. Pamungkas and Y. K. Krisnandi, Processes, 2021, 9, 456 CrossRef CAS.
- K. J. Roy, T. V. Anjali and A. Sujith, J. Mater. Sci., 2017, 52, 5708–5725 CrossRef CAS.
- M. f. Gazulla, M. Rodrigo, E. Blasco and M. Orduña, X-Ray Spectrom., 2013, 42, 394–401 CrossRef CAS.
- R. Asmatulu and W. S. Khan, in Synthesis and Applications of Electrospun Nanofibers, ed. R. Asmatulu and W. S. Khan, Elsevier, 2019, pp. 135–152 Search PubMed.
- S. Parvate, P. Dixit and S. Chattopadhyay, J. Phys. Chem. B, 2020, 124, 1323–1360 CrossRef CAS PubMed.
- X. J. Dai, J. du Plessis, I. L. Kyratzis, G. Maurdev, M. G. Huson and C. Coombs, Plasma Process. Polym., 2009, 6, 490–497 CrossRef CAS.
- R. Gläser and J. Weitkamp, in Handbook of Porous Solids, John Wiley & Sons, Ltd, 2002, pp. 395–431 Search PubMed.
- R. R. Aquino, M. S. Tolentino, R. M. P. D. Elacion, R. Ladrillono, T. R. C. Laurenciana and B. A. Basilia, IOP Conf. Ser. Earth Environ. Sci., 2018, 191, 012139 CrossRef.
- F. Zhu, Y. Lu and L. Li, RSC Adv., 2016, 6, 111120–111128 RSC.
- M. B. Desta, J. Thermodyn., 2013, 2013, e375830 CrossRef.
- T. S. Khayyun and A. H. Mseer, Appl. Water Sci., 2019, 9, 170 CrossRef.
- A. Mittal, L. Kurup and J. Mittal, J. Hazard. Mater., 2007, 146, 243–248 CrossRef CAS PubMed.
- Q. Chen, Y. Tian, P. Li, C. Yan, Y. Pang, L. Zheng, H. Deng, W. Zhou and X. Meng, J. Chem., 2017, 2017, 1–11 Search PubMed.
- X. Sun, Ind. Eng. Chem. Res., 2013, 52, 14251–14260 CrossRef.
- A. Baysal, N. Ozbek and S. Akm, in Waste Water - Treatment Technologies and Recent Analytical Developments, ed. F. S. Garca Einschlag, InTech, 2013 Search PubMed.
- X. Fan, H. Liu, E. Anang and D. Ren, Mater. Basel Switz., 2021, 14, 4066 CAS.
- Y. Gao, L. Pan, H. Li, Y. Zhang, Z. Zhang, Y. Chen and Z. Sun, Thin Solid Films, 2009, 517, 1616–1619 CrossRef CAS.
- K. S. Hui, C. Y. H. Chao and S. C. Kot, J. Hazard. Mater., 2005, 127, 89–101 CrossRef CAS PubMed.
- Y. Li, L. Yang, X. Li, T. Miki and T. Nagasaka, J. Hazard. Mater., 2021, 411, 125044 CrossRef CAS PubMed.
- F. Einollahi Peer, N. Bahramifar and H. Younesi, J. Taiwan Inst. Chem. Eng., 2018, 87, 225–240 CrossRef CAS.
- S. Z. N. Ahmad, W. N. Wan Salleh, A. F. Ismail, N. Yusof, M. Z. Mohd Yusop and F. Aziz, Chemosphere, 2020, 248, 126008 CrossRef CAS PubMed.
Footnote |
† Electronic supplementary information (ESI) available: For general methods, supplementary details on the preparation and characterisation of TETA-PVC membrane, sorption isotherms and kinetics, ion selectivity, and regeneration of the adsorbent. See https://doi.org/10.1039/d2ra02946d |
|
This journal is © The Royal Society of Chemistry 2022 |