DOI:
10.1039/D2RA00724J
(Review Article)
RSC Adv., 2022,
12, 15885-15909
Recent advances of carbonyl olefination via McMurry coupling reaction
Received
2nd February 2022
, Accepted 7th May 2022
First published on 26th May 2022
Abstract
McMurry coupling reaction utilizes the low-valent titanium reagents and carbonyl compounds to produce olefins. The wide synthetic application of McMurry reagents in intermolecular and intramolecular coupling reactions, tandem coupling reactions, and keto ester coupling reactions of carbonyl compounds for the last five years have been reviewed. The resulting coupling reaction produces natural and non-natural products, including strained olefins and unusual molecules as a candidate for nanomaterials, pharmaceuticals, electronic materials, and so forth. The advantages, scope, and limitations along with the improvement of the McMurry coupling reaction, including the addition of high functional group compatibility, McMurry reagents substitution, and several other treatments, have also been discussed.
1. Introduction
In organic synthesis, the formation of olefin is essential to create numerous natural products and non-natural products that can be applied in health, industry, functional materials, etc. Several methods continue to be explored and developed in order to obtain an olefination reaction that is able to control the stereoselectivity, regioselectivity, and chemoselectivity of the reaction. Some of the methods are Horner–Wadsworth–Emmons reaction, Wittig reaction, Julia–Kocienski olefination, and Tebbe olefination.1,2 Other olefination reactions on a catalytic scale that are worth mentioning, such as olefin metathesis (e.g., Grubbs metathesis) and cross-coupling reactions (e.g., Heck reaction), continue to be known, studied and developed, and are still widely applied.
In 1973, the formation of olefin via reductive coupling of carbonyl compounds using low-valent titanium was discovered by Tyrlik with Wolochowicz and Mukaiyama et al.3,4 Mukaiyama et al. reported the reductive coupling of ketones and aromatic aldehyde by utilizing the TiCl4–Zn system. The result of the reductive coupling of acetophenone and benzaldehyde was successful conversion into olefin and pinacol.4 Due to the formation of pinacol, Mukaiyama et al. suggested that the metallopinacolate occurs as an intermediate during the reductive coupling reaction. On the contrary, Tyrlik and Wolochowicz proposed that tetramethylene was obtained through carbene species that occur during the deoxygenation of acetone.3 Due to the absence of by-products from the reductive coupling reaction, the presence of intermediates prior to the formation of carbene species cannot be ascertained. The proposed mechanisms of carbonyl olefination via reductive coupling by Tyrlik with Wolochowicz and Mukaiyama et al. can be seen in the (Fig. 1). Later in 1974, another formation of olefin via reductive coupling of carbonyl compounds, including aliphatic substrates has been developed by McMurry and Fleming.5 This reaction, known as McMurry reaction, utilizes low-valent titanium reagents and carbonyl compounds to produce olefins. In this experiment, McMurry and Fleming introduced the TiCl3/LiAH4 system (known as McMurry reagent) which works efficiently in reductive coupling reactions involving aromatic and aliphatic ketones. The success of McMurry and Fleming in isolating pinacol supports the proposed mechanism by Mukaiyama et al. which suggested the presence of metallopinacolate as an intermediate in the reductive coupling reaction.
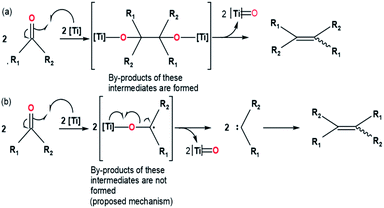 |
| Fig. 1 McMurry coupling involving a metallopinacolate intermediate mechanism proposed by Mukaiyama et al. (a) and by Tyrlik and Wolochowicz (b).3,4 | |
Continuing the previous research, McMurry and coworkers began to improve the procedures for the reductive coupling reaction. Various McMurry system, namely TiCl3/K,6,7 TiCl3/Li,7 and TiCl3(DME)1,5/Zn–Cu8 were tested to find the best system for preparing the low-valence titanium reagent. Other systems were also tested and reported by another researcher, such as TiCl4/Zn/pyridine,9 TiCl3/C8K,10,11 TiCl4/C8K/pyridine,10 and TiCl4/Mg (Hg)/pyridine.10 Various uses of organometals other than titanium for carbonyl coupling reactions such as zirconium, hafnium, niobium, tantalum, chromium, molybdenum, tungsten, iron, manganese, rhenium, lanthanide, and actinide groups have also been explored and briefly summarized by Kahn and Rieke.12 In addition, the scope and limitations of the McMurry reaction such as the compatibility of functional groups with low-valence titanium and some of its synthetic applications have also been reported and briefly summarized by McMurry.13
Nowadays, McMurry coupling reaction is widely utilized in the field of biochemistry, materials science, organic synthesis, and organometallic synthesis. A numerous carbonyl substrates including aldehyde, keto-esters, acylsilanes, oxoamides can be coupled efficiently, via intermolecular or intramolecular McMurry coupling reaction to construct several natural products and non-natural products including strained olefins and unusual molecules. This type of reaction has been applied in the synthesis of loratadine as an antihistamine drug to relieve symptoms of allergies, prevent motion sickness and treatment for insomnia, the synthesis of tamoxifen as an antitumor agent.14 McMurry coupling reaction has also been used to create material such as polycyclic aromatic hydrocarbons (PAHs) as a candidate of light emitting diodes, transistors, and solar cells.15
However, the potential of McMurry coupling reaction is now less utilized due to a statistical mixtures of products specifically an intermolecular reaction involving two different carbonyl compounds, non-stereospecific product, formation of pinacol as a side product,16 and undesirable pinacol rearrangement (Fig. 2). McMurry coupling reaction is also limited by substrate's structural requirement such as aryl ketones with aryl ketones, aryl ketones with aliphatic ketones, diaryl ketones with diaryl ketones. Moreover, this reaction also requires prolonged time for substrate deoxygenation and high temperatures. Therefore, many things still need to be improved from the McMurry coupling reaction. Another problem related to the McMurry reaction that is important to note is the stoichiometric amount of titanium salts and reductants used in reaction, resulting a lot of waste. This stoichiometric problem followed by the extreme conditions of McMurry reaction prompted the researchers to develop transition-metal-catalyzed deoxygenative coupling reactions under mild conditions. In 1995, Fürstner and Hupperts succeeded in designing a catalytic deoxygenation coupling reaction using the TiCl3/Zn system with the addition of excess chlorosilane (e.g., (TMS)Cl, (EtO)3SiCl, (i-Pr)3SiCl, ClSi(Me)2CH2CH2Si(Me)2Cl).17 This encounter is crucial considering the addition of chlorosilane can reduce the amount of stoichiometric titanium salt used to 5–10% mol with a high-yield synthesis.17 Excess chlorosilane can also be removed easily in vacuo, so complex workup of the reaction mixtures is not necessary. The Cp2TiCl2 catalytic system was also applied by Diéguez et al. in carrying out carbonyl olefination reactions, 1,2-diol deoxygenation, and alcohol deoxygenation.18 Other catalytic system such as TiCl4/Yb,19 TiCl4/Sm,19 TiCl4/Dy,19 [Ir(FCF3ppy)2dtbpy]PF6/B2pin2,20 [Rh2(OAc)4],21 and other organometals which require conversion of carbonyl into hydrazone have also been explored and briefly summarized by Asako and Ilies.22
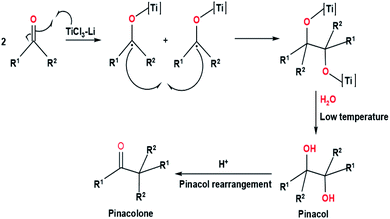 |
| Fig. 2 Formation and rearrangement of pinacol as a side product. | |
Many reviews have been discussing the latest in McMurry reaction such as the 2013 review by Takeda in Organic Reactions and the book “Modern Carbonyl Olefination: Methods and Applications”.23,24 In this review, we are summarizing and analyze the information, for the latest five years, related to the McMurry reaction in several reviews, which consists of the type of reaction, general procedure, reagents, functional group compatibility, additives, and its application in the synthesis of natural and non-natural products. In addition, we will also discuss the advantages and disadvantages of the McMurry reaction, also the treatments to minimize the drawbacks of the McMurry reaction.
2. Coupling reagents and general procedure
2.1 Coupling reagents
2.1.1 Substrates. In the McMurry reaction, the substrates must contain one or more carbonyl functional groups. Numerous carbonyl substrates such as aldehyde, ketone, oxoamide, acylsilanes, conjugated enones, acyloin, and keto-ester can be coupled to form olefins. The addition of functional groups to the substrates such as aryl, alcohol, alkene, halide, ester, ether, alkyl, phospholes, amine, sulfide, and others could either activate or deactivate the adjacent carbonyl that will be discussed in the latter section.13
2.1.2 Sources of low-valent metals. Low-valent metals have a predominant role in the McMurry reaction. The substrate coupling was induced by a single electron transfer from low-valent metal to the carbonyl groups. Due to the high reducing potential and strong oxophilicity of low-valent metals, the metal ion causes the carbonyl groups on the substrate to move closer to one another and easily deoxygenated. The common low-valent metal in the McMurry reaction is zero-valent titanium. Zero-valent titanium was prepared by reducing titanium tetrachloride or titanium trichloride with strong reducing agents. Several metals have been explored in the McMurry coupling reaction. Some of the metals are vanadium,25 tungsten,26,27 molybdenum,27 niobium,28 and lithium.29 In 1982, Geise et al. reported using cobalt, nickel, iron, copper, chromium, zinc, manganese, tin, hafnium, and tantalum in benzophenone coupling experiments with lithium aluminum hydride as a reducing agent shows negative results.29 Recently, the group of Nakamura has shown that active low-valent metal was effectively prepared by reducing hafnium tetrachloride and zirconium tetrachloride with zinc dust for the coupling of two aryl ketones molecules.30 Additionally, Moxter et al. has successfully performed the “Sila-McMurry” coupling reaction using disilicon hexachloride in benzene-d6 solution.31
2.1.3 Solvents. Tetrahydrofuran (THF) is the most widely used solvent in McMurry reaction because of its ability to dissolve intermediate pinacolate complexes, facilitate the electron transfer process, and maintain its form without being reduced under the McMurry reaction conditions. Another solvent as a substitute to THF solvent is dimethoxyethane (DME). DME is often used in organometallic reactions, due to its ability to form bidentate ligands with metal cations. This solvent is suitable for high-temperature reactions. McMurry has reviewed the reduction of TiCl3(DME)1.5 with Zn–Cu generates active zero-valent titanium.13
2.1.4 Reducing agents. Activated zinc metal or zinc-copper is a common reducing agent used for McMurry reaction. Zinc-copper has been preferred because copper can enhance the reactivity of zinc by weakening the zinc–zinc bond. Several other reducing agents that have been used are magnesium-mercury amalgam,32 lithium aluminum hydride,32 potassium metal,33 and lithium metal.33 The use of potassium or lithium metal has rarely been applied in the McMurry reaction due to its flammable and explosive characteristics. Based on the research conducted by Harit and Ramesh regarding the synthesis of aminocylitols via pinacol coupling reaction, the use of magnesium–mercury amalgam as a reducing agent in THF was unsuccessful, whereas lithium aluminum hydride in DME produced aminocylitols with 10% yields. By using the zinc–copper couple in THF, the product was successfully synthesized with 63% yields.32
2.1.5 Other functional reagents. Additional compatible reagents are used to enhance the carbonyl olefination process. Pyridine is used as a base to neutralize excess acid from metal ions. Potassium carbonate or sodium carbonate and HCl are used as a quenching reagent to deactivate any unreacted reagents.
2.2 General procedure
The McMurry reaction is completed in two steps: reduction of TiCl4 to form low-valent titanium and deoxygenation of carbonyl substrates. Under an inert atmosphere, a suspension of low-valent titanium was prepared from the dropwise injection of TiCl4 into a two or three-necked round-bottomed flask containing an amount of reducing agents in a dry THF or DME at 0 °C. The mixture was refluxed for several hours. After cooling the mixture to 0 °C up to −5 °C, the substrates and a small amount of pyridine were added to the reaction mixture and refluxed for several hours or up to overnight. The solution was cooled at room temperature and quenched by the dropwise addition of a quenching reagent. Furthermore, the product can be purified by extraction, column chromatography, or recrystallization.
3. Reaction mechanism
In general, two reaction steps occur in the McMurry coupling reaction (Fig. 3). The first step is carbonyl substrates reduction by a single electron transfer from titanium to produce ketyl radical compounds that can be dimerized to form intermediate pinacols (metallopinacolate). By decreasing the reaction temperature from reflux to 0 °C, the intermediate pinacols can be isolated in a high yield.13 The second step involves the deoxygenation of metallopinacolate to form olefins by maintaining the reflux temperature.
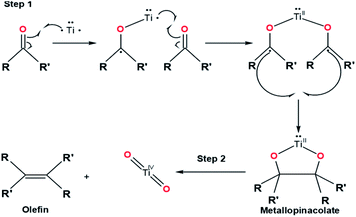 |
| Fig. 3 General steps in the McMurry coupling reaction. | |
Although the McMurry coupling reaction was discovered more than four decades ago, only a few details regarding the mechanism of the McMurry coupling reaction were known. By this time, the mechanism of the McMurry reaction can only be explained through a hypothetical approach. Four hypothetical pathways consist of paths A1, A2, B, and C related to McMurrys's reaction mechanism have been illustrated in Fig. 4.34
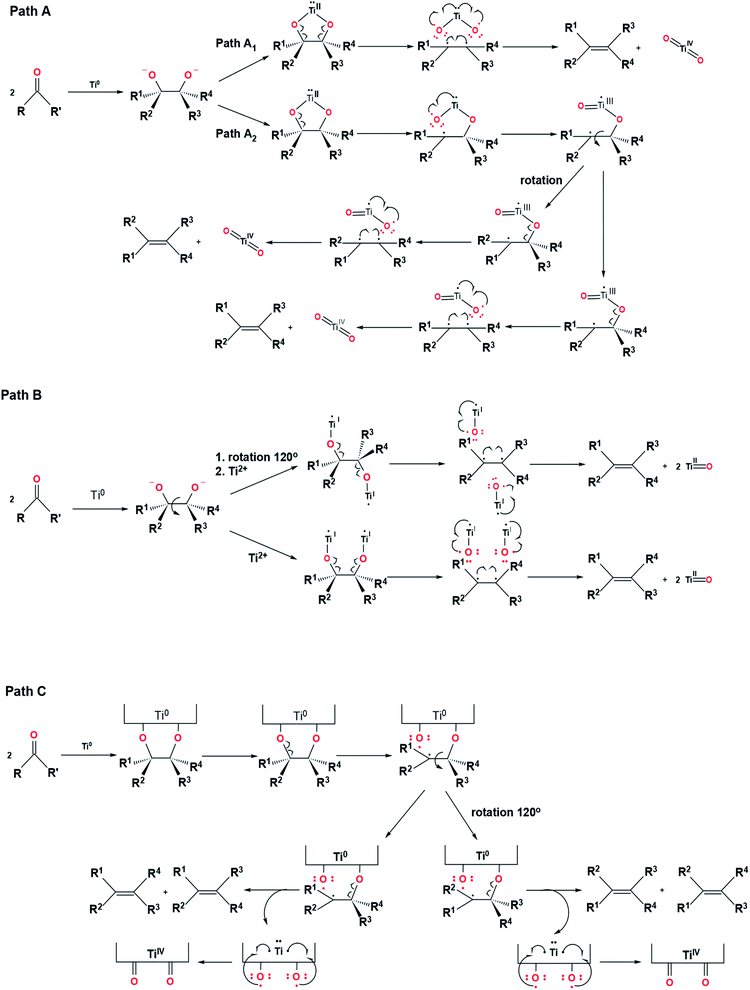 |
| Fig. 4 Four hypothetical pathways related to McMurry's reaction mechanism.34 | |
In path A, the metallopinacolate forms a five-membered ring structure with two oxygen atoms bound to the same zero-valent titanium. The homolytic cleavage of C–O bonds in metallopinacolate, oxidation of titanium, and the formation of the C–C bond were carried out simultaneously through the concerted process (path A1) or non-concerted process (path A2). The difference between path A1 and path A2 lies in the homolytic cleavage process of C–O bonds in metallopinacolate. In path A1, the two C–O bonds broke concurrently, whereas path A2 describes the cleavage of two C–O bonds at different times. Hypothetically, path A is a regioselective reaction due to the presence of a titanium ion bonded to two oxygen atoms. However, based on the experiment reported by McMurry et al., shows that the coupling of pentanal to form 5-decene (70% trans, 30% cis) via McMurry coupling reaction is not a regioselective reaction (Fig. 5).7 Hence, another path to describe the McMurry coupling reaction is needed.
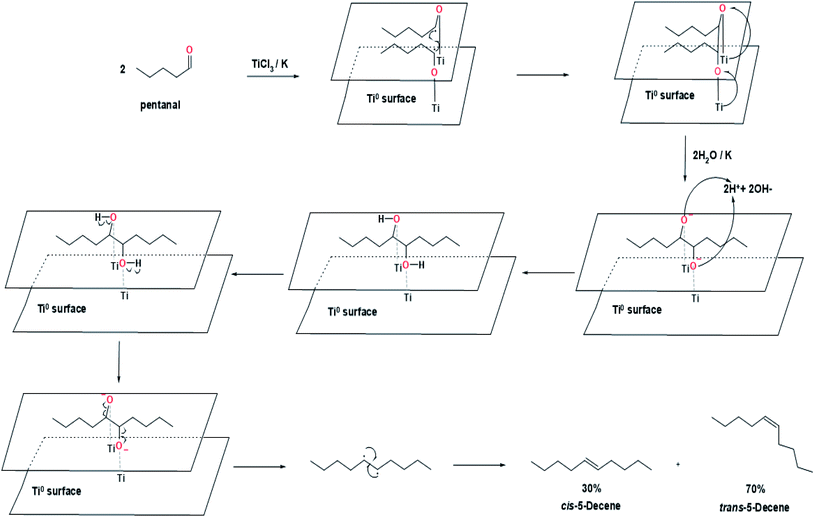 |
| Fig. 5 Proposed mechanism for the synthesis of cis-5-decene and trans-5-decene from pentanal.7 | |
In path B, the two oxygen atoms in the intermediate pinacols bonded to different titanium. This path does not involve the five-membered ring structure in the intermediate. The homolytic cleavage of C–O bonds in path B adopts a non-concerted process. The rotation of C–C bonds occurred due to the high-density electrons in the σ bond between two carbon nuclei. In 1978, McMurry et al. also reported an experiment related to the synthesizing of 1,2,3,4,5,6,7,8-octahydronaphthalene from cis-9,10-decalindiol and trans-9,10-decalindiol via intramolecular reaction using TiCl3/K system to determine the feasibility of path B (Fig. 6).7 The use of cis-9,10-decalindiol as a substrate to produce 1,2,3,4,5,6,7,8-octahydronaphthalene was successful with 80% yields, while trans-9,10-decalindiol did not produce 1,2,3,4,5,6,7,8-octahydronaphthalene due to the lack of energy to cleave the carbon and oxygen bonds.7 These results undermine the plausibility of path B in explaining the McMurry coupling reaction mechanism.
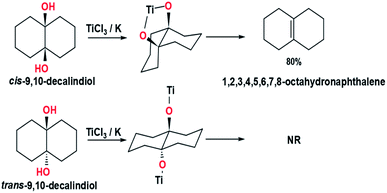 |
| Fig. 6 Synthesis 1,2,3,4,5,6,7,8-octahydronaphthalene from cis-9,10-decalindiol (top) and trans-9,10-decalindiol (bottom).7 | |
The last hypothetical pathway to explain the McMurry coupling reaction mechanism is path C. In path C, the two oxygen atoms from metallopinacolate bonded to the titanium(0) surface, which adopts heterogeneous catalysis process. The difference between path C and path A lies in the number of titanium involved in the reaction. Path C involves two titanium atoms bonded to two oxygen atoms without forming a five-ring intermediate, but the Ti–O bonds could break simultaneously. Path C also allows two oxygen atoms to bind to different titanium surfaces. This flexible mechanism is similar to a combination of path A and path B, which cover each other's shortcomings. The isomeric reaction mechanism of decalindiol to produce octahydronaphthalene can be explained through path A2 and path C. In 1978, McMurry et al. also mentioned an experiment related to the synthesis of 2-bornene via reductive coupling of cis-camphanediol and a 70
:
30 mixtures of trans-camphanediol 1 with trans-camphanediol 2 using TiCl3/K in THF to determine which pathway is more feasible to describe the McMurry coupling reaction mechanism (Fig. 7).7 If the reaction involves metallopinacolate intermediates with a five-membered ring structure (path A2), the reduction of cis-camphanediol will be faster than the reduction of trans-camphanediol. Meanwhile, if the reaction goes through path C, both cis- and trans-camphanediol will reduced at equal rates. Based on the experimental data in Table 1 shows the cis-camphanediols and trans-camphanediols were reduced at approximately equal rates.7 Therefore, the McMurry coupling reaction is more likely to occur via a heterogeneous catalysis process on an active titanium surface (path C).
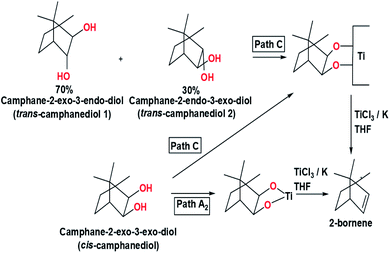 |
| Fig. 7 Synthesis of 2-bornene from cis-camphanediol and trans-camphanediol.7 | |
Table 1 Result of synthesis 2-bornene from cis-camphanediol and trans-camphanediol7
Run |
Time (h) |
Yield of 2-bornene (%) |
cis-Camphanediol |
trans-Camphanediol 1 + trans-camphanediol 2 (70 : 30) |
1 |
0.5 |
51 |
29 |
2 |
1 |
76 |
33 |
3 |
5 |
81 |
60 |
4. Functional group compatibilities
When carrying out the McMurry coupling reaction, the selection of substrates-containing functional groups bonded to the carbonyl needs to be considered. Some functional groups are incompatible with the McMurry reaction due to the ability of low-valent titanium to reduce certain functional groups. Characterization of olefin products can be performed to determine whether the functional group has been transformed into another group during the reaction process. This section discusses several functional groups classified as compatible, semi-compatible, and incompatible with the McMurry coupling reaction.
4.1 Compatible functional groups
Functional groups that are compatible with the McMurry coupling reaction is a group that is not easily reduced and have an ability to maintain its form under reaction conditions.
4.1.1 Acetal. Acetals are prone to hydrolysis in acidic conditions. This could be problematic since metal ions contribute to the acidic atmosphere in the reaction can cause the acetal groups to be hydrolyzed during workup. The addition of aqueous sodium carbonate, aqueous ammonia, pyridine, or triethylamine could prevent the hydrolysis process by keeping the reaction in an alkaline atmosphere. Several experiments have applied the McMurry coupling reaction in combining the acetal functional group substrate (Fig. 8), including the synthesis of olefin, 2, through the intramolecular coupling reaction of carbonyl substrate, 1, with the presence of acetal (Table 2).11 Another unpublished experiment conducted by McMurry and Matz showed that tetrahydropyran ether, 4, were obtained from the intermolecular coupling of two acetals, 3.13 Moreover, Sander and Dehmlow successfully synthesized 1-(cis-4′,5′-dihydroxycycloheptylidene)-cis-4,5-dihydroxycycloheptane, 6, through McMurry coupling of the cis-4,5-dibenzyloxycycloheptanone compound, 5.35
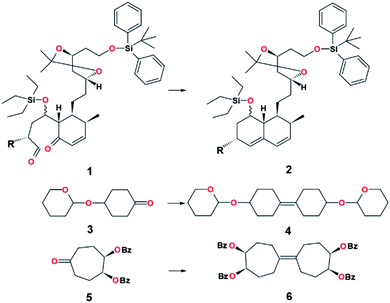 |
| Fig. 8 McMurry coupling reaction of carbonyl substrate containing acetal groups. | |
Table 2 McMurry coupling reaction of carbonyl substrate containing acetal groups
Substrate |
Product |
Source of metal |
Reducing agent |
Solvent |
Yield (%) |
Ref. |
1 |
2 |
TiCl3 |
C8K |
DME |
86 |
11 |
3 |
4 |
TiCl3(DME)1.5 |
Zn–Cu |
DME |
97 |
13 |
5 |
6 |
TiCl3 |
LiAlH4 |
THF |
98 |
35 |
4.1.2 Alcohol (saturated alcohol complex and some aromatic alcohol). Some experiments have tested the compatibility of the alcohol groups in the McMurry reaction (Fig. 9). Alcohol groups such as saturated alcohols for large molecules or common aromatic alcohols are inert to low-valent titanium. Saturated alcohol such as cholesterol, 7, cannot be coupled via McMurry reactions due to the steric effect that makes the oxygen atoms hardly interact with the low-valent titanium surface.13 In 2003, Yu and Forman successfully synthesized (E,Z)-4-hydroxytamoxifen, 10, through the McMurry coupling reaction (Table 3).36 The E and Z isomers were separated by a selective crystallization method to obtain (Z)-4-hydroxytamoxifen as a candidate of estrogen receptor modulator. The hydroxy complex, 13, was successfully synthesized by Top et al. as a precursor in the manufacture of hydroxyferrocifen compounds or ferrocifen metabolites.37 Another ferrocenyl analogs, namely 3-ferrocenyl-4-(4-hydroxyphenyl)-hex-3-ene, 16, have also been synthesized by coupling propionylferrocene, 14, with 4-hydroxypropiophenone, 15, via McMurry reaction.38
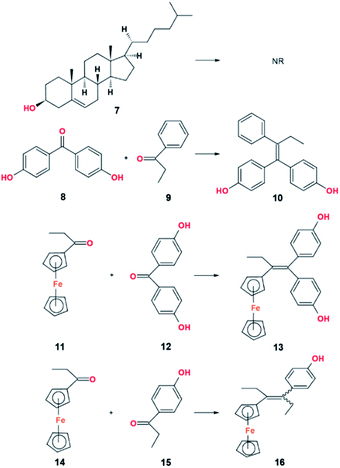 |
| Fig. 9 McMurry coupling reation of carbonyl substrate containing saturated alcohol and aromatic alcohol groups. | |
Table 3 McMurry coupling reaction of carbonyl substrate containing saturated alcohol and aromatic alcohol groups
Substrate |
Product |
Source of metal |
Reducing agent |
Solvent |
Yield (%) |
Ref. |
The measured yield was the yield after the precursor undergo alkylation with Cl(CH2)nNMe2 with the addition of NaOH, acetone, and HCl. |
7 |
NR |
LVT |
— |
THF |
— |
13 |
8 & 9 |
10 |
TiCl4 |
Zn |
THF |
91 |
36 |
11 & 12 |
13 |
TiCl4 |
Zn |
THF |
51a |
37 |
14 & 15 |
16 |
TiCl4 |
Zn |
THF |
30 |
38 |
4.1.3 Halide. The halide functional groups are the groups that are rich in electrons. Their electron-rich nature and compliance with the octet rule make the halide groups less likely to accept electrons from the low-valent titanium. Generally, organohalides are not reduced by low-valent titanium. For example, McMurry couplings of chloropropionylferrocene, 17, with 4,4′-dihydroxybenzophenone, 18, give the corresponding ClFcdiOH, 19.38 The intermolecular coupling via McMurry reaction of 4-bromobenzophenone, 20, yields 1,2-bis(4-bromophenyl)-1,2-diphenylethenes, 21.39 Synthesis 1,2-di(4-bromophenyl)-1,2-di(1,3,5-triphenylbenzene-4′-yl)ethylene, 23, from 4-bromo-4’-[(3,5-diphenyl)phenyl]benzophenone, 22, via McMurry coupling reaction has been successfully carried out.40 Additionally, synthesis of 1,6-dihalo-1,3,5-hexatrienes, 25, can also be obtained from the β-haloacrylaldehydes derivatives, 24.41 Another McMurry reaction was shown in the coupling of 2-iodobenzaldehyde, 26, to produce compound 27 with 55% yield (Table 4).42 Based on the information obtained through the literature shows that the halide functional groups in the product remain the same as the functional groups on the substrate (Fig. 10).
Table 4 McMurry coupling reaction of carbonyl substrate containing halide groups
Substrate |
Product |
Source of metal |
Reducing agent |
Solvent |
Yield (%) |
Ref. |
17 & 18 |
19 |
TiCl4 |
Zn |
THF |
47 |
38 |
20 |
21 |
TiCl3 |
LiAlH4 |
THF |
73 |
39 |
22 |
23 |
TiCl4 |
Zn |
THF |
24 |
40 |
24 |
25 |
TiCl4 |
Zn |
DME |
79 |
41 |
26 |
27 |
TiCl4 |
Zn |
THF |
55 |
42 |
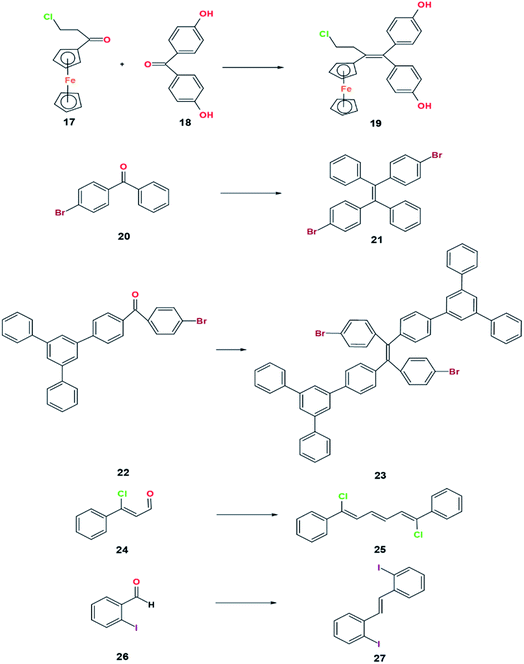 |
| Fig. 10 McMurry coupling reaction of carbonyl substrate containing halide groups. | |
4.1.4 Substituted silane. Substituted silanes such as vinyl and alkyl silane do not react with low-valent titanium (Fig. 11). In 1994, Roth and Horn reported that the intermolecular coupling of the tetraalkylsilane substrate, 28, yields an olefin containing two tetraalkylsilane groups, 29.43 Based on the experiment conducted by Disanavaka and Weedon, substituted silane compounds, 31, were successfully synthesized through the intramolecular reaction of, 30.44 The results showed that the silane product did not undergo any further transformation under reaction conditions. The reductive coupling of bifunctional aldehyde, 33, has also been performed by Paquette et al. to produce trans-1,2-bis[1-(trimethylsilyl)cyclopropyl]ethylene, 32.45 The first intermolecular and intramolecular McMurry coupling reaction of aroyltrimethylsilanes and bis-aroylsilane, 34 and 36, to produce substituted 1,2-bis(trimethylsilyl)ethene derivatives, 35 and 37, has also been performed successfully by Fürstner et al.46 The stability of substituted silane under reaction conditions provides an opportunity to synthesize olefins containing substituted silane groups through McMurry coupling reactions (Table 5).
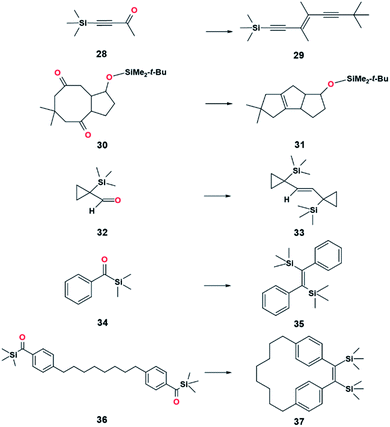 |
| Fig. 11 McMurry coupling reaction of carbonyl substrate containing substituted silane groups. | |
Table 5 McMurry coupling reaction of carbonyl substrate containing substituted silane groups
Substrate |
Product |
Source of metal |
Reducing agent |
Solvent |
Yield (%) |
Ref. |
28 |
29 |
TiCl4 |
Zn |
THF |
41 |
43 |
30 |
31 |
TiCl3 |
K |
THF |
38 |
44 |
32 |
33 |
TiCl3 |
Li |
DME |
53 |
45 |
34 |
35 |
TiCl3 |
Na on Al2O3 |
DME |
67 |
46 |
36 |
37 |
TiCl3 |
Na on Al2O3 |
DME |
49 |
46 |
4.1.5 Sulfide and thiophene. The compatibility of the sulfide group is represented by the coupling products, 39, of diketo-sulfides, 38, via intramolecular McMurry coupling reaction (Table 6).47 Other experiments have involved thiophene groups coupling reactions to synthesize various olefins. For example, the dimer thienylenevinylene or 1,6-dithienylhexa-1,3,5-trienes, 42a–b and 43, were obtained through McMurry coupling of conjugated ketones, 40a–b and 41.48 Additionally, DTEs or bridged dithienylethylenes with methyl substituents, 45, can be obtained through the intermolecular reductive coupling of 4-methyl-4,5-dihydro-6H-cyclopenta[b]thiophen-6-one, 44.49 All these reactions have been illustrated in Fig. 12.
Table 6 McMurry coupling reaction of carbonyl substrate containing sulfide and thiophene group
Substrate |
Product |
Source of metal |
Reducing agent |
Solvent |
Yield |
Ref. |
38 |
39 |
TiCl4 |
Zn |
THF |
84% |
47 |
40a |
42a |
TiCl4 |
Zn |
THF |
89% |
48 |
40b |
42b |
TiCl4 |
Zn |
THF |
48% |
48 |
41 |
43 |
TiCl4 |
Zn |
THF |
84% |
48 |
44 |
45 |
TiCl4 |
Zn |
THF |
83% |
49 |
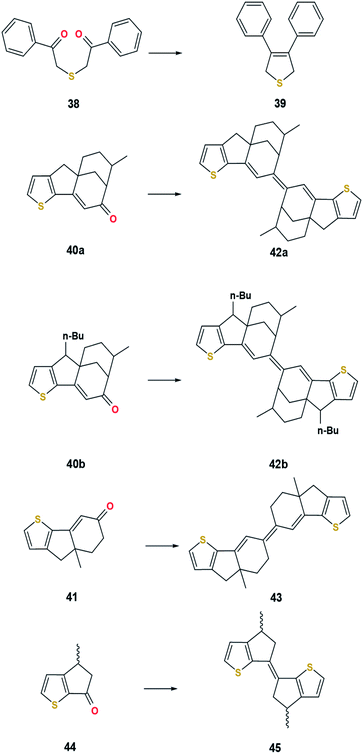 |
| Fig. 12 McMurry coupling reaction of carbonyl substrate containing sulfide and thiophene groups. | |
4.1.6 Ether. The compatibility of ether with the McMurry reaction can be depicted by the coupling products, 47, from diether substrate, 46.50 Additionally, McMurry coupling of two calixanthone, 48, was successfully performed by Agbaria and Biali to form dixanthylene, 49.51 The results of both experiments (Fig. 13) show that the ether groups are inert to low-valent titanium (Table 7).
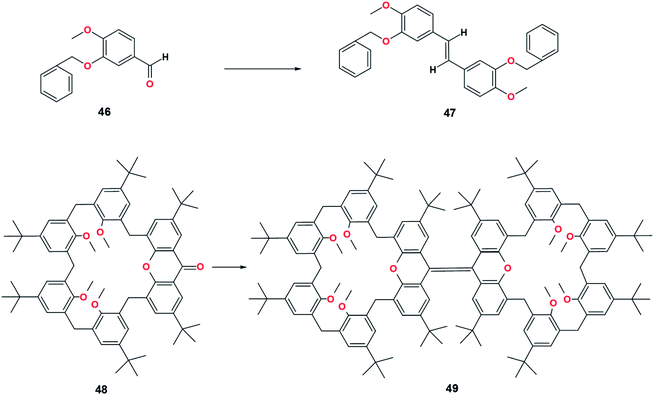 |
| Fig. 13 McMurry coupling reaction of carbonyl substrate containing ether groups. | |
Table 7 McMurry coupling reaction of carbonyl substrate containing ether groups
Substrate |
Product |
Source of metal |
Reducing agent |
Solvent |
Yield (%) |
Ref. |
46 |
47 |
TiCl3 |
Zn–Cu |
DME |
97 |
50 |
48 |
49 |
TiCl4 |
LiAlH4 |
THF |
18 |
51 |
4.1.7 Phospholes. Until recently, the application of the coupling reaction of carbonyl substrates containing phosphorus groups via the McMurry reaction was quite rare. The only experiments showing that phosphole is compatible under McMurry conditions (Fig. 14) are the synthesis of 1,2-bis(phosphacymantrenyl)alkenes, 51, from McMurry coupling of 2-acylphosphacymantrenes, 50,52 and 1,2-bis(2-phospholyl)alkenes, 53, from trifunctional substrates, 52 (Table 8).53
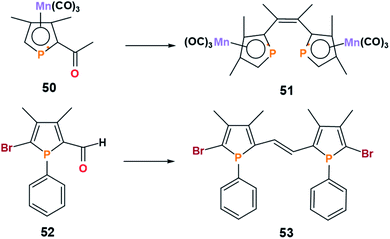 |
| Fig. 14 McMurry coupling reaction of carbonyl substrate containing phospholes groups. | |
Table 8 McMurry coupling reaction of carbonyl substrate containing phospholes group
Substrate |
Product |
Source of metal |
Reducing agent |
Solvent |
Yield (%) |
Ref. |
50 |
51 |
TiCl4 |
Zn |
THF |
27 (meso) |
52 |
45 (rac) |
52 |
53 |
TiCl4 |
Zn |
THF |
68 |
53 |
4.2 Semi-compatible functional groups
All the semi-compatible groups can be reduced slowly by low-valent titanium. Its resistance to low-valent titanium depends on the reaction conditions that can be adjusted by increasing or decreasing the reaction time, diluting, concentrating the mixture, or increasing the reaction temperature.
4.2.1 Amide. The compatibility of the amide functional group has been depicted in Fig. 15. The transformation of amide groups can be prevented by not extending the reaction time and not utilizing LiAlH4 as a reducing agent during the coupling reaction. In 1985, Seijas et al. successfully applied the McMurry reaction in coupling substrates containing amide groups, namely synthesis of stilbene derivatives, 56, from urethane, 54, and benzaldehyde, 55.54 Moreover, McMurry coupling of amide substrates, 57, has also been performed to produce 11-indenoisoquinolines, 59 (Table 9).55
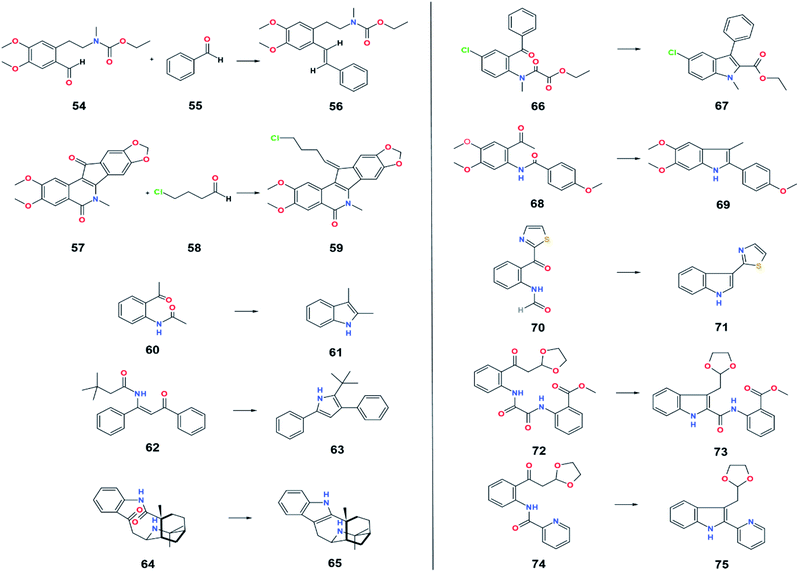 |
| Fig. 15 McMurry coupling reaction of carbonyl substrate containing amide and oxoamide groups. | |
Table 9 McMurry coupling reaction of carbonyl substrate containing amide and oxoamide group
Substrate |
Product |
Source of metal |
Reducing agent |
Solvent |
Yield (%) |
Ref. |
54 & 55 |
56 |
TiCl3 |
Li |
1,2-DME |
55 |
54 |
57 & 58 |
59 |
TiCl4(THF)2 |
Zn |
THF |
43 |
55 |
60 |
61 |
TiCl3 |
Graphite |
THF |
70 |
56 |
62 |
63 |
TiCl3 |
Zn |
DME |
60 |
57 |
64 |
65 |
TiCl3 |
Zn |
THF |
75 |
58 |
66 |
67 |
LVT |
Graphite |
DME |
94 |
59 |
68 |
69 |
TiCl3 |
C8K |
DME |
66 |
60 |
70 |
71 |
TiCl3 |
Zn |
DME |
71 |
61 |
72 |
73 |
TiCl3 |
C8K |
THF |
— |
62 |
74 |
75 |
TiCl3 |
C8K |
THF |
57 |
62 |
Although some experiments have demonstrated the resistance of the amide group to the McMurry reaction conditions, Fürstner et al. has reported the transformation of oxoamide, 60 and 62, to produce various indole and pyrrole derivatives, 61 and 63, via intramolecular coupling reaction.56,57 Aside from synthesizing non-natural compounds, McMurry coupling of amide substrates has also been applied to synthesize biologically active compounds such as (+)-aristoteline, 65, as a relaxation-inducing agent,58 salvadoricine, 67, as an antibiotic and antiseptic agent in Salvadora persica,59 zindoxifene analogs, 69, as an anti-cancer agent,60 and camalexin, 71, as an anti-bacterial and anti-pathogenic agent in Arabidopsis thaliana.61 Amide cyclization through the McMurry reaction is also applied in the synthesis of certain materials, 73 and 75, to produce secofascaplysin, which belongs to the sponge Fascaplysinopsis reticulata and indolopyridocolines that can be found in the bark of Gonioma kamassi.62
4.2.2 Carboxylic acid. There is little information regarding experiments testing the resistance of carboxylic acid groups to the McMurry reaction conditions (Table 10). In 1981, Geise et al. reported that the coupling reaction of benzoic acid, 76, resulted in an assortment of products with different functional groups, 77–80 (Fig. 16).63 One or both oxygen atoms from carboxylic acid or its salts can be abstracted by low-valent titanium to yields either alkyne or diketone. Partial reduction of alkyne using hydrogen gas with a Lindlar catalyst via syn addition mechanism or sodium with ammonia via anti-addition will produce olefins. According to McMurry's hypothesis, the transformation of the carboxylic acid group can be prevented by carrying the reaction in a relatively swift period.13 Another way to prevent the reduction of the carboxylic acid group is by transforming the carboxylic acid groups into a specific group listed in Table 11. Inactivation of carboxylic acid groups by converting them to esters is less desirable because esters react slowly with low-valent titanium. Further research is needed to ensure that the protected carboxylic acid is compatible with the McMurry reaction.
Table 10 McMurry coupling reaction of carbonyl substrate containing carboxylic acid group
Substrate |
Product |
Source of metal |
Reducing agent |
Solvent |
Yield (%) |
Ref. |
76 |
77 |
TiCl3 |
LiAlH4 |
THF |
39 |
63 |
76 |
78 |
TiCl3 |
LiAlH4 |
THF |
17 |
63 |
76 |
79 |
TiCl3 |
LiAlH4 |
THF |
12 |
63 |
76 |
80 |
TiCl3 |
LiAlH4 |
THF |
5 |
63 |
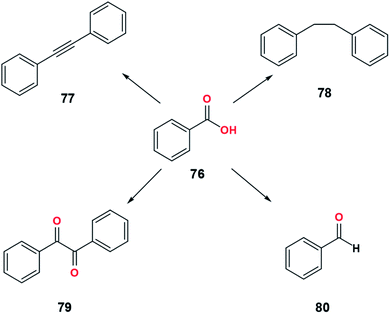 |
| Fig. 16 McMurry coupling reaction of carbonyl substrate containing carboxylic acid groups. | |
Table 11 Protection and deprotection of carboxylic acid group
Transformation (deactivation state) |
Deprotection (reactivation) |
High temperature required. |
Methyl ester |
Acid or base |
Benzyl ester |
Hydrogenolysis |
Silyl ester |
Organometallics, acid, and base |
tert-Butyl ester |
Reductants, acid, and base |
Oxazoline |
Strong acid or alkalia |
4.2.3 Ester. In contrast to carboxylic acids, many coupling reactions of carbonyl compounds containing ester groups have been reported (Fig. 17). One example that describes the stability of an ester group against the McMurry reaction is the synthesis of olefin, 82, from an ester substrate, 81, via intramolecular coupling reaction.64 The ester did not undergo a functional group transformation due to the presence of two carbonyls that close to each other. Hence, the intramolecular reaction between the two adjacent carbonyls is preferred rather than carbonyl from the ester. The synthesis of the lasiodiplodine-forming material describes some experiments showing the incompatibility of ester groups with the McMurry reaction, 84,65 and enol ether, 87,66 via intermolecular keto-ester couplings (Table 12).
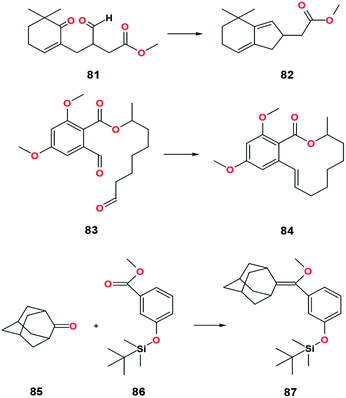 |
| Fig. 17 McMurry coupling reaction of carbonyl substrate containing ester groups. | |
Table 12 McMurry coupling reaction of carbonyl substrate containing ester group
Substrate |
Product |
Source of metal |
Reducing agent |
Solvent |
Yield (%) |
Ref. |
81 |
82 |
TiCl3 |
Zn–Cu |
DME |
52 |
64 |
83 |
84 |
Ti |
Graphite |
DME |
69 |
65 |
85 & 86 |
87 |
TiCl3 |
LiAlH4 |
THF |
41 up to 59 |
66 |
4.2.4 Toluenesulfonate ester. In the field of organic synthesis, toluenesulfonate or tosylate groups are generally used as a protecting group. Although this group is compatible with the McMurry reaction, the existence of a strong nucleophile substituted the group resulting in an undesired product. This group is a good leaving group due to the resonance or electron delocalization of the negative charge on oxygen. One example that demonstrates the compatibility of the tosylate group is the synthesis of the compound, 89, through the intermolecular coupling reaction of difunctional substrates, 88.50 By using TiCl3/Zn–Cu system in THF, the product was successfully synthesized with 64% yield. Neither transformation nor substitution occurs during the McMurry reaction (Fig. 18).
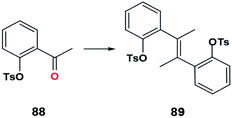 |
| Fig. 18 McMurry coupling reation of carbonyl substrate containing tosylate groups. | |
4.2.5 Nitrile. The nitrile group can be compatible with the McMurry reaction as long as the reaction conditions and the reagent are selected correctly. This group is inert to low-valent titanium but can be reduced rapidly to primary amines in the presence of LiAlH4 with diethyl ether solvent. The reaction's atmosphere should be neither too acidic nor too alkaline because nitriles can be hydrolyzed to carboxylic acids or carboxylic salts in an acid or alkaline atmosphere. Adjusting the pH by adding acid or base reagents in the McMurry reaction is not recommended. In general, adding an acid reagent such as HCl can quench the reaction, whereas an excess base reagent can react with the titanium to form titanium hydroxide deposits. One experiment which shows the inert nature of the nitrile group against low-valent titanium (TiCl3/LiAlH4 system) is the formation of the nitrile compound, 91, as minor products (yield = 15%) that did not undergo a further transformation (Fig. 19).63 In 2018, Mai et al. succeeded in combining a nitrile group substrate to form an unsymmetrical E-alkenes via phosphaalkene and phosphine intermediates as an alternative to the olefination of carbonyl other than the McMurry reaction.67
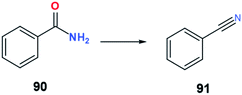 |
| Fig. 19 McMurry coupling reaction of carbonyl substrate resulting nitrile compound as a minor product. | |
4.3 Incompatible functional groups
Incompatible functional groups undergo a direct transformation when reacting with low-valent titanium. It is advisable to use other milder reactions than the McMurry reactions when involving a substrate containing this group. Some examples of non-compatible functional groups other than those described below include halohydrin, enedione, and quinone groups.
4.3.1 Alcohol (allylic alcohol and 1,2-diol). As discussed in the section on the reaction mechanism, hydrolysis of metallopinacolate as an intermediate in the first step of the McMurry reaction yields 1,2-diol compounds. The presence of two adjacent oxygen atoms can be easily abstracted by active titanium, resulting in carbon radicals on the substrate that can interact with the adjacent carbon radicals to form new carbon–carbon bonds. For example, 1,2-diol, 92 and 94, can be deoxygenated to form a new carbon–carbon bond, 93 and 95.7 The addition of 2-dimethoxypropane, boc-ethylidene, moc-ethylidene, and other diol protection agents should prevent the deoxygenation process.68 Further investigation of these protecting agents are needed to test the effect on the synthesized product. Besides 1,2-diol, allylic alcohol is also a group that can be reduced by active titanium. For example, dienes compound, 97, can be obtained from the intermolecular coupling of 2-cyclohepten-1-ol, 96 (Table 13).69 All of the reactions have been illustrated in Fig. 20.
Table 13 Products obtained from the reaction of allylic alcohol and 1,2-diol compounds with low-valent titanium
Substrate |
Product |
Source of metal |
Reducing agent |
Solvent |
Yield (%) |
Ref. |
92 |
93 |
TiCl3 |
K |
DME |
85 |
7 |
94 |
95 |
TiCl3 |
K |
DME |
80 |
7 |
96 |
97 |
TiCl3 |
LiAlH4 |
THF |
87 |
69 |
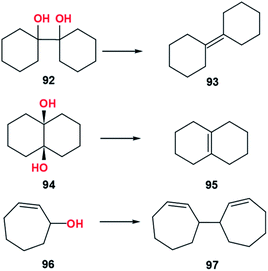 |
| Fig. 20 Products obtained from the reaction of allylic alcohol and 1,2-diol compounds with low-valent titanium. | |
4.3.2 Nitro. The reduction of the nitro group is one of the reactions to obtain a wide variety of functional groups. Several experiments related to the reaction of nitro groups with low-valent titanium have shown the transformation from nitro group to azo, aniline, nitroso, amine, oxime, and amino groups (Fig. 21).63,70–72 The presence of 2 phenyl groups on the substrate, 108, stabilizes the radical anion obtained from the electron transfer of titanium(III) to the nitro group. Reduction of these radical anions produced an oxime, 109, which can be further reduced to form imine by aqueous titanium halide.65 The ability of oxime to be reduced by low valence titanium shows the incompatibility of this group in the McMurry reaction (Table 14).
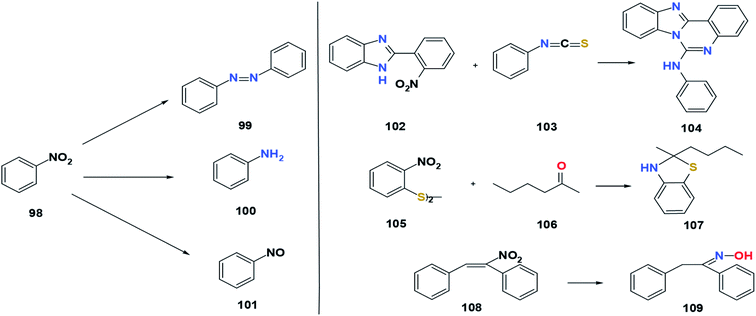 |
| Fig. 21 Products obtained from the reaction of nitro compounds with low-valent titanium. | |
Table 14 Products obtained from the reaction of nitro compounds with low-valent titanium
Substrate |
Product |
Source of metal |
Reducing agent |
Solvent |
Yield (%) |
Ref. |
98 |
99 |
TiCl3 |
LiAlH4 |
THF |
63 |
63 |
98 |
100 |
TiCl3 |
LiAlH4 |
THF |
23 |
63 |
98 |
101 |
TiCl3 |
LiAlH4 |
THF |
5 |
63 |
102 & 103 |
104 |
TiCl4 |
Zn |
THF |
74 |
70 |
105 & 106 |
107 |
TiCl4 |
Sm |
THF |
74 |
71 |
108 |
109 |
TiCl3 |
— |
THF |
62 |
72 |
4.3.3 Sulfoxide. Reduction of sulfoxide using LiAlH4, NaBH4–FeCl3, low valent titanium, or other reducing agents yields a sulfide group. Some experiments related to the transformation of sulfoxides to sulfides have been illustrated in Fig. 22. Coordination of titanium halide with oxygen attacks one of the halide ligands to give the intermediate halogenated species. The other halide anion attacks the intermediate species resulting in a deoxygenated sulfide (Table 15).73–76
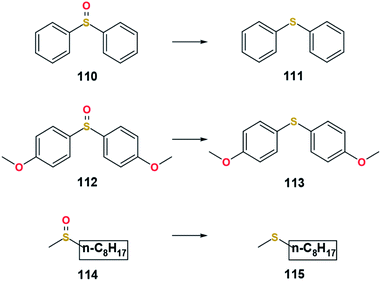 |
| Fig. 22 Products obtained from the reaction of sulfoxide compounds with low-valent titanium. | |
Table 15 Products obtained from the reaction of sulfoxide compounds with low-valent titanium
Substrate |
Product |
Source of metal |
Reducing agent |
Solvent |
Yield (%) |
Ref. |
110 |
111 |
TiCl3 |
— |
MeOH & CHCl3 |
63 |
73 |
112 |
113 |
Cp2TiCl2 |
In |
THF |
92 |
74 |
112 |
113 |
TiCl4 |
In |
THF |
95 |
75 |
114 |
115 |
TiI4 |
— |
CH3CN |
90 |
76 |
5. Effects of additives
The regulation of low-valent titanium activity has been reached by adding an additive compound or changing the reaction conditions. In some instances, additives improve the reactivity of TiClx-reducing agent systems resulting in an enhancement of olefin's formation at high-temperature reactions or, on the contrary, a pinacol at low to room-temperature reactions.
5.1 Addition of amine compounds
In 1976, Ishida and Mukaiyama conducted an experiment that showed the selectivity of olefin's formation after adding various amine compounds (Table 17).80 Some of the tertiary amine reagents consist of tri-n-butylamine, pyridine, triethylamine, proton sponge (1,8-bis(dimethylamino)naphthalene), and DABCO (1,4-diazabicyclo[2.2.2]octane). The results show that the addition of tertiary amine reagents suppressed the formation of pinacol, 127, and its rearrangement, 128.80 The presence of this additive serves to maintain the reaction atmosphere in an alkaline state to prevent protonation of metallopinacolate intermediates (Fig. 24).
Table 17 McMurry reaction of β-iononea80
Coupling reagent |
Additive |
Temperature (°C) |
Reaction time (h) |
Yield (%) |
126 |
127 |
128 |
A = TiCl4/Zn; B = TiCl4/LiAlH4; PS = proton sponge. |
A |
— |
−10 °C |
1 |
— |
94 |
— |
B |
— |
Reflux |
1 |
44 |
8 |
25 |
B |
n-Bu3N |
Reflux |
3 |
70 |
— |
20 |
B |
n-Bu3N |
Reflux |
3 |
91 |
— |
— |
A |
n-Bu3N |
Reflux |
3 |
63 |
20 |
— |
B |
Pyridine |
Reflux |
3 |
82 |
— |
— |
B |
(C2H5)3N |
Reflux |
3 |
82 |
— |
— |
B |
PS |
Reflux |
3 |
94 |
— |
— |
B |
DABCO |
Reflux |
3 |
72 |
— |
— |
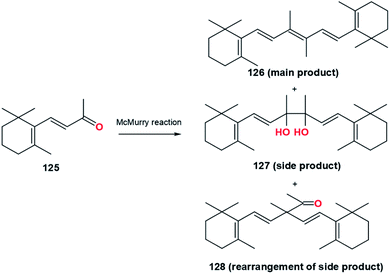 |
| Fig. 24 McMurry reaction of β-ionone.80 | |
Another experiment (Fig. 25) shows the role of pyridine to prevent the formation of the pinacol rearrangement product, namely synthesis of E.Z-bis([3]-ferrocenophane-1-ylidene), 130, from the coupling of [3]-ferrocenophan-1-one, 129.81 It shows that the presence of pyridine can increase the selectivity of the McMurry reaction in producing olefins.
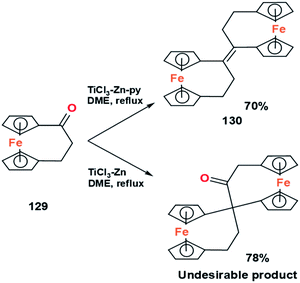 |
| Fig. 25 McMurry coupling of a ferrocenyl ketone with (top) and without (bottom) the presence of pyridin.81 | |
5.2 Addition of alkali metal salts, alkaline earth metal salts, arene, bromide and iodine
Several trials to observe the additive effect of alkali metal salts, alkaline earth metal salts, bromide, iodine, and arene have been reported (Table 18).82–84 Some of these additives increased the activity and E-selectivity of the TiClx–Li system. The experiment used acetophenone, 131, as substrate in TiCl3–Li system coupled to form stilbene, 132, with a minor product in the form of 1,2-diol compounds, 133.82 One of the advantages of using naphthalene and I2 additives is the high formation of olefin product in the low-temperature reaction. Moreover, the McMurry reaction in the TiCl3–Li–naphthalene system has a faster reaction time than the typical McMurry reaction. Additional information from the experiment shows that the main product is dominant in the E configuration compared to the Z configuration. Energetically, the Z configuration is more unstable than the E configuration because the two adjacent larger groups collide, forming an electrical repulsion that destabilizes the molecule (Fig. 26).
Table 18 McMurry reaction of acetophenone with various additives
Additives |
Temperature (°C) |
Reaction time (h) |
Yield (%) |
Ref. |
132 (E : Z) |
133 (dl : meso) |
— |
Reflux |
16 |
89 (75 : 25) |
— |
82 |
— |
0–5 |
16 |
Trace |
87 (75 : 25) |
82 |
I2 |
0–5 |
2 |
90 (80 : 20) |
— |
82 |
Ethyl bromide |
0–5 |
5 |
25 |
67 (80 : 20) |
82 |
1,2-Dibromoethane |
0–5 |
5 |
87 |
— |
82 |
LiI |
25 |
16 |
Trace |
75 (80 : 20) |
83 |
KCl |
25 |
2 |
82 (85 : 15) |
— |
83 |
CsCl |
25 |
2 |
65 (70 : 30) |
— |
83 |
MgCl2 |
25 |
16 |
25 (65 : 35) |
62 (70 : 30) |
83 |
Anthracene |
25 |
3 |
70 (70 : 30) |
Trace |
84 |
Naphthalene |
Reflux |
1.5 |
89 (56 : 44) |
— |
84 |
Naphthalene |
25 |
2 |
77 (68 : 32) |
Trace |
84 |
Naphthalene |
25 |
6 |
68 (30 : 70) |
18 (75 : 25) |
84 |
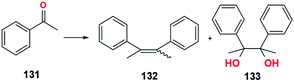 |
| Fig. 26 McMurry reaction of acetophenone.82 | |
6. Effects of Lewis acid, electron withdrawing group, and electron donating group
The first step of the McMurry reaction involves a single electron transfer from the active titanium to the carbonyl group. The carbonyl group needs to be activated to increase its ability to attract electrons from the titanium. Generally, activation of the carbonyl group can be performed by adding Lewis acid. Lewis acid can accept electrons from the carbonyl causing the group to become positively charged. It creates an electron-poor carbonyl with more tendency to attract electrons stronger than an inactivated carbonyl. Apart from carbonyl activation, some experiments have shown that Lewis acid increases radical reactions' selectivity (Table 19, Fig. 27).85,86 In the McMurry reaction, the TiXn compound also acts as Lewis acid. The Lewis acidity of TiXn is altered from mild to strong by replacing X from alkoxide to halide to triflate group.
Table 19 Effect of Lewis acid on the radical reaction of functionalized alkenes with substituted vinylcyclopropanes (Reaction 1) and radical reduction of methyl (±)-2-(1-methoxy-1-phenylmethyl)propenoate (Reaction 2)
Reaction |
Lewis acid |
Temperature (°C) |
Product |
Ref. |
Ratio (E : Z) |
Yield (%) |
1 |
— |
Reflux |
4.4 (136) |
53 |
85 |
1.9 (137) |
1.2 (138) |
1.0 (139) |
10.6 (136) |
1 |
AlMe3 |
−50 |
4.1 (137) |
52 |
85 |
1.4 (138) |
1.0 (139) |
2 |
— |
0 |
1.0 (141) |
49 |
86 |
4.0 (142) |
2 |
MgBr2·OEt2 |
0 |
6.0 (141) |
49 |
86 |
1.0 (142) |
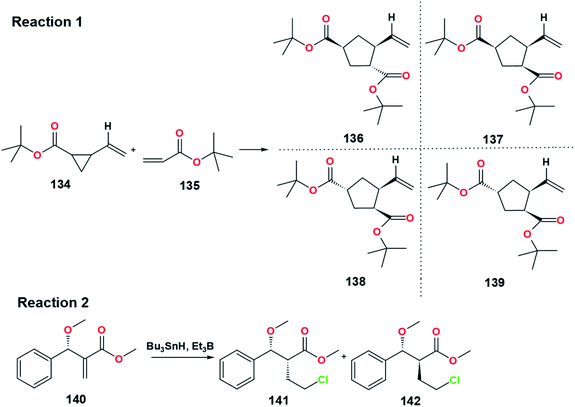 |
| Fig. 27 Effect of Lewis acid on the radical reaction of functionalized alkenes with substituted vinylcyclopropanes (Reaction 1) and radical reduction of methyl (±)-2-(1-methoxy-1-phenylmethyl)propenoate (Reaction 2).85,86 | |
Aside from adding Lewis acid, activation of the carbonyl group can also be carried out by utilizing an electron-attracting functional group or commonly known as the electron-withdrawing group (EWG group). The EWG can draw electrons from adjacent carbonyl towards themselves by resonance or inductive effects, causing an electron deficiency in the carbonyl group. In contrast to EWG, the presence of EDG reduces the reactivity of the carbonyl. An electron-donating group (EDG) donates some of its electrons into a conjugated π system such as carbonyl by resonance or inductive effects, causing the carbonyl tends to be difficult to accept electrons from titanium. By understanding the concepts of EWG and EDG, it can be hypothesized that the presence of EWG adjacent to carbonyl could increase the amount of olefin.
On the other hand, EDG could decrease the amount of olefin. Apart from analyzing the existence of EWG and EDG, determining each group's type of electronic effect is also very important. Although the EDG could deactivate carbonyl, some EDGs can attract electrons from the carbonyl inductively. Thus, the functional group categorized as EDG but able to attract electrons inductively would slightly enhance the olefin yield compared to the inductive electron donor. Several groups classified as EWG and EDG are listed in Table 20.
Table 20 List of electron donating group (EDG) and electron withdrawing group (EWG)a
Substituent |
Classification |
Electronic effect |
Magnitude |
I |
M |
+I (inductively electron donor), −I (inductively withdrawing electron), +M (electron donor via resonance), −M (withdrawing electron via resonance). |
Oxido |
EDG |
+ |
+ |
Very strong |
Hydroxy and ether |
|
− |
+ |
Strong |
Amino |
|
− |
+ |
Strong |
Acyloxy |
|
− |
+ |
Moderate |
Acylamido |
|
− |
+ |
Moderate |
Alkylthio and sulfhydryl |
|
|
+ |
Moderate |
(Di)alkylphosphino |
|
|
+ |
Moderate |
Phenyl |
|
− |
+ |
Weak |
Vinyl |
|
− |
+ |
Weak |
Alkyl |
|
+ |
|
Weak |
Anion carboxylate |
|
+ |
|
Weak |
Fluoro (para position) |
|
− |
+ |
Weak |
Nitro |
EWG |
− |
− |
Strong |
Sulfonic acid and sulfonyl |
|
− |
− |
Strong |
Trifluoromethylsulfonyl |
|
− |
− |
Strong |
Trisubstituted ammonium |
|
− |
|
Strong |
Cyano |
|
− |
− |
Strong |
Trihalomethyl |
|
− |
|
Strong |
Acyl halide, aldehyde, ketone |
|
− |
− |
Moderate |
Carboxylic acid and ester |
|
− |
− |
Moderate |
Amide |
|
− |
− |
Moderate |
Nitroso |
|
− |
± |
Weak |
Halide |
|
− |
+ |
Weak |
Fluoro (ortho and meta position) |
|
− |
+ |
Weak |
In 1981, Castedo et al. tested the effects of EDG substituents on carbonyl coupling reaction using TiCl3/Zn–Cu system (Fig. 28).50 In this section, it is shown that the effects of substituents at the para (R1) and ortho (R3) positions relative to the carbonyl group are more significant compared to meta position. Therefore, R2 can be neglected as long as the R2 group is not bulky (steric effect). The steric effect is shown here when comparing 143a and 143b. By substituting acyloxy (AcO), 143a, at R2 with the bulky CH3OOCCH2CH2OCO (143b), the yield drops from 97% to 60%.
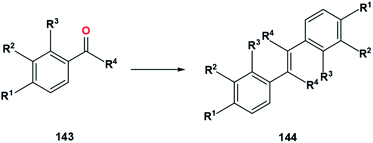 |
| Fig. 28 Carbonyl olefination in TiCl3/Zn–Cu system with various functional groups.50 | |
In term of electronic effect, the direct exchange of the R1 and R2 group between methoxy, 143a, and acyloxy (AcO), 143c, decreases the yield from 97% to 87%. Methoxy is a stronger EDG compared to acyloxy. As previously discussed, the presence of EDG will donate electrons to the aromatic ring. The electrons contained in the aromatic ring will be attracted by the carbonyl, causing the oxygen contained in the carbonyl to be more electron-rich and easier to bond with titanium. This might be the cause of the yield decrease.
However, the steric effect also plays a role in here since when there is no methoxy, 143d, the yield increases to 94%. Another example of the significance of steric effect is on 143e. The presence methyl at R4, even though known as EDG, gives a lower yield at 64% (Table 21).
Table 21 Carbonyl olefination in TiCl3/Zn–Cu system with various functional groups50
Substrate |
Functional group |
Product |
Yield (%) |
R1 |
R2 |
R3 |
R4 |
143a |
CH3O |
AcO |
H |
H |
144a |
97 |
143b |
CH3O |
CH3OOCCH2CH2OCO |
H |
H |
144b |
60 |
143c |
AcO |
CH3O |
H |
H |
144c |
87 |
143d |
AcO |
H |
H |
H |
144d |
94 |
143e |
AcO |
H |
H |
CH3 |
144e |
64 |
McMurry coupling of a substrate with various functional groups to produce indole derivatives by Fürstner and Jumbam (Fig. 29) also showed the expected result.56 Based on the data in Table 22, the replacement of the R group gives a significant difference in results compared to the R′ group. The presence of an amino group adjacent to the carbonyl is predicted to affect the induction from R′, especially amino groups able to attract electrons inductively. Since methyl inductively donating electrons while phenyl inductively withdrawing electrons, exchanging the functional group from phenyl to methyl reduced the amount of indole, 146, synthesized.56 However, even though the reaction is an intramolecular reaction between two adjacent carbonyls, the steric factor also needs to be considered.
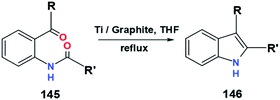 |
| Fig. 29 Synthesis of indole derivatives with various functional groups through McMurry coupling reaction.56 | |
Table 22 Synthesis of indole derivatives with various functional groups through McMurry coupling reaction56
Substrate |
Functional group |
Reaction time (h) |
Product |
Yield (%) |
R |
R′ |
145a |
Me |
Ph |
8 |
146a |
75 |
145b |
Me |
Me |
8 |
146b |
70 |
145c |
Ph |
Ph |
8 |
146c |
90 |
145d |
Ph |
Me |
8 |
146d |
87 |
145e |
Ph |
H |
8 |
146e |
92 |
There are still many factors to increase or decrease the yield of synthesized products, such as steric effects, types of interaction, reaction conditions, reagents, and others. Some of the previously described experiments have slightly different reaction conditions. Therefore, further research is needed to determine the effect of EWG and EDG on carbonyl reactivity without being affected by other factors.
7. Applications of the McMurry reaction
7.1 Synthesis of non-natural products
Numerous synthetic applications of the McMurry coupling reaction in preparing crowded, strained, and polyaromatic alkenes have been reported (Fig. 31). Non-natural products are materials or compounds that can only be obtained through synthesis and cannot be produced by living organisms. In the field of organic electronics, the McMurry reaction is used to prepare a double-helical compound, 148, as a precursor to synthesize naphthotetraindole cores.15 Further treatment of 148 with Cu(I) salt and AgOAc as an oxidant with the addition of PivOH could produce naphthotetraindole with a yield of 67%.15 The use of the conventional McMurry method utilizing the TiCl4–Zn–THF system could produce a moderate yield due to four aromatics stabilizing the substrate, 147. Naphthotetraindole is one of the polycyclic aromatic hydrocarbons (PAHs) as promising organic-based electronics. Generally, compounds belonging to the PAHs group can be obtained from coal and oil deposits. However, naphthotetraindole can only be acquired through organic synthesis pathways that also utilize several inorganic compounds. The presence of a large electron conjugation system in PAHs makes it a candidate for high-performance optoelectronic devices, such as organic-based LEDs, solar cells, and transistors.15 The properties of each compound belonging to PAHs are relatively different due to the various magnitude of the electron conjugation system in PAHs. However, in general terms, PAHs are uncharged and non-polar molecules. One of the other intriguing things about PAHs is the variation of the redox potential that depends on the size of the PAHs. Radical formation of PAHs may be formed when present in McMurry system. Radical PAHs could interact with the same or different radical PAHs, creating larger conjugated systems with new physicochemical and chemical properties.87
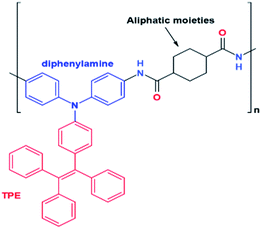 |
| Fig. 30 Design strategy of a modified TPE-based electrofluorochromic material.93 | |
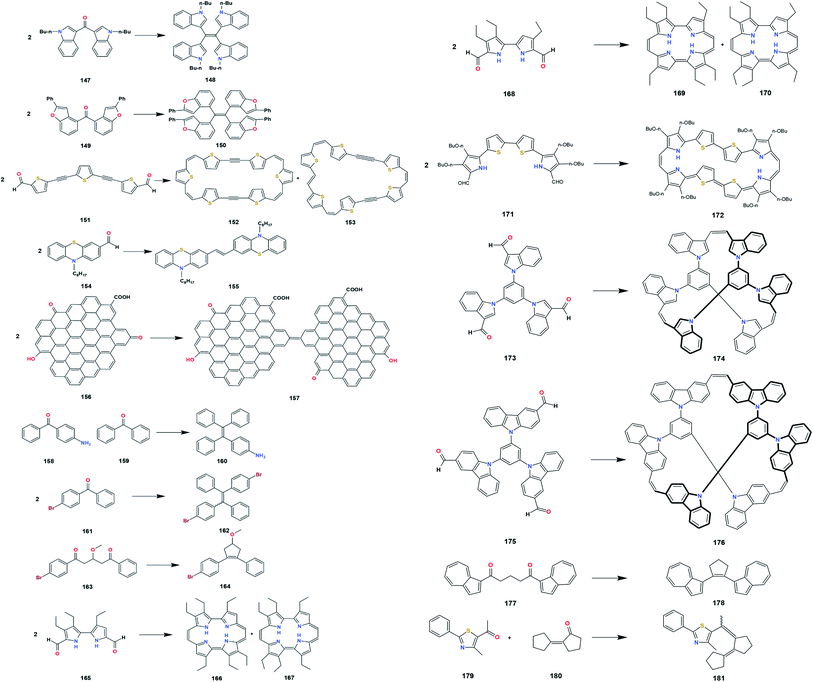 |
| Fig. 31 Application of McMurry reaction in synthesis of non-natural products. | |
In 2018, the group of Nakamura succeeded in synthesizing tetrakis(benzo[b]furyl)ethene (TBFE), 150. This compound is known as an aggregation-responsive dye for their fluorescence-switching property.30 TBFE adopts three switching stages involving intramolecular charge transfer and aggregation-induced emission. According to Hamada, the change in TBFE's wavelength occurred by changing the composition of the solvent. The presence of solvents such as water affected the size of TBFE due to the polarity difference between TBFE and water. In this experiment, the low-valent titanium was replaced by low-valent hafnium due to the involvement of sterically hindered compounds. The use of hafnium is easier to reduce the carbonyl than titanium because of its lower standard reduction potential than titanium (EHf4+/Hf = −1.55 V, ETi4+/Ti = −1.37 V). Another application of the McMurry reaction is related to the reduction of ethynylene-linkage substrates to form a photosensitive product with vinylene-linkage units, 152 and 153.88 Oligothiophenes with π-conjugated macrocycles exhibit optoelectronic properties for multifunctional materials. Based on Shirahata's experiment, the yield of oligothiophene depends on the reaction temperature, the amount of titanium, and the thiophene substituent.88
A new novel synthetic of oligomers is a combination of the McMurry reaction, the Wittig reaction, and the Heck reaction applied to synthesize vinylene-linked molecules, 155, specifically phenothiazinevinylene-based oligomers.89 Phenothiazine has a bent-shape conformation, known as “butterfly” geometry, that can induce molecular aggregation and excimer formation. An excimer is a short-lived complex formed between two identical molecules whose composition is a molecule in the excited and ground states. According to Cibotaru et al., phenothiazine excimers can inhibit the quenching luminescence process.90 This process is crucial in making organic-based LEDs because LEDs are expected to have a constant light intensity. The bent-shape structure of phenothiazine is a result from the two heteroatoms with electron-donor characters on the corner point of the ring. Phenothiazine can develop a supramolecular structure with the involvement of large intermolecular distances. The large intermolecular distance of phenothiazine could facilitate the luminescence of the compound in the solid state. The ability of phenothiazine to delocalize electrons and maintain charge mobility might enhance optical and electronic properties. Phenothiazine was first used as an insecticide and as a drug to treat parasitic worm infections. In addition, phenothiazine can also be used as a sedative, antitumor, anti-allergies, antimalarial, analgesic, antifilarial, trypanocidal, and antipyretics.89,90 Other than that, phenothiazine derivative compounds also have high bioactive activity in dealing with allergies. One of the disadvantages of using phenothiazines is the difficulty of these compounds to dissolve in water, thus requiring polar solvents, which are generally harmful to the environment. In the latest 2020, PEGylated phenothiazine derivatives were successfully synthesized by Cibotaru et al.90 PEGylated phenothiazine is a response to environmental problems caused by phenothiazines. Based on the experiment, the synthetic PEGylated phenothiazine derivative can be dissolved in water or organic solvents such as acetone, DMF, ethanol, methanol, and THF with concentrations below 100 mM.90 In vivo PEGylated phenothiazine testing showed cytotoxicity against cervical cancer cells and biocompatibility on human dermal fibroblasts.90
Enhancing optical and electronic properties can also be achieved by utilizing Graphene Quantum Dots (GQD) material. In 2017, nano GQDs, 157, was successfully crosslinked by Chen et al. via intermolecular McMurry reaction catalyzed by TiCl4 and Zn dust in refluxing THF.91 GQD is a material derived from both carbon dots (CD) and graphene. The formation of GQDs sheets through the McMurry reaction is of particular interest because, with relatively inexpensive reagents, it can form nano-sized products that have the potential to become optoelectronic materials, catalysts, storage, and energy generation.91 One of the advantages of GQDs materials is their use to manufacture and develop photoluminescence-based sensors with the observed colors generally blue and green. In the field of biomaterials, GQDs are used as biosensors to detect the presence of double-stranded DNA (ds-DNA) methylated by DNA methyltransferase I. This is important considering that the presence of abnormal enzyme expression can cause cancer.92 Through this experiment also proved the advantages of the McMurry reaction method for coupling bulk and conjugated substrates.
McMurry coupling reaction is also used to synthesize tetraphenylethylene (TPE) derivatives, 160 and 162, as a candidate for electrochromic (EC) and aggregation—induced emission (AIE)-polyamide materials.93,94 The McMurry reaction produces olefins with a mixture of E and Z isomers which are difficult to separate. To respond to these problems, adding certain functional groups to TPE can cause polarity differences between the E and Z isomers. This difference in polarity causes the mixture of E and Z isomers to be separated easily by polarity-based separation instruments such as HPLC. EC materials exhibit color due to the presence of conjugated polymers that can absorb visible light. Furthermore, the color change in EC occurs reversibly through a redox reaction. Redox reactions can cause changes in the charges of the species that are present in the EC material. In some instances, the difference in charge on certain species causes a significant color change. For example, Fe2+ has a green color while Fe3+ has an orange-brown color. In 2018, sun designed a modified TPE-based electrofluorochromic material with diphenylamine containing aliphatic moieties at the para position against TPE (Fig. 30). The presence of diphenylamine serves as an electroactive site and acts as fluorochromes. Fluorochrome is a chemical compound that can re-emit light upon excitation by light. The aliphatic moieties prevent fluorescence quenching by decreasing the effect of charge transfer. The para position is suggested to ensure the electrochemical stability of the modified TPE.93
A simple and efficient asymmetric synthesis of diaromatic compounds as a precursor for phenanthrenes also utilized the intramolecular McMurry coupling reaction of a substrate containing aldehyde and aromatic, 163, in the TiCl4–Zn–dioxane system.95 Further oxidation of the product, 164, produced cyclopenta[l]phenanthrenes as a starting material to create large aromatic compounds or conjugated polymers.95 Phenanthrene also belongs to the PAH group of compounds used to make dyes, pesticides, medicines, and plastics.15 In porphycene chemistry, two β-hexaalkylated porphycenes, 166 and 169, along with their cis analogues, 167 and 170, were successfully synthesized by Pati et al.96 The reaction utilized TiCl4–Zn–THF mixtures with the addition of copper(I) chloride reagent. Copper halides are used to prevent the oxidation of low-valent titanium by an unpredicted oxidizing agent. The separation of cis- and trans-porphycenes derivative is quite tricky due to the absence of polarity differences. Therefore, separation is performed by repeated recrystallization. Porphycene compounds generally have relatively stable isomers due to the presence of strong intramolecular hydrogen bonds. These interactions contribute to NH tautomerism and impact the double hydrogen transfer process.96 Another experiment related to porphycene is the synthesis of expanded porphycene containing 34 π-electrons, 172.97 The molecule is stable in ambient air and light due to a highly effective cyclic π-conjugation. The large conjugated pi-electron system also plays a role in stabilizing highly reactive organic radical compounds.
Cyclophane is a hydrocarbon compound containing aromatic units and aliphatic chains. The aliphatic chains serve as a bridge that connects aromatic units in a non-adjacent position. Lately, the application of the McMurry reaction in synthesizing new non-natural products from the cyclophane class, 174 (indolophanetriene) and 176 (carbazolophanetriene), has been reported.98 Compounds belonging to the cyclophane class generally can be used as molecular recognition, information storage, and nanodevices.98 By utilizing the McMurry reaction, the intermolecular coupling was performed successfully. Although there are two carbonyls in the same substrate, the intramolecular reaction does not occur because the positions of the two carbonyls are relatively far apart. Reductive McMurry cyclization of α,ω-(diazulen1-yl)-α,ω-diketones, 177, permitted the formation of cycloalkenes, 178, with pinacol and pinacolone as minor products (Table 23).99
Table 23 Application of McMurry reaction in synthesis of non-natural products
Substrate |
Product |
Reagent |
Condition |
Yield (%) |
Ref. |
Source of metal |
Reducing agent |
Solvent |
Additive |
Reaction time (h) |
Temperature (°C) |
147 |
148 |
TiCl4 |
Zn |
THF |
— |
Over-night |
Reflux |
56 |
15 |
149 |
150 |
HfCl4 |
Zn |
CH3CN |
— |
48 |
70 |
50 |
30 |
151 |
152 & 153 |
TiCl4 |
Zn |
THF |
Pyridine |
18 |
66 |
10 (152); 4 (153) |
88 |
154 |
155 |
TiCl4 |
Zn |
THF |
Pyridine |
12 |
Reflux |
63 |
89 |
156 |
157 |
TiCl4 |
Zn |
THF |
— |
24 |
66 (reflux) |
— |
91 |
158 & 159 |
160 |
TiCl4 |
Zn |
THF |
Pyridine |
12 |
Reflux |
61 |
93 |
161 |
162 |
TiCl4 |
Zn |
THF |
Pyridine |
12 |
Reflux |
80 |
94 |
163 |
164 |
TiCl4 |
Zn |
Dioxane |
— |
3 |
Reflux |
63 |
95 |
165 |
166 & 167 |
TiCl4 |
Zn |
THF |
CuCl |
2 |
Reflux |
8 |
96 |
168 |
169 & 170 |
TiCl4 |
Zn |
THF |
CuCl |
2 |
Reflux |
15 |
96 |
171 |
172 |
TiCl4 |
Zn |
THF |
CuCl |
16 |
Reflux |
30 |
97 |
173 |
174 |
TiCl4 |
Zn |
THF |
Pyridine |
12 |
Reflux |
16 |
98 |
175 |
176 |
TiCl4 |
Zn |
THF |
Pyridine |
12 |
Reflux |
14 |
98 |
177 |
178 |
TiCl4 |
Zn |
THF |
Pyridine |
1 |
0 |
88 |
99 |
179 & 180 |
181 |
TiCl4 |
Zn |
THF |
Pyridine |
1.75 |
Reflux |
48 (E : Z = 1 : 3) |
100 |
An experiment conducted by Kochi et al. demonstrated the effectiveness of McMurry coupling to produce 1-arylbutadiene, 181, as a photochromic compound.100 In addition to the previously discussed application of photochromic compounds, one of the important functions of photochromic compounds in the manufacture of glasses is to prevent some objects from being exposed to UV radiation. For example, a product that applies the photochromic concept is photochromic lenses. Photochromic lenses are similar to eyeglass lenses in general when in a room with relatively low light intensity. However, these lenses may darken when exposed to sunlight. Color changes occur due to UV radiation from the sun, which has an impact on photochromic molecules. By using photochromic lenses, the eyes will be protected from direct exposure to UV light. Excessive exposure to UV B rays into the eye can cause cataracts up to permanent blindness.
7.2 Synthesis of natural products
Various applications of McMurry coupling in the synthesis of natural and related products have appeared that efficiently construct a wide variety of macromolecules (Fig. 32). A natural product is a substance or compound produced by bio-based materials, biotic materials, biological fluids, and other materials from living organisms.
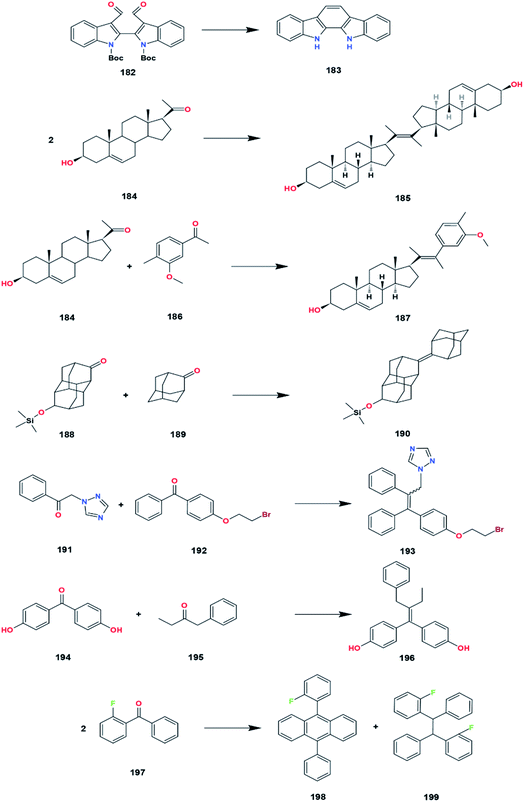 |
| Fig. 32 Application of McMurry reaction in synthesis of natural products. | |
The intramolecular cyclization promoted by titanium halides with reducing agents has been applied to the total synthesis of indolo[2,3-α]carbazole, 183, that provides pharmacological effects such as antifungal, antihypertensive, antimicrobial, and anticancer.101 Protection of the amine with boc compound increased the yield of the indolo[2,3-α]carbazole. Both amines and amides can react slowly with low valent titanium. Therefore, protection of these groups is needed to prevent the complexation of titanium to nitrogen atoms. Apart from being synthesized, indolo(2,3-a)carbazoles can be isolated directly from marine invertebrates (sponge, tunicate, mollusk), myxomycetes (Arcyria ferruginea and Arcyria cinerea), actinomycetes, and blue-green algae (Nostoc sphaericum and Fischerella ambigua).
In 2019, Abdul-Reda and Muhee succeeded in synthesizing pregnenolone derivatives, 185 and 187, via an intermolecular coupling reaction.102 Pregnenolone is hydrophobic with the basic structure of four hydrocarbon rings connected. Some of the functional groups present in pregnenolone consist of a ketone bonded to C17, two methyls bonded to C13 and C18, a hydroxyl bonded to C3, and a carbon–carbon double bond at C5. It is a main steroid synthesized from cholesterol in invertebrates and mammals that plays an essential role in producing other steroid hormones, including progesterone, estrogen, and dehydroepiandrosterone (DHEA). Pregnenolone is naturally produced in the adrenal glands, brain, and gonads.103 Some of its derivatives are candidates for treating prostate cancer, bacterial infection, and fungal infection. Moreover, pregnenolone and its derivatives could enhance the neuroactive properties for learning and keeping memory.
In 2018, A novel synthetic of diamondoids compound, especially adamantane, 190, has also been reached by Hoc et al. using the McMurry reaction.104 Diamondoid is an unusual molecule that has been widely applied in geochemistry since 1990 due to its peculiar cage molecular structure. Both diamondoid and its derivatives are contained naturally in oil and fossil deposits with a 1–100 ppm concentration.104 The low series of diamondoids plays a vital role in designing and synthesizing drug delivery systems. The experimental results showed that the McMurry coupling of diamantanone and amantanone was successful with high product yields.
McMurry reaction is also used as the fundamental synthetic step in preparing the precursor, 193 and 196, to synthesize tamoxifen derivative compound as a candidate for treating breast cancer, dysmenorrhea, and gynecomastia.105,106 Tamoxifen belongs to the triphenylethylene family that acts as a selective estrogen receptor modulator (SERM). The structure of tamoxifen was derived from estrogen (diethylstilbestrol) and antiestrogen such as chlorotrianisene. This causes tamoxifen to have estrogen and antiestrogen activity that can work in certain parts of the body. For example, tamoxifen has a predominant antiestrogen activity in the breast area and predominant estrogen activity in the uterus.
Anthracene or green oil is a compound that can be obtained from coal tar. In the previous section, the presence of anthracene also increased the selectivity of the McMurry reaction. In 2019, the phenylanthracene core compound 198 was successfully synthesized by Akhmetov et al. using fluorinated benzophenone.107 The success of these experiments opens up opportunities for fluoroorganic chemistry to be researched and studied further (Table 24).
Table 24 Application of McMurry reaction in synthesis of natural products
Substrate |
Product |
Reagent |
Condition |
Yield (%) |
Ref. |
Source of metal |
Reducing agent |
Solvent |
Additive |
Reaction time (h) |
Temperature (°C) |
182 |
183 |
TiCl4 |
Zn–Cu |
THF |
— |
20 |
Reflux |
65 |
101 |
184 |
185 |
TiCl4 |
Zn |
THF |
— |
16 up to 45 |
Reflux |
74 |
102 |
184 & 186 |
187 |
TiCl4 |
Zn |
THF |
— |
16 up to 45 |
Reflux |
73 |
102 |
188 & 189 |
190 |
TiCl4 |
Zn |
THF |
Pyridine |
20 up to 24 |
Reflux |
92 |
104 |
191 & 192 |
193 |
TiCl4 |
Zn |
THF |
— |
6 |
−10 up to reflux |
44 (E : Z = 3 : 1) |
105 |
194 & 195 |
196 |
TiCl4 |
Zn |
THF |
— |
2.5 |
Reflux |
87 |
106 |
197 |
198 & 199 |
TiCl4 |
Zn |
THF |
Pyridine |
Over-night |
Reflux |
30 (198); 45 (199) |
107 |
8. Conclusions
McMurry's reaction provides an efficient way to create a new carbon–carbon bond from carbonyl compounds. Over the past 5 years, the reductive coupling of carbonyls has been widely applied to the synthesis of sophisticated and complicated molecules with fascinating optical, optoelectronic, photochromic, luminescent properties, and even diverse biological activities. Several medium and large ring systems such as PAHs, indole derivatives, carbazole derivatives, cross-linked GQD, diamondoid derivatives, and other complicated molecules have been successfully obtained through intermolecular, intramolecular, tandem coupling, and keto ester McMurry coupling reaction.
Based on the current hypothesis, the McMurry reaction adapts a heterogeneously catalysed reaction which assumes that the low-valent titanium species is a surface that interacts with oxygen on the carbonyl substrate. Further investigations are needed to confirm the number of titanium species involved on one surface during the interaction process and the manner of Ti–O bond-breaking to get a better understanding to the McMurry reaction mechanism.
Despite various applications of the McMurry reaction in recent decades, there are still some drawbacks of this reaction that should be considered. The major disadvantage of the McMurry reaction is its intolerance of easily reduced side functional groups such as allylic alcohol, 1,2-diol, nitro, sulfoxide, epoxide, halohydrin, enedione, and quinone. This problem has now been controlled by applying a low-temperature reaction method that utilizes certain additive compounds. It is essential to look for further alternatives considering there is little chance of redox reactions and diol formation occurring at low temperatures. Another way to address this problem, Asako has reviewed some recent progress in transition-metal-catalyzed deoxygenative coupling reactions under mild conditions.22 Some of the examples are [Ir(FCF3ppy)2dtbpy]PF6/B2pin2 system and [Rh2(OAc)4]. By utilizing these catalysts, the reactions can be carried out in milder condition and increase the tolerance to easily reduced side functional groups. In addition to this problem, the development of the enantioselectivity of this reaction is necessary by considering that complicated systems are still required to obtain products with higher selectivity, causing an inefficient reaction with relatively low yields.
In summary, McMurry reaction has a lot of potentials to be applied and developed in the future. Further explorations with a different approach might be needed to verify the specific reaction mechanism, the interaction between the substrate and the reagent on a molecular basis, optimum reaction condition, and the suitability of additive with reaction. Understanding these factors may lead to discovering a modified McMurry reaction to synthesize various complicated compounds with satisfactory results and overcome the shortcomings of conventional McMurry reactions.
Author contributions
All authors contributed to the preparation of the draft. A. Bongso participated for the preparation of the original draft and graphic visualisations. R. Roswanda and Y. M. Syah contributed substantial revision for the final draft.
Conflicts of interest
The authors declare that there are no conflicts of interest.
References
- B. Huang, Y. Shen, Z. Mao, Y. Liu and S. Cui, Org. Lett., 2016, 18, 4888–4891 CrossRef CAS PubMed.
- K. Esfandiarfard, J. Mai, S. Ott, K. Esfandiarfard, J. Mai and S. Ott, J. Am. Chem. Soc., 2017, 139, 2940–2943 CrossRef CAS PubMed.
- S. Tyrlik and I. Wolochowicz, Bull. Soc. Chim. Fr., 1973, 2147 CAS.
- T. Mukaiyama, T. Sato and J. Hanna, Chem. Lett., 1973, 2, 1041–1044 CrossRef.
- J. E. McMurry and M. P. Fleming, J. Am. Chem. Soc., 1974, 96, 4708–4709 CrossRef CAS PubMed.
- J. E. McMurry and M. P. Fleming, J. Org. Chem., 1976, 41, 1–2 CrossRef.
- J. E. McMurry, M. P. Fleming, K. L. Kees and L. R. Krepski, J. Org. Chem., 1978, 43, 3255–3266 CrossRef CAS.
- J. E. McMurry, T. Lectka and J. G. Rico, J. Org. Chem., 1989, 54, 3748–3749 CrossRef CAS.
- D. Lenoir, Synthesis, 1977, 553–554 CrossRef CAS.
- A. Fürstner, R. Csuk, C. Rohrer and H. Weidmann, J. Chem. Soc., Perkin Trans. 1, 1988, 1729–1734 RSC.
- D. L. J. Clive, K. S. K. Murthy, A. G. H. Wee, J. S. Prasad, G. V. J. da Silva, M. Majewski, P. C. Anderson, R. D. Haugen and L. D. Heerze, J. Am. Chem. Soc., 1988, 110, 6914–6916 CrossRef CAS.
- B. E. Kahn and R. D. Rieke, Chem. Rev., 1988, 88, 733–745 CrossRef CAS.
- J. E. McMurry, Chem. Rev., 1989, 89, 1513–1524 CrossRef CAS.
- X.-F. Duan, J. Zeng, J.-W. Lü and Z.-B. Zhang, J. Org. Chem., 2006, 71, 9873–9876 CrossRef CAS PubMed.
- X. Zheng, R. Su, Z. Wang, T. Wang, Z. Bin, Z. She and G. Gao, Org. Lett., 2019, 21, 797–801 CrossRef CAS PubMed.
- J. E. McMurry and J. G. Rico, Tetrahedron Lett., 1989, 30, 1169–1172 CrossRef CAS.
- A. Fürstner and A. Hupperts, J. Am. Chem. Soc., 1995, 117, 4468–4475 CrossRef.
- H. R. Diéguez, A. López, V. Domingo, J. F. Arteaga, J. A. Dobado, M. M. Herrador, J. F. Quílez del Moral and A. F. Barrero, J. Am. Chem. Soc., 2010, 132, 254–259 CrossRef PubMed.
- L. Zhang, X. Yu, L. Zhang, X. Zhou and Y. Lin, Org. Chem. Front., 2014, 1, 929–935 RSC.
- S. Wang, N. Lokesh, J. Hioe, R. M. Gschwind and B. König, Chem. Sci., 2019, 10, 4580 RSC.
- Y. Xia, Z. Liu, Q. Xiao, P. Qu, R. Ge, Y. Zhang and J. Wang, Angew. Chem., Int. Ed., 2012, 51, 5714 CrossRef CAS PubMed.
- S. Asako and L. Ilies, Chem. Lett., 2020, 49, 1386–1393 CrossRef CAS.
- T. Takeda and A. Tsubouchi, Org. React., 2013, 82, 1–470 CAS.
- M. Ephritikhine and C. Villiers, in Modern Carbonyl Olefination, ed. T. Takeda, Wiley-VCH, Hoboken, NJ, 1st edn, 2004, pp. 223–285 Search PubMed.
- J. J. Eisch and P. O. Fregene, Eur. J. Org. Chem., 2008, 4482–4492 CrossRef CAS.
- J. Howarth and J. Finnegan, Synth. Commun., 1997, 27, 3663–3668 CrossRef CAS.
- Y. Fujiwara, R. Ishikawa, F. Akiyama and S. Teranishi, J. Org. Chem., 1978, 43, 2477–2480 CrossRef CAS.
- T. Kauffmann and H. Kallweit, Chem. Ber., 1992, 125, 149–151 CrossRef CAS.
- R. Dams, M. Malinowski and H. J. Geise, Bull. Soc. Chim. Belg., 1982, 91, 149–152 CrossRef CAS.
- H. Hamada, H. Tsuji and E. Nakamura, Mater. Chem. Front., 2018, 2, 296–299 RSC.
- M. Moxter, J. Tillmann, M. Füser, M. Bolte, H. W. Lerner and M. Wagner, Chem.–Eur. J., 2016, 22, 16028–16031 CrossRef CAS PubMed.
- V. K. Harit and N. G. Ramesh, J. Org. Chem., 2016, 81, 11574–11586 CrossRef CAS PubMed.
- J. E. McMurry and L. R. Krepski, J. Org. Chem., 1976, 41, 3929–3930 CrossRef CAS.
- J. A. Bravo and J. L. Vila, Rev. Boliv. Quim., 2018, 35, 73–84 CAS.
- M. Sander and E. V. Dehmlow, Eur. J. Org. Chem., 2001, 5, 399–404 CrossRef.
- D. D. Yu and B. M. Forman, J. Org. Chem., 2003, 68, 9489–9491 CrossRef CAS PubMed.
- S. Top, A. Vessières, G. Leclercq, J. Quivy, J. Tang, J. Vaissermann, M. Huché and G. Jaouen, Chem.–Eur. J., 2003, 9, 5223–5236 CrossRef CAS PubMed.
- P. Pigeon, M. Görmen, K. Kowalski, H. Müller-Bunz, M. J. Mcglinchey, S. Top and G. Jaouen, Molecules, 2014, 19, 10350–10369 CrossRef PubMed.
- R. Daik, W. J. Feast, A. S. Batsanov and J. A. K. Howard, New J. Chem., 1998, 22, 1047–1049 RSC.
- I. A. Khotina, V. A. Izumrudov, N. V. Tchebotareva and A. L. Rusanov, Macromol. Chem. Phys., 2001, 202, 2360–2366 CrossRef CAS.
- S. Gupta, G. K. Kar and J. K. Ray, Synth. Commun., 2000, 30, 2393–2399 CrossRef CAS.
- V. Rauniyar, H. Zhai and D. G. Hall, J. Am. Chem. Soc., 2008, 130, 8481–8490 CrossRef CAS PubMed.
- W. R. Roth and H. H. C. Horn, Chem. Ber., 1994, 1781–1795 CrossRef CAS.
- B. W. Disanavaka and A. C. Weedon, J. Org. Chem., 1987, 52, 2905–2910 CrossRef.
- L. A. Paquette, G. J. Wells and G. Wickham, J. Org. Chem., 1984, 49, 3618–3621 CrossRef CAS.
- A. Fürstner, G. Seidel, B. Gabor, C. Kopiske, C. Krüger and R. Mynott, Tetrahedron, 1995, 51, 8875–8888 CrossRef.
- J. Nakayama, H. Machida, R. Saito and M. Hoshino, Tetrahedron Lett., 1985, 26, 1983–1984 CrossRef CAS.
- P. Blanchard, H. Brisset, R. Hierle and J. Roncali, J. Org. Chem., 1998, 63, 8310–8319 CrossRef CAS.
- P. Blanchard, H. Brisset, B. Illien, A. Riou and J. Roncali, J. Org. Chem., 1997, 62, 2401–2408 CrossRef CAS PubMed.
- L. Castedo, J. M. Saá, R. Suau and G. Tojo, J. Org. Chem., 1981, 46, 4292–4294 CrossRef CAS.
- K. Agbaria and S. E. Biali, J. Org. Chem., 2001, 66, 5482–5489 CrossRef CAS PubMed.
- P. Toullec, L. Ricard and F. Mathey, Organometallics, 2002, 21, 2635–2638 CrossRef CAS.
- T.-A. Niemi, P. L. Coe and S. J. Till, J. Chem. Soc., Perkin Trans. 1, 2000, 1519–1528 RSC.
- J. A. Seijas, A. R. De Lera, M. C. Villaverde and L. Castedo, J. Chem. Soc., Chem. Commun., 1985, 839–840 RSC.
- B. M. Fox, X. Xiao, S. Antony, G. Kohlhagen, Y. Pommier, B. L. Staker, L. Stewart and M. Cushman, Bioorg. Med. Chem., 2004, 12, 5147–5160 CrossRef PubMed.
- A. Fürstner and D. N. Jumbam, Tetrahedron, 1992, 48, 5991–6010 CrossRef.
- A. Fürstner, H. Weintritt and A. Hupperts, J. Org. Chem., 1995, 60, 6637–6641 CrossRef.
- E. J. A. Fürstner, A. Hupperts and A. Ptock, J. Org. Chem., 1994, 59, 5215–5229 CrossRef.
- A. Fürstner and D. N. Jumbam, J. Chem. Soc., Chem. Commun., 1993, 211–212 RSC.
- A. Fürstner, D. N. Jumbam and G. Seidel, Chem. Ber., 1994, 127, 1125–1130 CrossRef.
- A. Fürstner and A. Ernst, Tetrahedron, 1995, 51, 773–786 CrossRef.
- A. Fürstner, A. Ernst, H. Krause and A. Ptock, Tetrahedron, 1996, 52, 7329–7344 CrossRef.
- R. Dams, M. Malinowski and H. J. Geise, Recl. Trav. Chim. Pays-Bas, 1982, 101, 112–114 CrossRef CAS.
- U. Berlage, J. Schmidt, U. Peters and P. Welzel, Tetrahedron Lett., 1987, 28, 3091–3094 CrossRef CAS.
- A. Fürstner, O. R. Thiel, N. Kindler and B. Bartkowska, J. Org. Chem., 2000, 65, 7990–7995 CrossRef PubMed.
- S. Sabelle, J. Hydrio, E. Leclerc, C. Mioskowski and P. Y. Renard, Tetrahedron Lett., 2002, 43, 3645–3648 CrossRef CAS.
- J. Mai, A. I. Arkhypchuk, A. K. Gupta and S. Ott, Chem. Commun., 2018, 54, 7163–7166 RSC.
- X. Ariza, A. M. Costa, M. Faja, O. Pineda and J. Vilarrasa, Org. Lett., 2000, 2, 2809–2811 CrossRef CAS PubMed.
- J. E. M. Murry and M. Silvestri, J. Org. Chem., 1975, 40, 2687–2688 CrossRef.
- G. Dou, M. Wang and D. Shi, J. Comb. Chem., 2009, 11, 151–154 CrossRef CAS PubMed.
- W. Zhong, X. Chen and Y. Zhang, Synth. Commun., 2000, 30, 4451–4460 CrossRef CAS.
- A. Sera, S. Fukumoto, M. Tamura, K. Takabatake, H. Yamada and K. Itoh, Bull. Chem. Soc. Jpn., 1991, 64, 1787–1791 CrossRef CAS.
- T.-L. Ho and C. M. Wong, Synth. Commun., 1973, 3, 37–38 CrossRef CAS.
- B. W. Yoo, K. H. Choi, S. J. Lee, C. M. Yoon, S. H. Kim and J. H. Kim, Synth. Commun., 2002, 32, 63–67 CrossRef CAS.
- B. W. Yoo, K. H. Choi, D. Y. Kim, K. Il Choi and J. H. Kim, Synth. Commun., 2003, 33, 53–57 CrossRef CAS.
- M. Shimizu, K. Shibuya and R. Hayakawa, Synlett, 2000, 1437–1438 CAS.
- J. E. McMurry and M. P. Fleming, J. Org. Chem., 1975, 40, 2555–2556 CrossRef CAS.
- T. Jiménez, A. G. Campaña, B. Bazdi, M. Paradas, D. Arráez-Román, A. Segura-Carretero, A. Fernández-Gutiérrez, J. E. Oltra, R. Robles, J. Justicia and J. M. Cuerva, Chem.–Eur. J., 2010, 4288–4295 Search PubMed.
- F. Bermejo and C. Sandoval, J. Org. Chem., 2004, 69, 5275–5280 CrossRef CAS PubMed.
- A. Ishida and T. Mukaiyama, Chem. Lett., 1976, 5, 1127–1130 CrossRef.
- P. Härter, K. Latzel, M. Spiegler and E. Herdtweck, Polyhedron, 1998, 17, 1141–1148 CrossRef.
- S. Talukdar, S. K. Nayak and A. Banerji, J. Org. Chem., 1998, 63, 4925–4929 CrossRef CAS.
- S. Rele, S. Chattopadhyay and S. K. Nayak, Tetrahedron Lett., 2001, 42, 9093–9095 CrossRef CAS.
- S. Rele, S. Talukdar, A. Banerji and S. Chattopadhyay, J. Org. Chem., 2001, 66, 2990–2994 CrossRef CAS PubMed.
- K. S. Feldman, A. L. Romanelli, R. E. Ruckle and R. F. Miller, J. Am. Chem. Soc., 1988, 110, 3300–3302 CrossRef CAS.
- Y. Guindon and J. Rancourt, J. Org. Chem., 1998, 63, 6554–6565 CrossRef CAS.
- R. S. Ruoff, K. M. Kadish, P. Boulas and E. C. M. Chen, J. Phys. Chem., 1995, 99, 8843–8850 CrossRef CAS.
- K. Shirahata, M. Takashika, K. Hirabayashi, M. Hasegawa, H. Otani, K. Yamamoto, Y. Ie, T. Shimizu, S. Aoyagi and M. Iyoda, J. Org. Chem., 2021, 86, 302–309 CrossRef CAS PubMed.
- X. Qiu and R. Lu, ChemistrySelect, 2020, 5, 12218–12223 CrossRef CAS.
- S. Cibotaru, A. I. Sandu, D. Belei and L. Marin, Mater. Sci. Eng., C, 2020, 116, 111216 CrossRef CAS PubMed.
- L. Chen, P. Hu, J. E. Lu and S. Chen, Chem.–Asian J., 2017, 12, 973–977 CrossRef CAS PubMed.
- D. Shahdeo, A. Roberts, N. Abbineni and S. Gandhi, Compr. Anal. Chem., 2020, 91, 175–199 CAS.
- N. Sun, K. Su, Z. Zhou, Y. Yu, X. Tian, D. Wang, X. Zhao, H. Zhou and C. Chen, ACS Appl. Mater. Interfaces, 2018, 10, 16105–16112 CrossRef CAS PubMed.
- N. Sun, K. Su, Z. Zhou, D. Wang, A. Fery, F. Lissel, X. Zhao and C. Chen, Macromolecules, 2020, 53, 10117–10127 CrossRef CAS.
- D. M. Connors and N. S. Goroff, Org. Lett., 2016, 18, 4262–4265 CrossRef CAS PubMed.
- N. N. Pati, B. S. Kumar and P. K. Panda, Org. Lett., 2017, 19, 134–137 CrossRef CAS PubMed.
- A. Rana, Y. Hong, T. Y. Gopalakrishna, H. Phan, T. S. Herng, P. Yadav, J. Ding, D. Kim and J. Wu, Angew. Chem., Int. Ed., 2018, 130, 12714–12717 CrossRef.
- N. Venkatesan and P. Rajakumar, ChemistrySelect, 2019, 4, 1103–1107 CrossRef CAS.
- E. A. Dragu and A. C. Razus, Rev. Roum. Chim., 2020, 83–88 CrossRef.
- J. I. Kochi, T. Ubukata and Y. Yokoyama, J. Org. Chem., 2018, 83, 10695–10700 CrossRef CAS PubMed.
- V. Lösle and H. Knölker, ARKIVOC, 2020, 7, 192–200 Search PubMed.
- N. A. Abdul-Reda and A. A. Muhee, J. Phys.: Conf. Ser., 2019, 1294, 1–17 Search PubMed.
- M. Vallée, J. Steroid Biochem. Mol. Biol., 2016, 160, 78–87 CrossRef PubMed.
- N. T. Hoc, V. N. Rodionov and A. A. Fokin, Org. Commun., 2018, 11, 75–79 CrossRef CAS.
- M. S. R. Murty, M. R. Katiki, J. B. Nanubolu, S. Garimella, S. Polepalli, N. Jain, S. K. Buddana and R. S. Prakasham, Mol. Diversity, 2016, 20, 687–703 CrossRef CAS PubMed.
- N. H. Elghazawy, M. Engel, R. W. Hartmann, M. M. Hamed, N. S. Ahmed and A. H. Abadi, Future Med. Chem., 2016, 8, 249–256 CrossRef CAS PubMed.
- V. Akhmetov, M. Feofanov, V. Ioutsi, F. Hampel and K. Amsharov, Chem.–Eur. J., 2019, 25, 1910–1913 CrossRef CAS PubMed.
|
This journal is © The Royal Society of Chemistry 2022 |