DOI:
10.1039/D2RA02167F
(Paper)
RSC Adv., 2022,
12, 17617-17620
Stereocontrolled access to δ-lactone-fused-γ-lactams bearing angular benzylic quaternary stereocenters†
Received
4th April 2022
, Accepted 7th June 2022
First published on 14th June 2022
Abstract
C-fused γ-lactam-lactones are resident in several bioactive molecules, including anticancer agents such as omuralide. In this embodiment, we report mild conditions for the catalytic halolactonization of lactam-tethered 5-aryl-4(E)-pentenoic acids. The use of dichloromethane as the solvent and Ph3P
S as the catalyst led to predominant 6-endo-trig cyclization and furnished the trans-fused-γ-lactam-δ-lactones. The transformation is modular, regioselective, chemoselective, and diastereoselective. The γ-lactam-δ-lactones bear angular quaternary benzylic stereocenters, which is noteworthy since the presence of a quaternary carbon in bioactive small molecules often promotes an element of conformational restriction that imparts potency, selectivity, and metabolic stability. The generated halogen and lactone motifs are important functional handles for late-stage diversification.
There are high incentives for the construction of architecturally complex sp3-enriched azaheterocyclic scaffolds. Specifically, the lactam topology is omnipresent in medicinal chemistry and drug discovery programs.1 In addition to their well-studied antibiotic activity,2 several functionalized lactams act as opioid receptor agonists,3 HIV-1 integrase inhibitors,4 anticancer,5 antidepressant,6 and anti-inflammatory agents.7 Meanwhile, lactones are ubiquitous architectures in a variety of natural products and pharmaceuticals.8 Lactones are also frequently used as versatile building blocks for accessing other oxygen-containing heterocycles and carboxylic acid derivatives.9 Importantly, sp3-rich fused γ-lactam-lactones are resident in bioactive molecules such as neooxazolomycin, UCS 1025 A, and omuralide (Fig. 1). Lovering has articulated that both molecular complexity (as measured by Fsp3, where Fsp3 refers to the ratio of sp3 hybridized carbons to the total number of carbons) and the presence of carbon stereocenters correlate with success as compounds transition from discovery, through clinical testing, to drugs.10 Medicinal chemists are therefore becoming increasingly keen on escaping flatland in view of exploring 3D-structural space, which makes these sp3-rich fused lactam-lactones valued targets for pharmaceutical companies. Some elegant strategies have fittingly emerged for the construction of fused lactam-lactones, including those developed by Wee11 (using C–H insertion) and Burton12 (via oxidative radical cyclization).
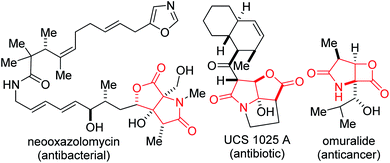 |
| Fig. 1 Examples of C-fused bioactive γ-lactam-lactones. | |
Our interest in the synthesis and post-diversification of 1,3-azadiene-cyclic anhydride annulation products13 prompted us to explore a lactamization/lactonization sequence as a method to rapidly construct sp3-rich fused γ-lactam-δ-lactones bearing quaternary and contiguous stereocenters. Toward this end, we sought to interrogate lactam-bearing alkenoic acids of type 3 in a catalytic halolactonization protocol (Fig. 2). Molecules containing all-carbon quaternary stereocenters have the propensity to mitigate a spectrum of structural diversity issues in medicinal chemistry mainly because the presence of a quaternary carbon in bioactive small molecules promotes an element of conformational restriction that imparts potency, selectivity, and metabolic stability.14 Indeed, of the top 120 chiral small-molecule pharmaceuticals by retail sales in the United States in 2018, 13% contained a quaternary stereocenter.15
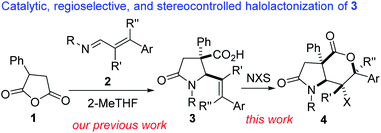 |
| Fig. 2 Proposed plan for the synthesis of fused γ-lactam-fused-δ-lactones. | |
Among the reported methods for the construction of common-ring lactones,16 halogen-initiated cyclization of unsaturated carboxylic acids is an attractive approach for the stereoselective synthesis of halogenated lactones.17,18 The transformation is very versatile given that the generated halogen and lactone motifs are important functional handles for late-stage diversification. In these studies, we show that lactam-tethered alkenoic acids of type 3 undergo efficient catalytic halolactonization to furnish fused γ-lactam-δ-lactones of type 4.
We initiated studies toward the construction of fused γ-lactam-lactones by benchmarking our optimization efforts for bromolactonization of alkenoic acid 3a with the reaction conditions described in Table 1. Dichloromethane emerged as the preferred reaction medium (entries 1–4). Other sources of bromine that were surveyed were less efficient than N-bromosuccinimide (entries 5 and 6). Ph3P
S emerged as the most effective catalyst (entries 7–12). Other reaction conditions known to promote bromolactonization of simple alkenoic acids were also surveyed (entries 13–17). Under the optimized conditions, lactam-lactone 4a was obtained in good yield, high anti-stereoselectivity, and in impeccable 6-endo selectivity. A widely accepted mechanism for this electrophilic halolactonization is that an electrophilic halogen is first transferred from a halogen source to the olefin to form a halonium ion, followed by an intramolecular attack by the carboxylate group. The observed anti addition in a Markovnikov sense is the expected outcome for such 5-aryl-4(E)-pentenoic acids, characterized by an unsymmetrical build-up of positive charge next to the aryl group in the transition state.19 Previous ab initio simulations revealed some syn-directing noncovalent interactions such as hydrogen bonding between the nucleophile and the halogen source, which control the formation of the syn-product.20 The disruption of these syn-directing interactions by a basic additive or a protic solvent, results in a strongly increased diastereoselectivity toward the anti-product. Thus, we surmise that Ph3P
S enhances the anti-diastereoselectivity. This is further supported by observations that in the absence of Ph3P
S (entry 7), 4a was obtained in 83
:
17 (anti
:
syn) ratio.
Table 1 Optimization of the bromolactonization of lactam acid 3a
The scope of the transformation with respect to the nature of the styrenyl group and the substituent on nitrogen (alkyl, aryl, allyl, and benzyl) has been explored. Gratifyingly, several γ-lactam-tethered alkenoic acids undergo 6-endo bromolactonization, giving rise to the 5,6-bicycles depicted in Scheme 1 (see 4a–s). Electron-deficient styrenoic acids are more competent than their electron-rich congeners, owing to their ability to further stabilize the benzylic positive charge (4b vs. 4c). The N-substituent has a profound effect on the diastereoselectivity as exemplified by the switch from a tert-butyl group to a less bulky but electronically similar isopropyl substituent (4a vs. 4g). Encouragingly, several N-arylated γ-lactam-tethered alkenoic acids underwent productive 6-endo-cyclization (see 4i–n), which is noteworthy since N-aryl γ-lactams are embedded in several pharmacologically pertinent targets. As a testament to the remarkable chemoselectivity of the transformation, lactam-tethered alkenoic acids bearing an N-allyl substituent react with NBS to afford bicycles 4o/p, without complications arising from bromolactonization of the kinetically more accessible allyl group. We attribute this chemoselective bromolactonization to conformational constraints and to the more activated nature of the styrenyl double bond. The use of trisubstituted alkenoic acids has facilitated the stereocontrolled construction of lactam-lactones bearing two tetrasubstituted stereocenters (see 4q–s). This is noteworthy since halolactonization reactions of trisubstituted alkenes are not often stereospecific.19 The successful and efficient synthesis of 4r/s suggests that the electronic benefit of the two phenyl groups far outweighs the presumed steric encumbrance.
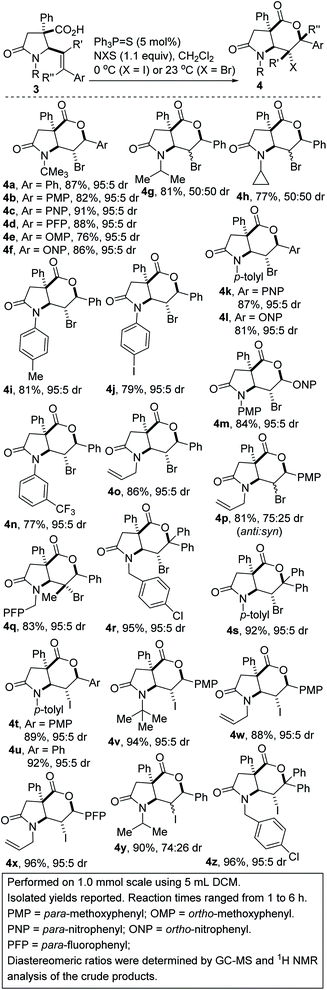 |
| Scheme 1 Regioselective 6-endo-cyclization of γ-lactam-tethered alkenoic acids by catalytic halolactonization. | |
Iodolactonization with N-iodosuccinimide (NIS) also proceeded regio- and diastereoselectively (see 4t–z). In these cases, the iodolactonization was performed at 0 °C, owing to the enhanced reactivity of NIS. The results indicate that the anti
:
syn ratio is further enhanced when NIS is used in place of NBS (4g vs. 4y or 4p vs. 4w).
In summary, the site-selective, diastereoselective, and scalable synthesis of halogenated fused γ-lactam-δ-lactones has been accomplished, through the deployment of γ-lactam-tethered alkenoic acids21 in a catalytic halolactonization protocol. The expected 6-endo cyclization of these lactam-tethered 5-aryl-4(E)-pentenoic acids predominates in dichloromethane. These sp3-rich fused γ-lactam-δ-lactones bear medicinally relevant quaternary and contiguous stereocenters. We anticipate that this practical, cost-effective, and catalytic strategy would undeniably expand the 3D-structural space for the discovery of new γ,δ-lactam-lactones with medicinal value. Post-modification of these versatile N,O-heterocycles is underway. Additionally, efforts to render the transformation enantioselective will be reported indue course following completion of the studies.
Author contributions
M. J. R. – investigation, data curation, validation; C. B. – investigation, methodology; T. K. B. – conceptualization, project administration, supervision, writing – original draft, internal funding acquisition.
Conflicts of interest
There are no conflicts of interest to declare.
Acknowledgements
We are grateful to Central Washington University for financial support through startup funds. The school of graduate studies is thanked for research fellowships to T. K. B. and M. J. R. We also thank the Office of Undergraduate Studies and Provost DenBeste for research fellowships to C. B.
Notes and references
-
(a) L. I. Llarrull, S. A. Testero, J. F. Fisher and S. Mobashery, Curr. Opin. Microbiol., 2010, 13, 551–557 CrossRef CAS PubMed;
(b) J. Caruano, G. G. Muccioli and R. Robiette, Org. Biomol. Chem., 2016, 14, 10134–10156 RSC;
(c) A. Lepikhina, O. Bakulina, D. Dar'in and M. Krasavin, RSC Adv., 2016, 6, 83808–83813 RSC;
(d) P. Gross and J. A. Zapp, CRC Crit. Rev. Toxicol., 1984, 13, 205–216 CrossRef CAS PubMed;
(e) F. J. R. Rombouts, G. Tresadern, O. Delgado, C. Martinez Lamenca, M. Van Gool, A. Garcia-Molina, S. A. Alonso de Diego, D. Oehlrich, H. Prokopcova, J. M. Alonso, N. Austin, H. Borghys, S. Van Brandt, M. Surkyn, M. De Cleyn, A. Vos, R. Alexander, G. Macdonald, D. Moechars, H. Gijsen and A. A. Trabanco, J. Med. Chem., 2015, 58, 8216 CrossRef CAS PubMed.
-
(a) M. Shahid, F. Sobia, A. Singh, A. Malik, H. M. Khan, D. Jonas and P. M. Hawkey, Crit. Rev. Microbiol., 2009, 35, 81–108 CrossRef CAS;
(b) K.-F. Kong, L. Schneper and K. Mathee, APMIS, 2010, 118, 1–36 CrossRef CAS PubMed;
(c) G. Kapoor, S. Saigal and A. Elongavan, J. Anaesthesiol., Clin. Pharmacol., 2017, 33, 300–305 CrossRef CAS.
-
(a) R. D. Marco, A. Bedini, S. Spampinato, L. Comellini, J. Zhao, R. Artali and L. Gentilucci, J. Med. Chem., 2018, 61, 5751–5757 CrossRef PubMed;
(b) S. M. Rawls, W. Robinson, S. Patel and A. Baron, Neuropharmacology, 2008, 55, 865 CrossRef CAS PubMed.
- E. J. Velthuisen, B. A. Johns, D. P. Temelkoff, K. W. Brown and S. C. Danehower, Eur. J. Med. Chem., 2016, 117, 99–112 CrossRef CAS.
-
(a) M. Baiula, P. Galletti, G. Martelli, R. Soldati, L. Belvisi, M. Civera, S. D. Dattoli, S. M. Spampinato and D. Giacomini, J. Med. Chem., 2016, 59, 9721–9742 CrossRef CAS;
(b) D. Kuhn, C. Coates, K. Daniel, D. Chen, M. Bhuiyan, A. Kazi, E. Turos and Q. P. Dou, Front. Biosci., 2004, 9, 2605–2617 CrossRef;
(c) B. Xing, J. Rao and R. Liu, Mini-Rev. Med. Chem., 2008, 8, 455–471 CrossRef CAS.
-
(a) D. J. Greenblatt and R. I. Shader, N. Engl. J. Med., 1978, 299, 1342–1344 CrossRef CAS;
(b) I. A. Volchegorskii and E. A. Trenina, Bull. Exp. Biol. Med., 2006, 142, 73–75 CrossRef CAS;
(c) K. Gillard, H. B. Miller and M. S. Blackledge, Chem. Biol. Drug Des., 2018, 92, 1822–1829 CrossRef CAS PubMed.
-
(a) C. Saturnino, B. Fusco, P. Saturnino, G. D. E. Martino, F. Rocco and J.-C. Lancelot, Biol. Pharm. Bull., 2000, 23, 654–656 CrossRef CAS;
(b) J. Wei, X. Pan, Z. Pei, W. Wang, W. Qiu, Z. Shi and G. Xiao, J. Trauma Acute Care Surg., 2012, 73, 654–660 CrossRef CAS.
-
(a) R. M. Trend, Y. K. Ramtohul, E. M. Ferreira and B. Stoltz, Angew. Chem., Int. Ed., 2003, 42, 2892 CrossRef CAS PubMed;
(b) X.-F. Cheng, Y. Li, Y.-M. Su, F. Yin, J.-Y. Wang, J. Sheng, H. U. Vora, X.-S. Wang and J.-Q. Yu, J. Am. Chem. Soc., 2013, 135, 1236 CrossRef CAS PubMed;
(c) W. Yang, S. Wang, Q. Zhang, Q. Liu and X.-X. Xu, Chem. Commun., 2015, 51, 661 RSC;
(d) X.-M. Xie and S. S. Stahl, J. Am. Chem. Soc., 2015, 137, 3767 CrossRef CAS PubMed;
(e) H. Shigehisa, M. Hayashi, H. Ohkawa, T. Suzuki, H. Okayasu, M. Mukai, A. Yamazaki, R. Kawai, H. Kikuchi, Y. Satoh, A. Fukuyama and K. Hiroya, J. Am. Chem. Soc., 2016, 138, 10597 CrossRef CAS PubMed;
(f) Y.-J. Zhang, T. Abe, T. Tanaka, C.-R. Yang and I. Kouno, J. Nat. Prod., 2001, 64, 1527 CrossRef CAS PubMed;
(g) J. J. Beck and S.-C. Chou, J. Nat. Prod., 2007, 70, 891 CrossRef CAS PubMed;
(h) Y. Wache, M. Aguedo, J. M. Nicaud and J. M. Belin, Appl. Microbiol. Biotechnol., 2003, 61, 393 CrossRef CAS PubMed;
(i) A. Parenty, X. Moreau and J. M. Campagne, Chem. Rev., 2006, 106, 911 CrossRef CAS;
(j) M. I. Konaklieva and B. J. Plotkin, Mini-Rev. Med. Chem., 2005, 5, 73 CrossRef CAS PubMed;
(k) I. Collins, J. Chem. Soc., Perkin Trans. 1, 1998, 1, 1869–1888 RSC.
-
(a) S. Rashid, B. A. Bhat and G. Mehta, Org. Lett., 2015, 17, 3604–3607 CrossRef CAS;
(b) J. Cao and P. Perlmutter, Org. Lett., 2013, 15, 4327–4329 CrossRef CAS PubMed.
- F. Lovering, J. Bikker and C. Humblet, J. Med. Chem., 2009, 52, 6752–6756 CrossRef CAS PubMed.
- A. G. H. Wee, G.-J. Fan and H. M. Bayirinoba, J. Org. Chem., 2009, 74, 8261–8271 CrossRef CAS PubMed.
- A. W. J. Logan, S. J. Sprague, R. W. Foster, L. B. Marx, V. Garzya, M. S. Hallside, A. L. Thompson and J. W. Burton, Org. Lett., 2014, 16, 4078–4081 CrossRef CAS.
-
(a) H. Braunstein, S. Langevin, M. Khim, J. Adamson, K. Hovenkotter, L. Kotlarz, B. Mansker and T. K. Beng, Org. Biomol. Chem., 2016, 14, 8864–8872 RSC;
(b) T. K. Beng and A. Moreno, New J. Chem., 2020, 44, 4257–4261 RSC;
(c) T. K. Beng, M. Bauder, M. J. Rodriguez and A. Moreno, New J. Chem., 2018, 42, 16451–16455 RSC;
(d) K. Hovenkotter, H. Braunstein, S. Langevin and T. K. Beng, Org. Biomol. Chem., 2017, 15, 1217–1221 RSC;
(e) T. K. Beng and A. Moreno, RSC Adv., 2020, 10, 8805–8809 RSC.
-
(a) L. D. Julian and J. F. Hartwig, J. Am. Chem. Soc., 2010, 132, 13813–13822 CrossRef CAS PubMed;
(b) H. Shigehisa, N. Koseki, N. Shimizu, M. Fujisawa, M. Niitsu and K. Hiroya, J. Am. Chem. Soc., 2014, 136, 13534–13537 CrossRef CAS PubMed;
(c) Z. Fang, Y. Song, P. Zhan, Q. Zhang and X. Liu, Future Med. Chem., 2014, 6, 885–901 CrossRef CAS PubMed;
(d) P. A. Clemons, J. A. Wilson, V. Dancik, S. Muller, H. A. Carrinski, B. K. Wagner, A. N. Koehler and S. L. Schreiber, Proc. Natl. Acad. Sci., 2011, 108, 6817–6822 CrossRef CAS PubMed;
(e) Y. Liu, S. J. Han, W. B. Liu and B. M. Stoltz, Acc. Chem. Res., 2015, 48, 740–751 CrossRef CAS PubMed.
- N. A. Mcgrath, M. Brichacek and J. T. Njardarson, J. Chem. Educ., 2010, 87, 1348–1349 CrossRef CAS.
- M. Maier, in Science of Synthesis, ed. E. M. Carreira and J. S. Panek, Thieme, Stuttgart, 2010, vol. 20, pp. 1421–1551 Search PubMed.
-
(a) A. N. French, S. Bissmire and T. Wirth, Chem. Soc. Rev., 2004, 33, 354 RSC;
(b) H. Fujioka and K. Murai, Heterocycles, 2013, 87, 763 CrossRef;
(c) Y. Cheng, W. Yu and Y.-Y. Yeung, Org. Biomol. Chem., 2014, 12, 2333 RSC.
-
(a) M. Okada, K. Kaneko, M. Yamanaka and S. Shirakawa, Org. Biomol. Chem., 2019, 17, 3747–3751 RSC;
(b) R. Kristianslund, J. E. Tungen and T. V. Hansen, Org. Biomol. Chem., 2019, 17, 3079–3092 RSC;
(c) T. Chen, T. J. Y. Foo and Y.-Y. Yeung, ACS Catal., 2015, 5, 4751–4755 CrossRef CAS;
(d) J. Wong and Y.-Y. Yeung, RSC Adv., 2021, 11, 13564–13570 RSC;
(e) K. Moriyama, M. Kuramochi, S. Tsuzuki, K. Fujii and T. Morita, Org. Lett., 2021, 23, 268–273 CrossRef CAS PubMed;
(f) J. E. Tungen, R. Kristianslund, A. Vik and T. V. Hansen, J. Org. Chem., 2019, 84, 11373–11381 CrossRef CAS PubMed;
(g) Y. A. Cheng, T. Chen, C. K. Tan, J. J. Heng and Y.-Y. Yeung, J. Am. Chem. Soc., 2012, 134, 16492–16495 CrossRef CAS PubMed.
-
(a) F. Freeman, Chem. Rev., 1975, 75, 439–490 CrossRef CAS;
(b) K. Murai, A. Nakamura, T. Matsushita, M. Shimura and H. Fujioka, Chem.–Eur. J., 2012, 18, 8448–8453 CrossRef CAS PubMed.
- R. Van Lommel, J. Bock, C. G. Daniliuc, U. Hennecke and F. De Proft, Chem. Sci., 2021, 12, 7746–7757 RSC.
- T. K. Beng, J. Fessenden, K. Quigley, J. Eichwald and J. Zesiger, New J. Chem., 2022 Search PubMed.
|
This journal is © The Royal Society of Chemistry 2022 |