DOI:
10.1039/D2RA02427F
(Paper)
RSC Adv., 2022,
12, 18773-18778
Photocatalysed direct amination of benzene and ammonia over Ti–V-MCM-41†
Received
15th April 2022
, Accepted 21st June 2022
First published on 28th June 2022
Abstract
Aniline is one of the important organic chemical raw materials and is widely used in the chemical production industry, including dyes, pharmaceuticals, pesticides, explosives, spices, etc. At present, the yield and selectivity of aniline synthesis by direct amination are relatively low, and how to improve the catalytic efficiency has become an urgent problem to be solved. A series of Ti–V-MCM-41 catalysts with different silicon–titanium ratios was prepared by the hydrothermal synthesis method. According to the analysis of XRD, FT-IR, UV-vis DRS, BET, and SEM characterization of Ti–V-MCM-41, it was found that V–O–Si and Ti–O–Si were distributed on Ti–V-MCM-41. The direct photocatalysis amination of benzene and ammonia to aniline can be realized under mild conditions with this catalyst. The yield and selectivity of aniline were improved to 6.11% and 90.7%, respectively, by optimizing the synthesis and reaction conditions.
1. Introduction
Aniline (C6H5NH2) is widely applied to the manufacturing of chemical products, such as dyes, drugs, resins, and rubber vulcanization accelerators.1,2 At present, there is a strong and robust market demand for aniline.3 By the end of 2018, the world's aniline production capacity was 8.647 million tons per year, and the output was 6.706 million tons, increasing by 4.1% and 2.2%, respectively, over the previous year.
The synthesis methods of aniline mainly include multi-step amination and one-step amination. The multi-step amination mainly includes reduction of nitrobenzene, catalytic hydrogenation of nitrobenzene, and amination of phenol,4,5 all of which have the disadvantages of harsh reaction conditions, complicated operation, severe environmental pollution, many by-products, and low atom utilization. The one-step method is also called direct amination, which is the process of activating the C–H bond of the benzene ring, introducing the amino group into the benzene ring directly.6–8 But the yield of aniline synthesized by the one-step method is still low. Therefore, how to improve the yield of one-step amination of benzene has become the core problem in this field, and the key lies in the research of catalysts and amination conditions.9,10
Along with continuously going deep into the research, the yield of aniline has been improved11–13 by direct amination of benzene and hydroxylamine hydrochloride. Compared with the direct amination with hydroxylamine hydrochloride, the yield of aniline with ammonia is much lower. Although hydroxylamine is used as an aminating agent, the yield and selectivity of aniline have significantly been improved. However, the reaction is carried out in an acid medium,13,14 which leads to many problems, such as severe corrosion of instruments and equipment, dissolution of metal catalysts. Furthermore, the cost of hydroxylamine is much higher than that of ammonia. From the perspective of industrialization and green chemistry in the future, it is necessary to further study the one-step synthesis of aniline using ammonia as aminating agent under mild conditions.
According to the previous research, the photocatalysis reaction catalyzed by tetravalent titanium follows a radical reaction process,15–17 and the direct amination of benzene is also a radical substitution reaction process.18 Encouraged by previous work, titanium and vanadium loaded MCM-41 materials were designed and for the first time applied to the photocatalytic one-step synthesis of aniline from benzene using ammonia as the aminating agent under mild conditions, and the photocatalytic radical substitution mechanism has been proposed. The Ti–V-MCM-41 catalyst synthesized here improved the yield of aniline in this system, and provided a theoretical basis for the industrialization of one-step synthesis of aniline using ammonia as the aminating agent.
2. Experimental section
2.1 Preparation of catalyst material samples
Cetyltrimethylammonium bromide (CTAB), tetraethyl orthosilicate (TEOS), vanadyl sulfate (VOSO4), and tetra butyl titanate (TBOT) were required. The reagents and ratio of catalyst preparation were shown in Table S1.†
All the catalysts sample were prepared according to the material ratio in the Table S1.† Firstly, CTAB was dissolved in 30 mL deionized water to prepare a template solution. The VOSO4 was dissolved in 5 mL deionized water. Next, the TEOS, VOSO4 solution and TBOT was added to the template solution according to the proportion in Table S1.† At the same time, the pH of the mixed solution was adjusted using ammonia. Then, the solution was continuously stirred at 60 °C for 1 hour and was left at room temperature for 24 hours. After these procedure, the solution was transferred to the reactor and crystallized at a specific temperature and specific time. After crystallization, the production was washed, filtered and dried. Finally, the catalyst was roasted in a muffle furnace at a specific temperature and specific time. After calcination, the product was ground continuously, and then catalyst powder was obtained. The optimal synthesis conditions will be discussed in ESI from Fig. S1–S5.† The optimal synthesis conditions are: pH = 10.0, crystallization temperature = 110 °C, crystallization time = 24 h, calcination temperature = 550 °C, calcination time = 6 h.
2.2 Characterization and analysis of catalyst
The catalysts prepared were characterized by XRD, FT-IR, UV-vis DRS, BET, and SEM. XRD is used to analyze the diffraction patterns of the materials, and the information of the composition of the materials, the structure or morphology of the atoms or molecules inside the materials can be obtained.19 The scanning angles 2θ are 1–8° and 10–80° respectively, the working voltage is 40 kV, the operating current is 50 mA, and the scanning rate is 1° min−1.
Since the vibration or rotation of different functional groups and chemical bonds will absorb infrared light with different wave numbers, the functional groups or chemical bonds on the catalyst were characterized by FT-IR.19–21 The scanning wave number is 4000–400 cm−1.
2.3 Direct amination of benzene by photocatalysis
Benzene, ammonia, hydrogen peroxide was required for the direct amination. 365–370 nm ultraviolet lamps were installed at the mouth of the conical flask. To ensure that the reaction conditions are isolated from visible light, the conical flask is completely wrapped with aluminium foil. Then the catalyst and benzene were added to the conical flask, ammonia water was added, and hydrogen peroxide was added slowly. In this process, the amination reaction was carried out in a water bath by continuous stirring for three hours. After the reaction, the catalyst was filtered, and then the product was diluted for liquid chromatography and gas chromatography analysis.
The effects of raw material ratio, titanium loading of catalyst, reaction temperature, and UV light intensity on the direct amination of benzene were studied.
2.4 Qualitative and quantitative analysis of products
The reaction products were qualitatively analyzed by gas chromatography. The test conditions were as follows: the vaporizer temperature was 280 °C, the detector temperature was 280 °C. The initial column temperature was 60 °C, and the temperature was kept for 3 min. Then, the temperature was programmed to 280 °C and kept for 10 min. The product was quantified by high performance liquid chromatography. The column temperature was 35 °C, the mobile phase was methanol and water with a ratio of 30
:
70, and the gradient elution method was adopted. The yield and selectivity of the product iscalculated as follows formula:
3. Results and discussion
3.1 Characterization and analysis of catalyst
3.1.1 XRD analysis. As shown in Fig. 1, the diffraction patterns for the molecular sieve samples have 2 peaks at 2.1° and 3.7°, corresponding to (100) and (110) of MCM-41.22 The result indicated that a series of catalysts have a hexagonal pore structure. However, with the decreasing of the molar ratio of silicon to titanium, i.e., with the increasing of titanium load, the diffraction peaks shifted to a large angle. It showed that the skeleton (–SiO4–) of MCM-41 is slightly deformed after loading titanium and vanadium, but the essential skeleton and pore channel of MCM-41 was still intact. However, with the increase of titanium loading, the diffraction peaks of (100) and (110) were no longer sharp at the molar ratio of silicon to titanium is 20, which may be due to the titanium loading, which causes the molecular sieve framework to deform seriously. According to the strength and sharpness of the characteristic peak, it can not only ensure that titanium is loaded on MCM-41, but also keep the characteristic pore structure of MCM-41 when the nSi/nTi = 60.
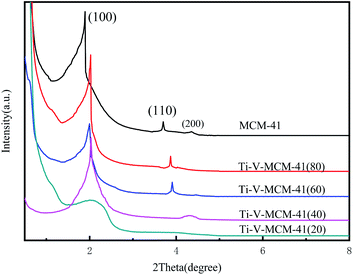 |
| Fig. 1 The XRD patterns of MCM-41 catalysts at 1–8°. | |
As shown in Fig. 2, the characteristic diffraction peaks of SiO2 appeared at 2θ = 22°, 28.4°, 31.4°, which is the framework of MCM-41 molecular sieve. At 2θ = 21.8°, 26°, 31°, the characteristic diffraction peaks of V2Ti3O9 appeared in the Ti–V-MCM-41, which proves that titanium and vanadium have been loaded on MCM-41.
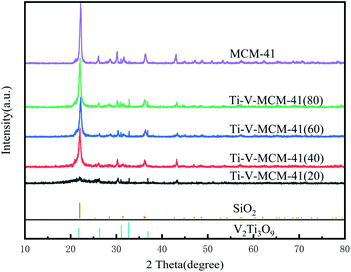 |
| Fig. 2 The XRD patterns of MCM-41 catalysts at 10–80°. | |
3.1.2 FT-IR analysis. As shown in Fig. 3, the infrared absorption peaks of the five groups of molecular sieve samples were 787 cm−1, 1084 cm−1, 3467 cm−1 and 3751 cm−1. The peak at 787 cm−1 corresponds to the bending vibration peak of Si–O–Si, the peak at 1084 cm−1 corresponds to the stretching vibration peak of Si–O–Si, and the peak at 3467 cm−1 corresponds to the vibration absorption peak of Si–OH.23–25 These were the characteristic peaks of MCM-41. And the absorption peak at 3751 cm−1 was caused by lattice water. In the spectrum of Ti–V-MCM-41 sample, a weak peak appeared at 963 cm−1, which corresponds to the asymmetric stretching vibration peak of Ti–O–Si.23,24 It was due to the skeleton asymmetry caused by titanium replacing silicon. Although this absorption peak was weak as a result of the small loading amount of titanium, it could still prove that titanium has been loaded on the MCM-41.
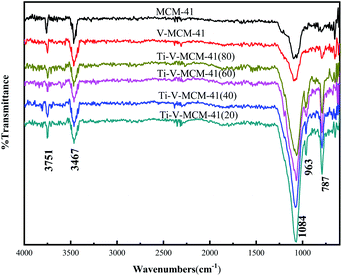 |
| Fig. 3 The FT-IR spectrograms of different catalysts. | |
3.1.3 UV-vis DRS analysis. As shown in Fig. 4, there is no peak in the range of 180–800 nm for MCM-41 samples. The samples loaded with vanadium have a strong peak at 270–300 nm. The samples loaded with titanium also has peaks at 210–300 nm. It can be concluded that Ti–V-MCM-41 has good UV absorption performance. The peak at 200–220 nm indicates that titanium exists in the form of tetra coordinated Ti(IV).23,24 The absence of a peak at 380 nm indicates that anatase type TiO2 does not exist in the catalyst, and titanium in the catalysts exist in the form of tetra-coordination Ti(IV), without non-skeleton titanium.23,24
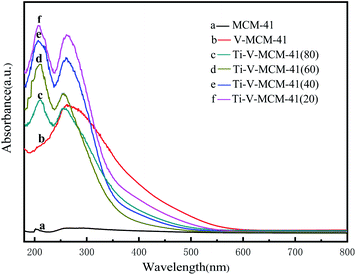 |
| Fig. 4 The UV-vis DRS spectrograms of different catalysts. | |
3.2 Direct amination of benzene by photocatalysis
3.2.1 Effect of titanium loading. According to the above experimental results, the optimal synthesis conditions are: pH = 10.0, crystallization temperature = 110 °C, crystallization time = 24 h, calcination temperature = 550 °C, calcination time = 6 h. Under these optimal synthesis conditions, a series of molecular sieve catalysts with different nSi/nTi molar ratios were synthesized, denoted as MCM-41, V-MCM-41, Ti-MCM-41(60), Ti–V-MCM-41(20), Ti–V-MCM-41(40), Ti–V-MCM-41(60) and Ti–V-MCM-41(80). The catalyst V-MCM-41 is not loaded with Ti, so its nSi/nTi is denoted as “∞”. In aniline synthesis experiments, the MCM-41 had no catalytic effect on the direct amination of benzene. The yield and selectivity of aniline were enhanced while MCM-41 was loaded with vanadium (yield and selectivity of V-MCM-41 are 86.3 and 2.19, respectively), as shown in Fig. 5. In addition, when Ti is loaded on MCM-41, it also exhibits relatively high catalytic efficiency. The yield and selectivity of Ti-MCM-41(60) are 0.47% and 40.3%, respectively. However, when bimetallic supported Ti–V-MCM-41 catalyst were used to catalyzed the reaction, the catalytic efficiency was further improved and reached the maximum value when nSi
:
nTi = 60 (yield and selectivity are 6.11% and 90.7%, respectively). It can be seen that the loading of Ti and V together improved the performance of the catalyst.
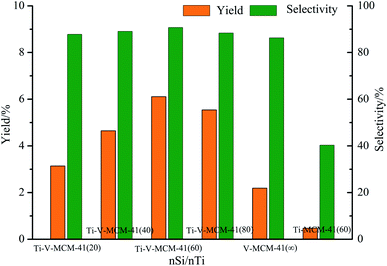 |
| Fig. 5 Yield and selectivity of aniline over Ti–V-MCM-41 catalysts prepared at different titanium loading. | |
3.2.2 Ratio of benzene, NH3·H2O and H2O2. To explore the effect of reactant ratios on the amination of benzene, six experiments were designed for the direct amination of benzene with different ratios of benzene, NH3·H2O, and H2O2. Ti–V-MCM-41(60) (1.05 g) was used in the experiments because it showed the highest catalytic efficiency, as mentioned in 3.2.1 Section. The power of the UV lamp is 0.18 W, and the reaction temperature was 50 °C. The yield and selectivity of aniline with different reactant ratios are shown in Fig. 6. From Fig. 6, it can be concluded that the yield and selectivity of aniline increase with the increase of NH3·H2O and H2O2. And the yield and selectivity of aniline reached the maximum at C6H6
:
NH3·H2O
:
H2O2 = 1
:
3
:
2, which were 6.11% and 90.7%, respectively. The amounts of benzene, ammonia and hydrogen peroxide used were 0.0467, 0.1401 and 0.0934 moles, respectively.
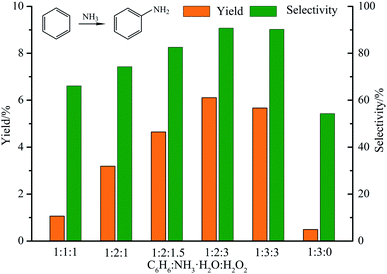 |
| Fig. 6 The yield and selectivity of aniline with different ratios of raw materials. | |
We also discussed the effect of H2O2 on yield and selectivity, as shown in Fig. 6. Among all controls groups, the group without H2O2 (C6H6
:
NH3·H2O
:
H2O2 = 1
:
3
:
0) exhibited the lowest catalytic efficiency, the yield and selectivity are 0.49% and 54.3% respectively. Indeed, H2O2 promoted the ammoniation of benzene. In particular, when the ratio was C6H6
:
NH3·H2O
:
H2O2 = 1
:
3
:
3, the catalytic efficiency began to decrease, which may be caused by unnecessary side reactions. That is, excess H2O2 lead to production of a large amount of phenol.17
3.2.3 Reaction temperature. To explore the effect of reaction temperature on the amination of benzene, five groups of direct amination experiments of benzene were designed under different reaction temperatures. The catalyst is Ti–V-MCM-41(60) (1.05 g), the ratio of benzene, NH3·H2O, and H2O2 were 1
:
3
:
2, the power of the ultraviolet lamp is 0.18 W. As shown in Fig. 7, when the temperature is lower than 50 °C, increasing the temperature promoted the direct amination of benzene. The yield and selectivity of aniline reached the highest at 50 °C (the yield and selectivity of aniline were 6.11% and 90.7%). On the contrary, when the temperature is higher than 50 °C, the increase of temperature inhibited the direct amination of benzene, which may be due to excessive volatilization of ammonia.
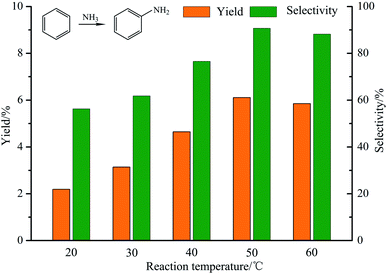 |
| Fig. 7 The yield and selectivity of aniline with different temperatures. | |
3.2.4 Intensity of ultraviolet light. To explore the effect of intensity of ultraviolet light on the amination of benzene, six groups of direct amination experiments of benzene were designed under different UV intensities. The catalyst was Ti–V-MCM-41(60) (1.05 g), the ratio of benzene, NH3·H2O, and H2O2 were 1
:
3
:
2, the reaction temperature was 50 °C. The yield and selectivity of aniline are shown in Fig. 8. According to Fig. 8, the yield and selectivity of aniline increase with the increase of UV intensity before 0.18 W. When the ultraviolet emission power is 0.18 W, the yield and selectivity of aniline both reach the maximum value, which are 6.11% and 90.7%, respectively. The yield and selectivity of aniline will maintain a constant value, instead of continuing to increase while the UV intensity continues to grow. In order to prove that UV light played an important role in the reaction, the control group experiment was performed which was under heat and without UV light, the date was shown in Fig. 8. When the UV intensity was 0, the yield and selectivity are 0.87% and 70.6%, which was obviously lower than when UV intensity was 0.06 W. It shows that UV irradiation plays a great role in promoting the amination of benzene. Indeed, UV irradiation greatly accelerated the reaction.
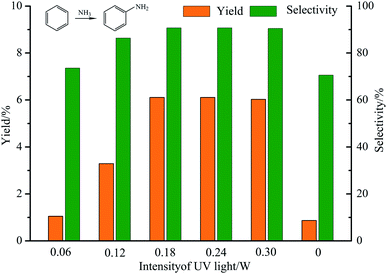 |
| Fig. 8 The yield and selectivity of aniline with different intensity of UV. | |
3.3 Catalytic reaction mechanism
The reaction path of the photocatalytic amination reaction is proposed as follows: titanium(IV) in the Ti–V-MCM-41 catalyst generates electron–hole pair (e−/h+) under the excitation of the photon. Water molecules (H2O) and hydroxyl ions (OH−) generated a small number of hydroxyl radicals (˙OH) with substantial oxidation properties under the action of the hole. Electron and hydrogen peroxide (H2O2) produced a large number of hydroxyl radicals. These hydroxyl radicals oxidize ammonia molecules (NH3·H2O) into amino radicals (˙NH2), and then the amino radicals are converted into protonated amino radicals (˙NH3+) in the water. Benzene is activated into phenyl radical in the presence of the catalyst, and then protonated amino radicals can combine with phenyl radical to produce aminocyclohexanene intermediate. Under the action of the catalyst, the intermediate is transformed into aniline and water.
4. Conclusions
In the present work, a series of Ti–V-MCM-41 catalysts different silicon–titanium ratios was prepared by hydrothermal synthesis. After different characterization analysis, Ti–V-MCM-41 has good crystallization condition, titanium is successfully loaded on MCM-41 support, and no non-framework titanium is generated. The pore structure is relatively complete, with a large specific surface area. Pore size distribution is uniform, mostly concentrated in 2–5 nm, which is beneficial to improve the yield of aniline. The N2 adsorption–desorption isotherm, pore size distribution, BET analysis and SEM can be seen in Fig. S6, S7, Table S2 and Fig. S8.†
The synthesized Ti–V-MCM-41 catalysts were used in the experiment of direct amination of benzene by ultraviolet light, and the optimal synthesis conditions were as follows: the mixed solution pH = 10.0, the crystallization temperature = 110 °C, the crystallization time = 24 h, the calcination temperature = 550 °C, the calcination time = 6 h, and the nSi/nTi = 60. The optimal reaction conditions are as follows: C6H6
:
NH3·H2O
:
H2O2 = 1
:
3
:
2, the reaction temperature is 50 °C, and the UV intensity is 0.18 W. Under these reaction conditions, the yield and selectivity can reach the maximum value, 6.11% and 90.7%, respectively.
Conflicts of interest
The authors declare no competing financial interest.
Author contributions
Conceptualization, C.-H. Y.; investigation, X.-M. W. and C.-H. Y.; methodology, C.-H. Y. and X.-M. W.; visualization, X.-M. W. and C.-H. L.; writing – original draft, X.-M. W.; writing – review & editing, C.-H. Y., C.-H. L., X.-M. W. and W.-T. Z. All authors reviewed the manuscript.
Acknowledgements
This research was funded by Scientific Research Staring Foundation for PhDs of Northeast Electric Power University (BSJXM-201322) and Department of Education of Jilin Province for the “the Twelfth Five” Scientific and Technological Research Projects (2015-247).
Notes and references
- L. Schmerling, US Pat.No. 2948755, 1960 Search PubMed.
- D. Edgren, H. Leeper, K. Nichols, et al. Kirk-Othmer Encyclopedia of Chemical Technology, John Wiley & Sons, Inc., 2000 Search PubMed.
- J. F. Hartwig, S. Shekhar, Q. Shen, et al. Synthesis of Anilines. Patai's Chemistry of Functional Groups, John Wiley & Sons, Ltd, 2009 Search PubMed.
- D. S. Surry and S. L. Buchwald, J. Am. Chem. Soc., 2007, 129, 10354–10355 CrossRef CAS PubMed.
- K. Weissermel and A. Hansmgürgen, Industrial Organic Chemistry, 4th edn, 2008 Search PubMed.
- S. Diao, W. Qian, G. Luo, W. Fei and W. Yao, Appl. Catal., A, 2005, 286, 30–35 CrossRef CAS.
- A. Hagemeyer, R. Borade, P. Desrosiers, S. Guan and U. Notheis, Catal. Today, 2003, 81, 319–328 CrossRef.
- Y. W. Zheng, B. Chen, P. Ye, K. Feng, W. Wang, Q. Y. Meng, L. Z. Wu and C. H. Tung, J. Am. Chem. Soc., 2016, 138, 10080–10083 CrossRef CAS PubMed.
- T. H. Yu, R. G. Yang, S. Xia, G. Y. Li and C. W. Hu, Catal. Sci. Technol., 2014, 4, 3159–3167 RSC.
- J. Becker and W. F. Hlderich, Catal. Lett., 1998, 54, 125–128 CrossRef CAS.
- C. Tong, C. Hu, Z. Fu and A. Tian, Chin. Sci. Bull., 2002, 47, 1937–1939 CrossRef.
- C. H. Yang, G. Chen and L. Zhang, Adv. Mat. Res., 2012, 550–553, 2607–2611 CAS.
- K. M. Parida, S. S. Dash and S. Singha, Appl. Catal., A, 2008, 351, 59–67 CrossRef CAS.
- H. Yuzawa and H. Yoshida, Chem. Commun., 2010, 46, 8854–8856 RSC.
- Y. Sohn, W. X. Huang and F. Taghipour, Appl. Surf. Sci., 2017, 396, 1696–1711 CrossRef CAS.
- S. J. Tan, H. Feng, Y. F. Ji, Y. Wang, J. Zhao, A. D. Zhao, B. Wang, Y. Luo, J. L. Yang and J. G. Hou, J. Am. Chem. Soc., 2012, 134, 9978–9985 CrossRef CAS PubMed.
- Q. W. Huang, S. Q. Tian, D. W. Zeng, X. X. Wang, W. L. Song, Y. Y. Li, W. Xiao and C. S. Xi, ACS Catal., 2013, 3, 1477–1485 CrossRef CAS.
- C. H. Yang and G. Chen, J. Chem. Soc. Pak., 2016, 38, 282–286 CAS.
- C. Y. Chen, H. X. Li and M. E. Davis, Microporous Mater., 1993, 2, 17–26 CrossRef CAS.
- K. Nakamoto, Theory & Applications in Inorganic Chemistry, 1977, vol. 5, pp. 88–97 Search PubMed.
- E. R. Lippincott, J. Am. Chem. Soc., 1963, 85, 3532 CrossRef.
- E. R. Lippincott, J. Am. Chem. Soc., 1963, 85, 3532 CrossRef.
- T. Blasco, A. Corma, M. T. Navarro and J. P. Pariente, J. Catal., 1995, 156, 65–74 CrossRef CAS.
- J. Zhang, D. S. Zhao, M. S. Liu and J. P. Li, Adv. Mat. Res., 2012, 557–559, 1411–1414 CAS.
- L. Wei, H. Zhang, Y. Dong, W. Song, X. Liu and Z. Zhao, RSC Adv., 2016, 6, 71375–71383 RSC.
|
This journal is © The Royal Society of Chemistry 2022 |