DOI:
10.1039/D2RA00392A
(Paper)
RSC Adv., 2022,
12, 7485-7496
Improvement strategies for quality defects and oxidation of pale, soft and exudative (PSE)-like chicken meat: effects of domestic cooking and core temperature
Received
19th January 2022
, Accepted 28th February 2022
First published on 7th March 2022
Abstract
This study compared the quality, oxidation, and microstructure of high-market-share PSE-like chicken meat (PSE) after domestic cooking with those of normal chicken meat (NOR). Cooking techniques included steaming (ST), boiling (BO), roasting (RO), and microwaving (MV) at 60, 70, and 80 °C. The results indicated that PSE-induced chicken breasts were of poor quality, with significantly higher cooking loss rates (NOR: 22.1% vs. PSE: 26.2%) and shear force (NOR: 50.4 N vs. PSE: 69.2 N) than normal chicken meat. In addition, PSE-like chicken meat showed higher oxidative sensitivity and more severe muscle fiber structure damage. Among the four cooking techniques, RO increased meat toughness (NOR: 78.5 N vs. PSE: 98.3 N) and intensified excessive protein oxidation and aggregation in PSE chicken breast most significantly, manifested by the increased malondialdehyde (NOR: 0.46 vs. PSE: 0.57, mg kg−1 meat) and carbonyl (NOR: 11.2 vs. PSE: 13.4, nmol mg−1 protein), reduced tryptophan and thiols (NOR: 41.3 vs. PSE: 33.7, nmol mg−1 protein), and prominent protein cross-linking such as Schiff bases and disulfide bonds during heat treatment (p < 0.05). BO was the second most destructive technique, while MV caused the least impact (p > 0.05). Principal component analysis indicated a correlation between oxidative damage and meat quality, which was attributed to variations of the PSE and normal samples by BO, RO, and ST treatments. Thus, MV is suggested to be a promising and effective cooking method in reducing the differences in quality and oxidation attributes between PSE and normal chicken meat.
1. Introduction
Global production and consumption of poultry meat, especially chicken, have grown rapidly over the past few decades. Chicken, which constitutes more than 90% of the demands in the poultry market, is considered an excellent source of protein and a healthier dietary alternative than red meat.1 The quality of chicken meat, manifested by color and water holding capacity, is particularly important to consumers and industrial companies. Due to the breeding and feeding of genetic varieties, rapid muscle growth of chickens may result in meat quality defects. In particular, PSE-like chickens receive much attention for their pale appearance and exudative loss.2 Currently, many efforts of pre-mortem management and standardized post-mortem treatments have been performed to reduce PSE-like poultry meat.3 It has been reported that PSE-like meat accounts for more than 40% of the unprocessed poultry products sold in the market and is directly available to consumers for domestic cooking.4,5 However, the differences between PSE-like chicken and normal meat after being purchased for domestic cooking deserve to be investigated.
Tiwari and O'Donnell6 classified meat cooking methods into several categories, including dry (roasting), moist (boiling and steaming), and novel heating (microwaving), all of which were frequently used in domestic cooking. Each cooking method has its own characteristics, including heat transfer method and medium as well as cooking time and temperature, which are essential for developing the sensory and textural qualities of meat.7,8 It has been reported that steaming, boiling, and microwaving can lead to protein denaturation, muscle fiber contraction, and collagen dissolution, resulting in tender meat.9,10 Roasting is thought to denature myofibrillar proteins and increase the contraction and dehydration of collagen, leading to tougher meat.7 Furthermore, the different processing methods lead to changes in the structure of the meat, thus affecting the quality and palatability of the final product.8 Studies have shown that boiling, with an internal temperature ranging from 40 to 95 °C, contributes to increasingly dense and compact fiber arrangements of duck and chicken meat.11 In contrast, longitudinal and lateral shrinkage of chicken muscle fibers after microwave cooking is significantly lower than that after roasting and steaming, and the destruction and loss of protein matrix are less.6,12
Cooking can also induce a cascade of chemical and physical changes, including the oxidation of meat, which may potentially impact human health. Oxidation of protein in the diet may represent a human disease risk factor through increase in oxidative stress.13 Meat heating has been proved to induce protein oxidation, including the gain of carbonyl derivatives, reduction of thiol groups, loss of tryptophan fluorescence, accumulation of Schiff base (SB) structures, and formation of intra- and intermolecular cross-linking.14 Moreover, the extent of protein oxidation in cooked meat is also associated with organoleptic characteristics of meat, including water loss, texture changes, and flavor formation.15 It has been documented that PSE-like chicken breasts are more susceptible to protein hydrolysis and endogenous antioxidant enzyme activity during storage than normal meat.16,17 However, there is no report on the extent of oxidation in PSE-like chicken after domestic cooking. Thus, it is crucial to clarify the occurrence, intensity, and consequences of PSE-induced chicken meat protein oxidation during cooking.
This study aims to investigate the quality, microstructure, and meat oxidation of PSE-like chicken meat by different domestic cooking methods at core meat temperatures of 60, 70, and 80 °C, and compared with normal chicken meat. The primary outcome of this paper can give the basis for gaining important practical guidelines when consuming PSE-like chicken in domestic cooking collections.
2. Materials and methods
2.1 Meat samples and cooking methods
All animal procedures were performed in accordance with the Guidelines for Care and Use of Laboratory Animals of Yangzhou University and approved by the Institutional Animal Care and Use Committee (IACUC) of the Yangzhou University Animal Experiments Ethics Committee (permit number: SYXK (Su) IACUC 2016-0020). A total of 200 eight-week-old broiler chickens were acquired from a Chinese commercial meat processing plant (Jiulian Inc., Qingdao, Shandong, China). After slaughter, the chicken breasts (pectoralis major muscle) were removed from the carcass and then transported to the laboratory at 4 °C. Lightness (L*) and pH values were measured at 24 h post-mortem to classify PSE-like and normal meat. According to previous reports,2–5 the samples with 5.7 < pH24 h < 6.1, 46 <
< 53 were categorized as normal meat and the samples with pH24 h < 5.7,
> 53 were categorized as PSE-like meat.
Connective tissues in chicken breasts were trimmed, and these breasts were sliced (6 cm length × 5 cm width × 2 cm height) in the myofibril direction. Each slice weighed approximately 95 g ± 5 g. The 45 PSE-like and normal chicken breast pieces were equally divided into five groups. The first group was uncooked and assigned as the control group (RW). The other four groups were cooked by steaming (ST), boiling (BO), roasting (RO), and microwaving (MV), respectively, according to the methods of Hu et al.15 and Mitra et al.14 No additional ingredients were added to avoid interference. With the steaming treatment, the chicken breasts were evenly placed on a stainless steel tray and then sealed in a steamer (Midea, Zhejiang, China), and the gas cooker (TH28b, Fotile, China) was set at 100 °C. Boiling treatment was performed by firstly placing the chicken breasts into food-grade vacuum-packaged bags (PA/PE-70, Jiangsu Taili Packaging Products Co., Ltd, Jiangsu, China) and then immersing them in the water in a stainless-steel pot. The temperature of the induction cooker (EC30, Supor, China) was set to 100 °C. For roasting treatment, the chicken breasts were roasted on grid-shaped bakeware in a forced-air convection oven (SCC61WE, RATIONAL, Germany) preheated to 250 °C. Microwaving treatment was performed using a microwave-applicable tray in a microwave oven (W25800-01AG, Fotile, China), with the power of 800 W (the cooking program is shown in Fig. 1).
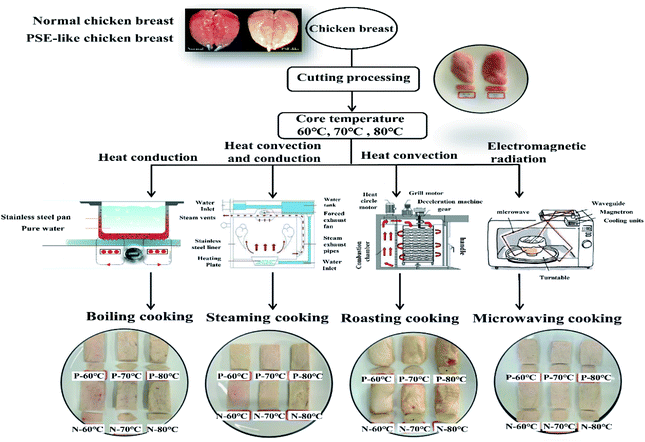 |
| Fig. 1 Cooking schematic of PSE-like and normal chicken breast under different cooking methods. Abbreviation: (RW) indicated raw meat, (N) indicated normal chicken breast, and (P) indicated PSE-like chicken breast. | |
The core temperature of the meat during cooking was monitored in real-time by inserting a fiber optic sensor (SSN-22, WenYu, China) into the geometric center of the chicken breast muscles. The final temperature was recorded by a Testo 176 T4 data logger fitted with a T-Type external probe and was further confirmed by a hand-held thermometer (A61, UNI-TREND, China). Once the core temperature reached 60, 70, and 80 °C, the samples were immediately removed from each container and cooled in an ice-cold water bath (4 °C). After the surface liquid was drained, the cooked chicken breasts were weighed to calculate the cooking loss, which was expressed as the percentage of the weight difference between the pre- and post-cooling over the pre-cooking weight. After the quality parameters of chicken breasts were obtained, the cooked meat was homogenized using a grinder (C-010, Joyoung, China). The minced meat was then stored at −80 °C until biochemical analysis including the carbonyl, total thiols (SH), disulfide bonds (S–S), thiobarbituric acid reactive substances (TBARS), tryptophan, Schiff base (SB) and sodium dodecyl sulfate-polyacrylamide gel electrophoresis (SDS-PAGE).
2.2 Evaluation of pH, color, and Warner–Bratzler shear force (WBSF)
The pH of the pre-and post-cooking chicken breasts was measured by the plug in pH meter (Testo 205, China). Firstly, the pH meter was calibrated by using the pH standard buffers of 4.0, 7.0, and 10.0 (Sangon Biotech Co., Ltd, China) to complete a three-point calibration. Then the probe was inserted into the center of the meat. The pH values were recorded for each sample at five different sites. The color of cooked and raw samples was determined using a reflectance colorimeter (CR-400, Konica, Minolta, Japan) with a light source of D65 and a measuring diameter of 8 mm. The lightness (L*), redness (a*), and yellowness (b*) were recorded. The WBSF was determined according to the method of Chao et al.11 Five rectangular meat slices (1 cm × 1 cm × 5 cm) were cut from the cooked meat along the fiber direction and sheared perpendicular to the muscle fiber direction using an HDP/BS adaptor (blade) on the texture analyzer (QTS-25, Northeast Agricultural University, China). The testing speed was 100 mm min−1, and the trigger force was 10 g. The WBSF was obtained as the maximum force during shearing and expressed in N.
2.3 Protein carbonyl content (PCC)
Protein carbonyl content is generally considered as protein oxidation markers,8 with higher levels of PCC indicating greater oxidation of the protein. The protein carbonyl content was determined using the commercial kits (A087, Nanjing Jiancheng Bioengineering Institute, Nanjing, China), and the steps were referred to the operating instructions of the kit. The absorbance was measured at 370 nm and the PCC was expressed as nanomoles of 2,4-dinitrophenylhydrazine (DNPH) per milligram of protein.
2.4 Total thiols (SH) and disulfide bonds (S–S) detection
The contents of the total SH and S–S groups were determined according to the method of Cui et al.18 Specifically, the cooked meat was homogenized using a homogenizer (T25 digital ULTRA-TURRAX Disperser, IKA, MA, USA), and the meat samples (0.3 g) were homogenized three times in 4.5 mL of 2% sodium dodecyl sulfate (SDS), each at 10
000 rpm for 30 s, cooling for 15 s between bursts. The homogenates were centrifuged at 4000 × g for 20 min at 4 °C. Protein concentration was determined by the BCA Protein Assay kit (Thermo Fisher Scientific, IL, USA). For determination of SH group levels, 0.5 mL of chicken breast homogenate was mixed with 2.5 mL of Tris–Gly–urea buffer (86 mM Tris, 0.09 M glycine, 5 mM EDTA, 8 M urea, pH 8.0) and 0.02 mL of 4 mg mL−1 5,5′-dithiobis-2,2′-nitrobenzoic acid (DTNB). After incubation at 25 °C for 30 min, the absorbance at 412 nm (A412) was recorded. The SH group level was calculated by the following formula: SH group level (μmol g−1 proteins) = 73.53A412 × D/C, where D was the dilution coefficient and C (mg mL−1) was the protein concentration.
To determine the level of the S–S group, 0.2 mL of chicken breast meat homogenate was added to 1.0 mL of Tris–Gly–urea buffer and 0.02 mL of mercaptoethanol. After incubation at 25 °C for 1 h, 10 mL of 12% (w/v) trichloroacetic acid was added and incubated for 1 h. Then the mixture was centrifuged at 3000 × g for 10 min. The pellet was dissolved in 3 mL Tris–Gly–urea buffer and 0.03 mL 4 mg mL−1 DTNB. After incubation at 25 °C for 30 min, the absorbance of the samples was recorded at 412 nm. The S–S group level was calculated according to the following formula: S–S group level (μmol g−1 chicken proteins) = 73.53A412 × D/C − SH group level.
2.5 Thiobarbituric acid reactive substances (TBARS)
The TBARS values were assessed by the method of He et al.19 with slight modifications. The minced cooked meat (2.0 g) was homogenized twice with 10 mL of 17.5% (v/v) trichloroacetic acid solution at 25
000 rpm for 10 s with a 30 s interval on ice using a homogeniser (T25 digital ULTRA-TURRAX Disperser). After the homogenate was filtered through with 8 mm filter paper, 1.0 mL of 0.02 M thiobarbituric acid solution was added to the filtrate and incubated in a boiling water bath for 40 min. After cooling in cold water, the solution was centrifuged at 2000 × g for 5 min at 4 °C. The supernatant was then collected and mixed with 1.0 mL of chloroform. The upper layer was collected for the absorbance measurement at 532 nm and 600 nm using a microplate reader (Multiskan FC ThermoFisher Scientific, USA). The TBARS content were calculated using the following formula: TBARS (mg kg−1) = (A532 − A600)/155 × (1/2) × 72.06 × 1000, where 155 and 1000 was the molar extinction coefficient (1.55 × 105 M−1 cm−1), 1/2 was the meat weight (2 g × 1/2), and 72.06 was the relative molecular mass of malondialdehyde (MDA). The values were expressed as mg of malondialdehyde per kg of meat.
2.6 Fluorescence measurements of tryptophan and schiff base structures (SB)
The fluorescence emission of Schiff bases and tryptophan was detected with reference to Gatellier et al.20 and Carvalho et al.16 Specifically, the meat sample (1.0 g) was homogenized (T25 digital ULTRA-TURRAX Disperser) with 5 mL of phosphate buffer solution (20 mM, 0.6 M NaCl, pH 6.5) for 30 s. Then 2 mL of homogenate was removed, and 8 mL of solvent (dichloromethane
:
ethanol (2
:
1, v/v)) was added with vortex for 30 s. After centrifugation of the mixture at 4000 × g for 10 min at 25 °C, the upper phase was collected for fluorescence intensity (FI) measurement by transferring 200 μL of supernatant to a 96-well plate. The emission spectra were scanned using a microplate reader (Infinite M200 Pro Multifunctional Enzyme Labeler, Tecan, Switzerland). The emission spectrum of tryptophan was recorded from 300 nm to 400 nm with an excitation wavelength of 285 nm. The emission spectrum of the Schiff base was recorded from 400 nm to 600 nm with the excitation wavelength at 360 nm. The fluorescence intensity was expressed in arbitrary units.
2.7 Sodium dodecyl sulfate-polyacrylamide gel electrophoresis (SDS-PAGE)
The SDS-PAGE was performed according to Yu et al.21 with minor modifications. Specifically, the meat sample (0.3 g) was homogenized with 4.5 mL of 2% SDS buffer at 10
000 rpm for 3 × 30 s and cooled for 15 s between bursts using a homogenizer (T25 digital ULTRA-TURRAX Disperser). The homogenate was centrifuged at 4000 × g for 20 min at 4 °C to obtain the supernatant. Protein concentration was determined and adjusted to 4 mg mL−1 with distilled water. The protein suspension was mixed with an equal volume of the sample loading buffer (100 mM Tris–HCl, 20% glycerol (w/w), 4% SDS (w/w), 0.05% bromophenol blue (w/v) with and without 5% β-mercaptoethanol (v/v)). The mixture was boiled at 100 °C for 3 min and stored at −80 °C until electrophoresis.
Subsequently, 20 μg of total protein was loaded onto an SDS-PAGE gel consisting of 4% stacking gel and 10% separating gel. Gels were run on a Bio-Rad Mini-Protean II electrophoresis unit (Bio-Rad Laboratories, Hercules, CA, USA) at 90 V for 30 min, and then at 120 V until the indicator line reached the bottom of the gel. Gels were stained with colloidal Coomassie brilliant blue solution (10% (v/v) glacial acetic acid and 0.025% (w/v) Coomassie brilliant blue R-250) for 1 h. Then gels were destained with 10% (v/v) methanol and 10% (v/v) glacial acetic acid for 24 h and scanned with the scanner Gel Doc XR+ system (Bio-Rad Laboratories, Hercules, CA, USA).
2.8 Microstructure detection
The slices of raw and cooked samples were cut into 1 cm × 1 cm × 0.5 cm along the myofibril direction. The slices were fixed in 2.5% glutaraldehyde in 0.1 M phosphate buffer, pH 7.3 for 2 h at room temperature. The test specimens were then rinsed with distilled water and gradually dehydrated (twice) in series solutions of 25, 50, 70, 95, and 100% ethanol, each incubated for 1 h. Samples were dried in liquid nitrogen and trimmed into 0.5 cm × 0.5 cm × 0.1 cm using a razor blade. The specimens were mounted on aluminum stubs and coated with gold (10−2 bar and 40 mA). The microstructure of the meat was examined by a scanning electron microscope (Gemini SEM 300, Carl Zeiss, Germany) with an accelerating voltage of 15 kV. Micrographs were captured at 400× magnification.
2.9 Statistical analysis
Data were expressed as the means ± standard deviation, and three independent triplicates were performed for all experiments. Comparisons were performed using mixed model analysis, and differences between individual means were assessed using the t-test (SPSS v.23.0). p < 0.05 was considered as a significant difference. Principal component analysis (PCA) was performed using the SIMCA software (v.14.1).
3. Results and discussion
3.1 pH
The pH value is considered as an important parameter to distinguish normal raw chicken and PSE-like chicken meat due to the difference in post-mortem glycolysis.16 As shown in Table 1, there were significant differences between normal and PSE-like meat cooked with different methods (p < 0.05). The pH in the MV group (p < 0.05) was significantly higher than that in the other three groups, which had no significant difference in pH (p > 0.05). In addition, compared with raw meat, the pH value increased significantly (p < 0.05) when the core temperature was heated to 60 °C. No further increase was detected as the core temperature increased to 70 and 80 °C (p > 0.05).
Table 1 Change of pH and color under chicken breast from normal (N) and PSE-like (P) with different cooking methods and core temperaturesa
Attribute |
Core temperature (°C) |
Treatment |
Cooking method |
Steaming |
Boiling |
Roasting |
Microwaving |
Note: (L*): lightness value, (a*): redness value, and (b*): yellowness value. Capital letters indicated the significant differences were found with in the same columns (p < 0.05). The lowercase letters indicated the significant differences were observed within the same row (p < 0.05). |
pH |
60 |
N |
5.94 ± 0.02Ab |
5.95 ± 0.03Ab |
5.95 ± 0.03Ab |
6.07 ± 0.03Aa |
P |
5.72 ± 0.02Bb |
5.74 ± 0.03Bb |
5.71 ± 0.05Bb |
5.88 ± 0.05Ba |
70 |
N |
5.92 ± 0.03Ab |
5.94 ± 0.03Ab |
5.97 ± 0.03Ab |
6.10 ± 0.04Aa |
P |
5.69 ± 0.02Bb |
5.71 ± 0.03Bb |
5.73 ± 0.05Bb |
5.84 ± 0.05Ba |
80 |
N |
5.93 ± 0.02Ab |
5.91 ± 0.03Ab |
5.94 ± 0.03Ab |
6.09 ± 0.04Aa |
P |
5.75 ± 0.02Bb |
5.72 ± 0.03Bb |
5.75 ± 0.05Bb |
5.89 ± 0.05Ba |
L* |
60 |
N |
82.56 ± 0.24Db |
81.01 ± 0.85Db |
79.87 ± 0.68Cc |
87.36 ± 0.44Aa |
P |
84.72 ± 0.66Bb |
83.77 ± 1.29Cb |
82.82 ± 0.05Bc |
87.56 ± 0.12Aa |
70 |
N |
84.86 ± 0.37Bb |
83.10 ± 0.88Cb |
82.01 ± 0.04Bc |
87.86 ± 0.48Aa |
P |
86.57 ± 0.14Ab |
86.52 ± 0.27Ab |
84.85 ± 1.06Ac |
87.25 ± 0.78Aa |
80 |
N |
86.52 ± 0.93Ab |
86.57 ± 0.31Ab |
85.33 ± 0.15Ac |
87.66 ± 0.80Aa |
P |
83.22 ± 0.23Cb |
84.51 ± 0.90Bb |
81.96 ± 0.27Dc |
87.85 ± 0.56Aa |
a* |
60 |
N |
3.22 ± 0.23Ab |
3.14 ± 0.10Ab |
2.98 ± 0.42Ac |
3.44 ± 0.16Aa |
P |
2.74 ± 0.33Bb |
2.68 ± 0.04Bb |
2.52 ± 0.12Bc |
3.16 ± 0.17Ba |
70 |
N |
2.72 ± 0.12Bb |
2.63 ± 0.18Bb |
2.41 ± 0.27Bc |
2.89 ± 0.26Ba |
P |
2.30 ± 0.26Cb |
2.27 ± 0.17Cb |
2.03 ± 0.09Cc |
2.57 ± 0.18Ca |
80 |
N |
2.06 ± 0.13Cb |
2.16 ± 0.38Db |
1.82 ± 0.08Dc |
2.53 ± 0.23Ca |
P |
1.78 ± 0.04Db |
1.80 ± 0.29Eb |
1.47 ± 0.16Ec |
2.42 ± 0.18Ca |
b* |
60 |
N |
14.48 ± 0.52Db |
14.52 ± 0.35Eb |
15.41 ± 0.44Ea |
13.82 ± 0.26Ec |
P |
16.86 ± 0.88Bb |
16.63 ± 0.34Cb |
17.64 ± 0.66Ca |
15.54 ± 0.23Cc |
70 |
N |
15.49 ± 0.56Cb |
15.70 ± 0.36Db |
16.94 ± 0.31Da |
14.85 ± 0.21Dc |
P |
17.31 ± 0.09Bb |
17.27 ± 0.12Bb |
18.95 ± 0.47Ba |
16.03 ± 0.22Bc |
80 |
N |
16.02 ± 0.19Cb |
16.40 ± 0.38Cb |
17.66 ± 0.52Ca |
15.04 ± 0.57Cc |
P |
18.44 ± 0.12Ab |
18.95 ± 0.51Ab |
19.87 ± 0.25Aa |
17.36 ± 0.24Ac |
The increase in pH of meat during cooking was mainly associated with protein denaturation and charge changes due to the loss of acidic groups and accumulation of alkaline groups.22 Becker et al.23 reported that the pH of pork loins was closely related to heating time when they were cooked in a steam convection oven at low temperatures of 53 °C and 58 °C for 20 h. In the present study, samples cooked at low temperatures have less protein denaturation and smaller pH values than those cooked at high temperatures. Moreover, it was found the pH value of the meat cooked for a long time was lower than that of the meat cooked for a short time.24 This finding is in agreement with the result in the current study that the MV group, which was cooked for a shorter time, presented a higher pH value than the other three groups.
3.2 Color
Color is an important indicator to characterize the quality and freshness of raw meat and the doneness of cooked meat.7 As presented in Table 1, there were significant differences in the color attributes of normal and PSE-like chicken meat between the four cooking methods. The MV treatment had the highest L* and a*, followed by steaming and boiling while roasting presented the lowest value. Consistent with the raw samples, normal chicken breasts possessed higher a* and lower L* than PSE-like meat by steaming, boiling, and roasting treatments (p < 0.05). When cooked by microwaving, there was no significant difference in L* between PSE-like and normal chickens (p > 0.05) but a significant difference in a* and b* values (p < 0.05).
Cooked meat displayed an off-white, grey, or brown appearance, depending on the extent of myoglobin denaturation and ferrihemochrome formation during cooking.25 The amount of ferrihemochrome was largely affected by the initial pH value of meat.16 The lower pH value of PSE-like chicken breasts than that of normal meat was mainly due to more protein denaturation and water loss, which promoted the formation of ferrihemochrome. Besides, PSE-like meat showed higher L* and b* values and a lower a* value compared with normal meat. Myoglobin denatured fastest at 55–65 °C and continued to denature at 75–80 °C, which explained the greatest color change at 60 °C, and a* value decreased significantly as the core temperature increased to 70 °C. In addition, it was observed that the color attributes of a* and L* in the normal sample at 70 °C were not significantly different from those of the PSE sample at 60 °C (p > 0.05). The core temperature of 70 °C was regarded as a safety standard for cooked meat.25 During the cooking of PSE-like chicken, using appearance as the only indicator might cause visual errors for consumers.
3.3 Cooking loss and Warner–Bratzler shear force
As shown in Fig. 2, the values of cooking loss and shear force presented an upward trend with the increase of the core temperature. Among the different cooking methods, the RO treatment had the highest cooking loss rate (NOR: 26.9% vs. PSE: 31.8%) and shear force (NOR: 78.5 N vs. PSE: 98.3 N), followed by the BO and ST treatments, and the lowest was found in the MV treatment (p < 0.05). As expected, except for the MV treated samples (p > 0.05), PSE-like samples possessed significantly higher cooking loss (NOR: 22.1% vs. PSE: 26.2%) and shear force (NOR: 50.4 N vs. PSE: 69.2 N) than normal samples under the other three cooking methods (p < 0.05). The ST-treated PSE-like samples showed a sharp drop in shear force at 80 °C (NOR: 52.5 N vs. PSE: 18.9 N), and the difference was significant for the normal samples (p < 0.05).
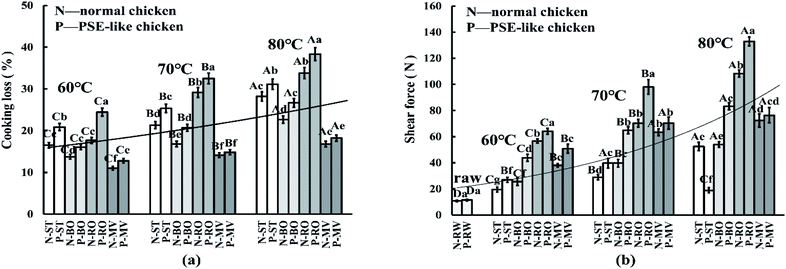 |
| Fig. 2 The cooking loss (a), shear force value (b) of PSE-like and normal chicken breast after cooking by roasting (RO), microwaving (MV), steaming (ST) and boiling (BO) at core temperature of 60, 70 and 80 °C. (RW) indicated raw meat, (N) indicated normal chicken breast, and (P) indicated PSE-like chicken breast. Capital letters indicated that there were significant differences between treatment groups with different core temperatures under the same cooking method (p < 0.05). The lowercase letters indicated that there were significant differences between treatment groups with different cooking methods at the same core temperature (p < 0.05). | |
The water loss in meat was closely related to the cooking method.26 The RO treatment required a higher temperature and more time to reach the corresponding core temperature than the MV treatment, resulting in a higher cooking loss rate and shear force in the RO group than in the MV group. This result is consistent with the findings of Półtorak et al.9 and Yarmand and Homayouni,27 who concluded that microwave heating could shorten cooking time, reduce cooking loss, and improve meat tenderness. In a previous study, the cooking loss was also significantly higher in PSE-like broiler breast fillets (26.2–26.4%) compared with normal broiler (21.0–23.0%) by roasting.4 It should be noted that the cooking loss of the PSE-like chicken group treated by MV at 70 °C and 80 °C was not significantly different from the normal chicken group (p > 0.05), probably due to the small sample size and the short cooking time. Another study has shown that low-temperature and high-speed heating might reduce the water loss rate of PSE-like chicken breasts.25 The change in the tenderness of cooked meat is mainly caused by heat-induced changes in muscle structure and protein denaturation during cooking. Heating can dissolve collagen, leading to a decrease in the shear force of the meat, and in contrast, heating can also denature myofibril protein, leading to stricter meat and increased shear force.28 It was believed that the difference in meat tenderness between PSE-like and normal chicken breasts was caused by more damaged myofibril in PSE-like meat (indicated in Section 3.7), which was consistent with the report of Desai et al.17 However, the ST-treated PSE-like samples appeared to have a sharp drop in shear force at 80 °C, possibly due to the influence of the heat transfer medium and the weak myofibril structure of raw PSE-like chicken.29 The water vapor and water molecules from steaming might directly penetrate into the muscle fiber tissues. High temperatures and humid air greatly damage muscle fiber tissues, resulting in a decrease in shear force.
3.4 Cooking-induced protein oxidation sensitivity and damage
The cooking process is one of the main factors that induce protein oxidation.30 As expected, cooking significantly increased the carbonyl content and decreased the tryptophan and thiols content as the core temperature increased (Fig. 3a, c, and d, p < 0.05). Among these groups, the RO group had the highest carbonyl content (NOR: 11.2 vs. PSE: 13.4, nmol mg−1 protein), followed by BO and ST groups, while the MV group showed the lowest carbonyl content (NOR: 5.1 vs. PSE: 5.6, Fig. 3a). Correspondingly, the MV treatment produced the highest tryptophan fluorescence and thiols content (NOR: 73.9 vs. PSE: 68.8, nmol mg−1 protein), followed by ST and BO, and the RO group (thiols, NOR: 41.3 vs. PSE: 33.7) possessed the lowest (Fig. 3b, p < 0.05). PSE-like chicken breast from the ST, BO, and RO treatment groups presented significantly higher carbonyl content and lower tryptophan and thiols content compared with the normal chicken breast (p < 0.05). However, there was no significant difference between the two chicken groups by the MV treatment (p > 0.05), suggesting that the MV treatment exhibited minimal impact on the difference in protein oxidation between PSE-like and normal chicken breast. Malondialdehyde (MDA) is a signature product of lipid oxidation and can react with amino acid side chains, leading to the formation of carbonyl groups.31 As shown in Fig. 3b, cooking significantly in-creased the MDA content as the core temperature increased (p < 0.05). The highest MDA content was observed in the BO treatment (NOR: 0.54 vs. PSE: 0.68, mg kg−1 meat), followed by the ST treatments (NOR: 0.48 vs. PSE: 0.60) and RO treatments (NOR: 0.46 vs. PSE: 0.57), while the lowest MDA content was found in the MV treatments (NOR: 0.42 vs. PSE: 0.44). In normal and PSE-like chicken samples, the ST and BO treatments caused the greatest difference in MDA content (p < 0.05), followed by the RO treatment (p < 0.05), while the MV treatment caused insignificant differences (p > 0.05).
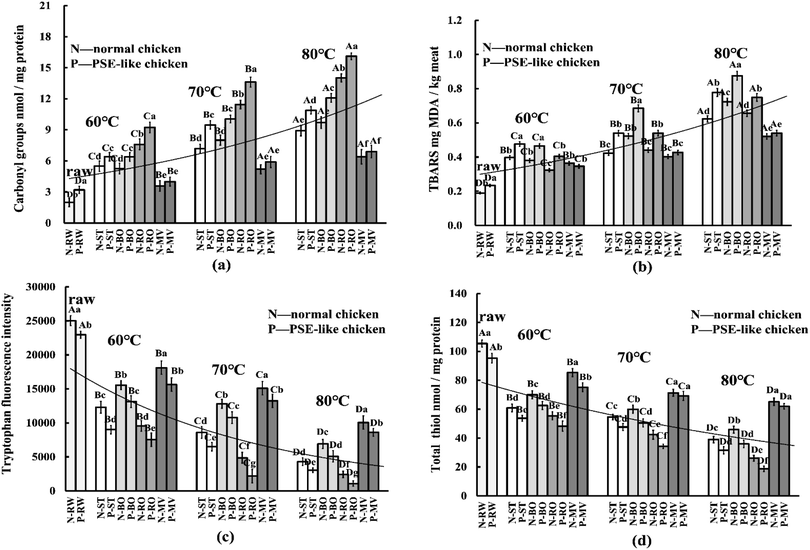 |
| Fig. 3 The carbonyl value (a), thiobarbituric acid-reactive substances (b), tryptophan fluorescence intensity (c) and total thiols value (d) of PSE-like and normal chicken breast under different cooking methods and different core temperatures (including raw meat samples). Abbreviation: RW: raw meat, RO: roasting, MV: microwaving, ST: steaming, BO: boiling. (N) indicated normal chicken breast, and (P) indicated PSE-like chicken breast. Capital letters indicated that there were significant differences between treatment groups with different core temperatures under the same cooking method (p < 0.05). The lower letters indicated that there were significant differences between treatment groups with different cooking methods at the same core temperature (p < 0.05). | |
As mentioned above, PSE-induced chicken breast showed higher oxidative sensitivity, and the heat treatment process exacerbated the increase in protein oxidation in PSE-induced chicken breasts, which in turn led to greater oxidative damage to the meat. In RW samples, the depletion of tryptophan and thiols and the increase in carbonyl and TBARS content were more severe in PSE-induced chicken breasts than in normal samples. Tryptophan and thiol residues from sulfurcontaining amino acids are preferred targets of reactive oxygen species (ROS), and hence are particularly susceptible to oxidative reactions. In addition, PSE-induced chicken breasts have a lower pH, which may promote the oxidative cycle of iron and enhance protein carbonylation.16,30 Moreover, there is an indirect reaction between lipid oxidation (TBARS) and protein oxidation, leading to a greater degree of protein carbonylation formation.31 Similar results showed that PSE-induced chicken breasts had higher protein hydrolysis and lower endogenous antioxidant enzymes activities, which was related to protein oxidation sensitivity.16 This result suggests that PSE-induced chicken breasts have a higher oxidative sensitivity before and during cooking, causing a higher degree of oxidative damage than normal chicken breasts.
During cooking, heat treatment led to a further increase in protein oxidative sensitivity and oxidative damage in PSE-like meat. The difference was constantly influenced by the cooking techniques and can be manifested by a significant difference in protein cross-linking and aggregation between PSE-like and normal meat. The phenomenon may be due to the loss of natural antioxidant properties of the meat in the early stages of heat treatment and the generation of free radicals at sustained high temperatures.15,30 High temperature can destroy the cellular structure of the meat, exposing it to oxygen and triggering a continuous attack of ROS.32 Under a continuous attack of heat-induced ROS, lipid oxidation was increased (Fig. 3b), and in turn, free radicals generated by lipid oxidation were transferred to proteins, thus promoting the degree of protein oxidation.31 As the core temperature increased, cooking methods resulted in significant differences in the cooking loss. Tryptophan and thiols might be affected and lost during cooking, possibly leading to a decrease in tryptophan and total thiols (Fig. 3c and d). In addition, the oxidation of tryptophan and thiols is due to the release of catalytic iron in the heme molecule and the inactivation of enzymes induced by high temperatures during cooking, and the amount of ferrihemochrome pigment is largely influenced by the initial meat pH.16 The oxidative breakage of the porphyrin ring occurred during heating, and heme iron (one of the pro-oxidants) was released, which promoted the Fenton/Fenton-like oxidation reaction and accelerated the deterioration of protein oxidation.15 This also resulted in greater protein oxidative damage in PSE-induced chicken breasts. In the current study, RO caused the highest degree of protein oxidation, while MV showed the lowest, corresponding to the difference in core temperature and increase rates. However, except for the RO group, the highest MDA content was detected in the BO group. Utrera et al.33 reported that roasting at 170 °C for 18 min reduced the MDA content in the beef patty compared with the raw beef patty. Hu et al.15 also reported that roasting caused the least MDA in sturgeon meat compared with steaming, boiling, frying, and microwaving. It was suggested that the decrease in MDA content in the RO group was due to the further conversion of lipid oxidation products such as aldehydes to volatile compounds at relatively high temperatures and prolonged heating.34 For normal and PSE-like samples, the differences in the degree of protein oxidation caused by the ST and BO treatment were prominent, but not significant for the MV treatment. Sobral et al.1 indicated that meat cooked by microwaving to a core temperature of 85 °C showed little impact on the formation of carbonyl groups, which was consistent with this study. Differences in carbonyl, tryptophan and thiols content are solid evidence that cooking methods and processes largely affect protein oxidative damage.
3.5 Protein cross-linking
Both Schiff base (SB) and disulfide bond (S–S) are indicators for protein cross-linking in cooked meat.35 As shown in Fig. 4a and b, the contents of disulfide bonds gradually increased as the core temperature increased, both significantly higher than the raw meat (p < 0.05). The highest content of disulfide bonds was in the RO treatment, followed by the ST and BO treatments, while the MV treatment showed the lowest (p < 0.05). The content of disulfide bonds of PSE-like chicken meat was significantly higher than that of normal meat under ST, BO, and RO treatments. The SB in cooked meat had a characteristic absorption peak at 412 nm in fluorescence emission spectra, as shown in Fig. 4b. The PSE-like chicken meat treated with RO showed the highest fluorescence intensity of SB at 60–80 °C than other cooking treatments. The MV, ST, and BO treatments showed comparable fluorescence intensity to SB at 60 °C, but showed gradual differences at 70 °C and 80 °C, suggesting a higher degree of protein cross-linking during prolonged cooking. Compared with normal meat, cross-linking was more pronounced in PSE meat.
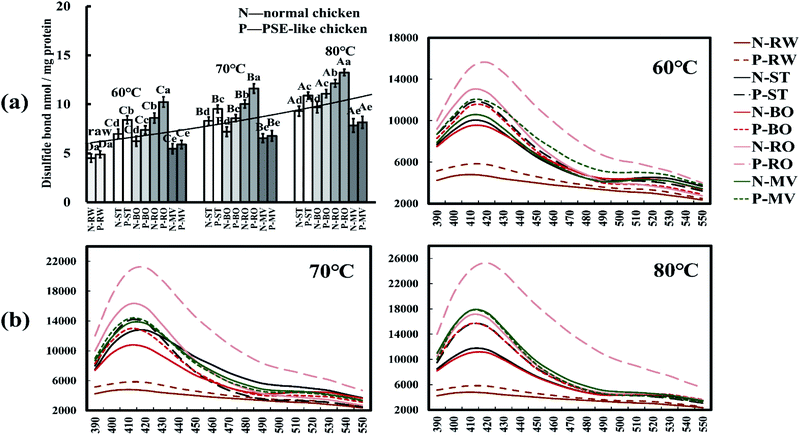 |
| Fig. 4 The disulfide bond (a) and Schiff base (b) of PSE-like and normal chicken breast under different cooking methods and different core temperatures (including raw meat samples). Abbreviation: RW: raw meat, RO: roasting, MV: microwaving, ST: steaming, BO: boiling. (N) indicated normal chicken breast, and (P) indicated PSE-like chicken breast. In (b), the dotted line (- - - -) represented normal chicken breast, and the solid line (—) represented PSE-like chicken breast. Capital letters indicated that there were significant differences between treatment groups with different core temperatures under the same cooking method (p < 0.05). The lower letters indicated that there were significant differences between treatment groups with different cooking methods at the same core temperature (p < 0.05). | |
The fluorescent products (SB) and disulfide bonds are believed to contribute to the formation of insoluble protein aggregates, leading to shrinkage, water loss, and toughness of meat during cooking.32 Consistent with Hu et al.,15 roasting generated higher SB content than boiling, steaming, microwaving, and frying cooking, which can be attributed to possible interactions between protein and lipid oxidation products. In the current study, the high content of SB and disulfide bonds in the RO treatment corresponded to the high cooking loss and meat shear force. Several studies showed that the formation of protein cross-linking in muscle had been considered a relevant mechanism for meat toughening.32 In addition, raw PSE-like chicken meat had slightly higher SB and S–S levels than normal meat, confirming that proteins in PSE-like meat have higher oxidative sensitivity and produce some cross-linking. It has been reported that there is a link between protein carbonylation and Schiff bases,30 while the loss of thiols leading to the formation of disulfide bonds.16 In cooked meat, PSE-like chicken also showed more protein cross-linking and differences. It is worth emphasizing that the degree of disulfide bond growth with increasing core temperature is not as great as the extent of thiols depletion (Fig. 3d and 4a). This result suggests that the growth of disulfide bonds does not explain the total depletion of thiols, implying that the oxidation of thiols at least partially follows a mechanism other than disulfide bond formation and is continuously influenced by cooking conditions.
3.6 SDS-PAGE
Non-reduced and reduced SDS-PAGE was performed to observe changes in protein aggregation by cooking at 70 °C (Fig. 5). Compared with the reduced gels, the remarkable changes in protein bands were the loss of myosin heavy chain (MHC) and the increase in protein aggregation at the top of the non-reduced gels. It was consistent with MHC being distinctively intolerant to heating.36,37 Compared to the RW sample, MV had the least effect on protein profile, while RO, BO, and ST significantly affected the degree of protein aggregation by showing with the top bands. Moreover, myosin heavy chain (MHC) was apparently lost in the BO and ST samples under non-reduced conditions, while most of the lost MHC was recovered under the reduced conditions. Unlike BO and ST, less missing MHC was recovered in RO samples in reduced gel, indicating the differences in cooking methods significantly affected the degree of protein aggregation at the same core temperature. The performance of PSE-like meat was constantly influenced by the cooking method. Furthermore, protein cross-linking is associated with the aggregation of meat protein during cooking, including dityrosine, Schiff bases, disulfide bridges, and protein carbonylation.30 At the beginning of cooking, protein aggregation has little effect due to low protein oxidation and low cross-linking of disulfide bonds formed by cysteine oxidation. With the constant influence of cooking temperature, time, and medium, excessive protein oxidation can lead to the formation of other covalent bonds (Schiff bases), which further promote protein aggregation. Compared with MHC, actin was more stable during heating, and there was almost no obvious change in the protein bands between the different cooking treatment groups. This result is consistent with other reports that the action bands showed no visible changes before heating at 140 °C for 20 min.36 The different observations in SDS-PAGE may be due to different degrees of protein oxidation, which is evident in carbonyl content, thiols content, disulfide bond content, and Schiff base content (Fig. 3 and 4).
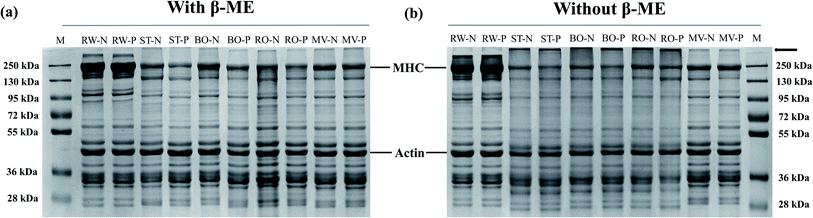 |
| Fig. 5 The SDS-PAGE of protein samples by roasting (RO), microwaving (MV), steaming (ST) and boiling (BO) to the core temperature of 70 °C. In the (a) and (b) indicated the reduced and nonreduced gel by adding and without adding β-mercaptoethanol, respectively. Abbreviation: M: protein standard marker, RW: raw meat, N: normal chicken breast, P: PSE-like chicken breast, MHC: myosin heavy chain. | |
3.7 Microstructure analysis
The meat quality was largely determined by the integrity and shrinkage of myofibrils during cooking.27 As shown in Fig. 6, the cross-section of meat cooked at 70 °C showed shrinkage and dissolution, losing their original arrangement of muscle cells compared with the RW sample. A significant difference could be found between raw samples of normal and PSE-like chicken meat. PSE-like samples had larger gaps between muscle fiber tissues, which is consistent with the poor water retention and loose texture of PSE-like chicken meat, and it is constantly affected during cooking. It can be seen that the gaps between muscle cells were larger in the RO and ST treatment groups compared with other cooking methods. PSE-like samples presented more disruption of muscles than normal meat, while the MV treatment showed less effects.
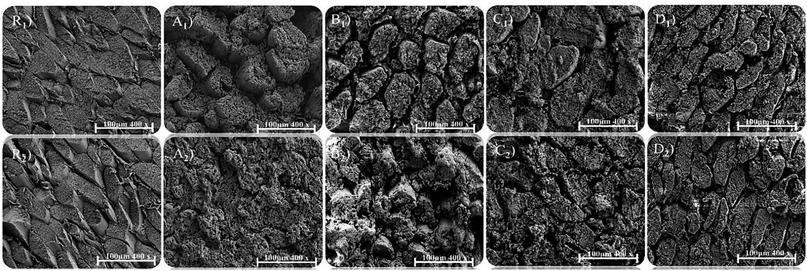 |
| Fig. 6 The transverse microstructure of PSE-like and normal chicken breast under different cooking methods and at 70 °C. In the picture, the subscript number of 1 represented normal chicken breast, and subscript number of 2 represented PSE-like chicken breast. The capital of R, A, B, C, and D indicated raw meat, steaming, boiling, roasting and microwaving, respectively. | |
The heat transfer principle of the ST treatment was the heat convection and transfer of water vapor, which directly impacted the muscle surface, cooled, and gathered on the surface. Water vapor would invade the muscle tissue structure and dissolve muscle fiber tissue, resulting in a reduction in shear force. The RO treatment was done in an air convection oven, in which heat was transferred by convection of dry-hot air to replace moisture. High temperatures and dry air disrupted the connections between muscle fibers and exacerbated the loss of tissue alignment, causing a severe loss of moisture and increased shear forces of the meat. BO and MV treatments show less damage to muscle tissues. Under the two treatments, myofibrils are still tightly attached to the muscle membrane. Boiling in a sealed bag allows indirect contact and penetration of water into the muscle with relatively less effect on the tissue structure. Even so, the microstructure of boiled PSE-like meat showed that the muscle structure disintegrated during heating, and the tissue also experienced slight swelling, and myofibril tissue was dissolved to a certain extent. The MV treatment had little effect on the microstructural differences between the cooked meat cross-sections of PSE-like and normal samples. Microwave heating is characterized by short startup time and fast heating rates due to the geometric and dielectric properties of the heated material.38 The principle of the microwave was that the electromagnetic waves generated by the magnetron in the microwave oven cause the water molecules in the food to vibrate. Water accounted for 74% of chicken meat with a high dielectric coefficient, and it was also the main substance that absorbed MV-treated energy.39 Without the intervention of an external heat transfer medium, the high-efficiency magnetic field vibrates the water molecules inside the muscle, achieving a high cooking efficiency with tissue structure remained intact. This result highlights that differences in the heat transfer method and medium are also important factors affecting the meat quality at the same core temperature.
3.8 Dependencies between meat quality and protein oxidation
The meat quality and oxidation properties of normal and PSE-like samples by cooking at a core temperature of 60, 70, and 80 °C were investigated using PCA analysis (Fig. 7). Strong links were found between cooking methods, core temperatures, protein oxidative damage, and meat quality. Meat quality and oxidative damage indicators were in the same quadrant, indicating a high correlation between oxidative damage and meat quality (Fig. 7a). Furthermore, the changes in protein carbonyl, thiols, Schiff base, and disulfide bonds were highly consistent with the trends in shear force at the same core temperature for different cooking methods. The increases in disulfide bonds and Schiff bases are often thought to promote the strengthening of protein structures in muscle tissue, leading to the mechanism of meat toughening.32 This phenomenon suggests that protein oxidation plays a role in meat tenderness. In Fig. 7b, as the core temperature increased, the mapping point of cooked meat that was completely separated from the RW samples moved forward from left to right in PCA plots. The change indicated that the meat quality and oxidation profile were largely influenced by the heating temperature. Among the cooking methods, the RO treatment was clearly scattered from other groups, showing the most significant impact on meat quality and oxidation profile. Although roasting can significantly improve the color and flavor of meat,10 it can also result in higher cooking loss rates (Fig. 2a), higher oxidation levels (Fig. 3 and 4), and toughness of the meat (Fig. 2b). When the maximum upper limit temperature was the same, BO and ST cooking showed different distributions of the PCA mapping points, indicating that the heat transfer medium and heat transfer method were key factors affecting meat quality and oxidation. These factors can cause serious damage to the tissue structure of PSE meat (indicated in Section 3.7). The moving amplitude of PCA mapping points under MV treatment is lower than the other three cooking methods, and the points are relatively concentrated. The mapping points of PSE-like and normal samples were very close by the MV treatment at 70 °C and 80 °C. This distribution indicated that the difference in meat quality and oxidation between normal chicken and PSE-like chicken caused by microwave cooking was the smallest. Through this experiment, the importance of the heating time and the absence of external heat transfer medium intervention in reducing oxidative damage and quality deterioration of cooked meat can be emphasized. Moreover, the PSE samples mostly differed from normal samples and showed a clear distance in the PCA plot, implying the importance of raw meat quality on cooked meat.
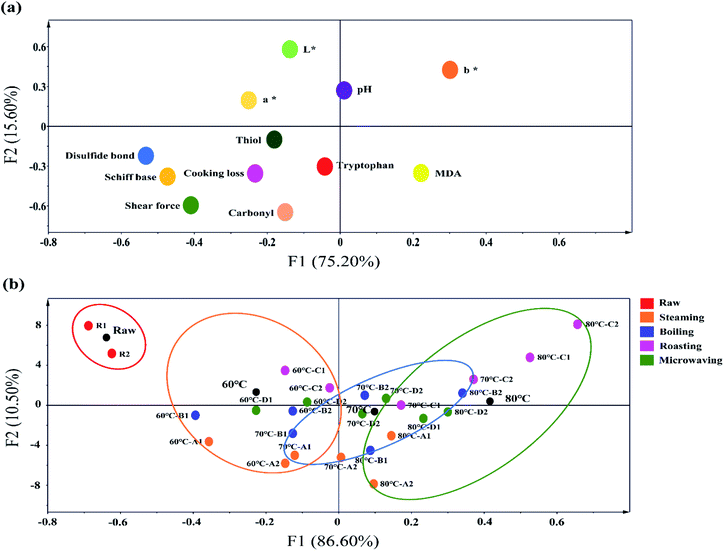 |
| Fig. 7 The principal component analysis diagram (PCA) of various indexes of normal chicken breast and PSE-like chicken breast under different cooking methods and different core temperatures. In the (a) and (b) indicate the PCA of various indexes and the PCA of different cooking methods and different core temperatures, respectively. Abbreviation: M: protein standard marker, RW: raw meat, N: normal chicken breast, P: PSE-like chicken breast, MHC: myosin heavy chain. | |
4. Conclusions
In this study, meat quality, oxidation profile, and microstructure of PSE-like chicken breast after the cooking with four methods and under three core temperatures were comprehensively investigated and compared with those of normal chicken breast. Results showed that PSE-like chicken breast had an inferior meat quality and a higher oxidative sensitivity compared to normal chicken meat. The heat treatment exacerbated the increase in protein oxidation and meat quality defects in PSE-like chicken breasts. Moreover, the differences in cooked PSE and normal chicken were constantly influenced by cooking methods. There is also a relationship between oxidation and quality, which is more evident in the PSE samples. In addition to cooking temperature and time, the heat transfer methods and the heat transfer medium also significantly affect the quality of PSE meat. Roasting exhibited convection of dry air at high temperatures, resulting in most cooking losses, meat toughness, excessive oxidation and aggregation of the proteins. Due to the longer times of steaming and boiling, conduction and convection heat transfer and the heat transfer medium (water vapor) caused greater damage and dissolution to the myofibrils. Microwave cooking involves no external heat transfer medium and has a faster heat transfer rate, resulting in little effect on the microstructure and oxidation characteristics of the cooked PSE-like chicken. This study suggested that microwaving is a preferable domestic cooking method for PSE-like chicken, which reduces the difference in meat quality and oxidation profile between PSE-like and normal chicken meat. In practice, this study will help to better elucidate the relationship between oxidation profile and meat quality, and provide consumers with recommendations for consuming PSE-like meat.
Author contributions
Conceptualization: R. L., X. Y. Z.; methodology: T. Y. Y.; software: H. Y., W. X. Y.; validation: R. L., X. Y. Z., T. Y. Y.; formal analysis: T. Y.Y.; investigation: L. Y., K. Y. L., M. Q.; resources: R. L., X. Y. Z.; data curation: R. L.; writing – original draft preparation: T. Y. Y.; writing – review and editing: R. L.; visualization: Q. F. G., M. G. W.; supervision: X. Y. Z.; project administration: R. L.; funding acquisition: R. L.
Conflicts of interest
The authors declare that they have no known competing financial interests or personal relationships that could have influenced the work reported in this paper.
Acknowledgements
We thank, for their technical support, Opening research of Key Laboratory of Chinese Cuisine Intangible Cultural Heritage Technology Inheritance, Ministry of Culture and Tourism, and Industrial Engineering Center for Huaiyang Cuisine of Jiangsu Province. This research was funded by the Natural Science Foundation of Jiangsu Province (BK20190888) and the National Natural Science Foundation of China (No. 31901610), Fellowship of China Postdoctoral Science Foundation (2020M671626).
Notes and references
- M. Sobral, S. Casal, M. A. Faria, S. C. Cunha and I. M. P. L. V. O. Ferreira, Food Chem. Toxicol., 2020, 141, 111401 CrossRef CAS PubMed
. - H. Q. Chen, H. H. Wang, J. Qi, M. Y. Wang, X. L. Xu and G. H. Zhou, Int. J. Food Sci. Technol., 2018, 53, 654–664 CrossRef CAS
. - S. Barbut, Poult. Sci., 2009, 88, 1506–1512 CrossRef CAS PubMed
. - R. Woelfel, C. Owens, E. Hirschler, R. Martinez-Dawson and A. Sams, Poult. Sci., 2002, 81, 579–584 CrossRef CAS PubMed
. - X. S. Zhu, X. L. Xu, H. H. Min and G. H. Zhou, J. Integr. Agric., 2012, 11, 1384–1390 CrossRef
. - B. K. Tiwari and C. O'Donnell, Thermal processing of meat and meat products, in Thermal food processing: new technologies and quality issues, ed. D. W. Sun, CRC Press, USA, 2012, pp. 195–220 Search PubMed
. - P. B. Pathare and A. P. Roskilly, Food Eng. Rev., 2016, 8, 435–447 CrossRef
. - M. M. C. Sobral, S. C. Cunha, M. A. Faria and I. M. Ferreira, Compr. Rev. Food Sci. Food Saf., 2018, 17, 309–333 CrossRef CAS PubMed
. - A. Półtorak, J. Wyrwisz, M. Moczkowska, M. Marcinkowska-Lesiak, A. Stelmasiak, U. Rafalska, A. Wierzbicka and D. W. Sun, Int. J. Food Sci. Technol., 2015, 50, 958–965 CrossRef
. - W. Chumngoen, C. F. Chen and F. J. Tan, J. Anim. Sci., 2018, 89, 193–201 CrossRef CAS PubMed
. - C. Li, D. Y. Wang, W. M. Xu, F. Gao and G. H. Zhou, LWT–Food Sci. Technol., 2013, 51, 266–274 CrossRef CAS
. - X. Wang, X. Wang, B. Muhoza, T. Feng, S. Xia and X. Zhang, Innovative Food Sci. Emerging Technol., 2020, 62, 102344 CrossRef CAS
. - M. Estévez and C. Luna, Crit. Rev. Food Sci. Nutr., 2017, 57, 3781–3793 CrossRef PubMed
. - B. Mitra, R. Lametsch, T. Akcan and J. Ruiz-Carrascal, Meat Sci., 2018, 140, 134–144 CrossRef CAS PubMed
. - L. L. Hu, S. J. Ren, Q. Shen, J. C. Chen, X. Q. Ye and J. G. Ling, RSC Adv., 2017, 7, 27496–27505 RSC
. - R. H. Carvalho, E. I. Ida, M. S. Madruga, S. L. Martínez, M. Shimokomaki and M. Estévez, Food Chem., 2017, 215, 129–137 CrossRef CAS PubMed
. - M. A. Desai, V. Jackson, W. Zhai, S. P. Suman, M. N. Nair, C. M. Beach and M. W. Schilling, Poult. Sci., 2016, 95, 2696–2706 CrossRef CAS PubMed
. - C. Cui, X. S. Zhou, M. M. Zhao and B. Yang, Innovative Food Sci. Emerging Technol., 2009, 10, 37–41 CrossRef CAS
. - J. He, C. L. Xia, Y. X. He, D. D. Pan, J. X. Cao, Y. Y. Sun and X. Q. Zeng, Food Chem., 2019, 295, 129–137 CrossRef CAS PubMed
. - P. Gatellier, V. Sante-Lhoutellier, S. Portanguen and A. Kondjoyan, Meat Sci., 2009, 83, 651–656 CrossRef CAS PubMed
. - T. Y. Yu, J. D. Morton, S. Clerens and J. M. Dyer, J. Agric. Food Chem., 2015, 63, 9112–9123 CrossRef CAS PubMed
. - R. Hamm and F. Deatherage, J. Food Sci., 1960, 25, 587–610 CrossRef CAS
. - A. Becker, A. Boulaaba, S. Pingen, C. Krischek and G. Klein, Meat Sci., 2016, 118, 82–88 CrossRef CAS PubMed
. - M. Karpińska-Tymoszczyk, A. Draszanowska, M. Danowska-Oziewicz and L. Kurp, Food Sci. Technol. Int., 2020, 26, 563–573 CrossRef PubMed
. - N. J. King and R. Whyte, J. Food Sci., 2006, 71, 31–40 CrossRef
. - J. Yancey, M. Wharton and J. Apple, Meat Sci., 2011, 88, 1–7 CrossRef CAS PubMed
. - M. Yarmand and A. Homayouni, Food Chem., 2009, 112, 782–785 CrossRef CAS
. - C. Li, D. Y. Wang, H. Dong, W. M. Xu, F. Gao, G. H. Zhou and M. H. Zhang, J. Sci. Food Agric., 2013, 93, 1979–1985 CrossRef CAS PubMed
. - B. Mora, E. Curti, E. Vittadini and D. Barbanti, Meat Sci., 2011, 88, 489–497 CrossRef CAS PubMed
. - T. Y. Yu, J. D. Morton, S. Clerens and J. M. Dyer, Compr. Rev. Food Sci. Food Saf., 2017, 16, 141–159 CrossRef CAS PubMed
. - W. Zhang, S. Xiao, E. J. Lee and D. U. Ahn, J. Agric. Food Chem., 2011, 59, 969–974 CrossRef CAS PubMed
. - O. Soladoye, M. Juárez, J. Aalhus, P. Shand and M. Estévez, Compr. Rev. Food Sci. Food Saf., 2015, 14, 106–122 CrossRef CAS PubMed
. - M. Utrera, D. Morcuende and M. Estévez, LWT–Food Sci. Technol., 2014, 56, 62–68 CrossRef CAS
. - M. Roldan, T. Antequera, M. Armenteros and J. Ruiz, Food Chem., 2014, 149, 129–136 CrossRef CAS PubMed
. - M. Estévez, Meat Sci., 2011, 89, 259–279 CrossRef PubMed
. - K. Kajak-Siemaszko, L. Aubry, F. Peyrin, M. L. Bax, P. Gatellier, T. Astruc, W. Przybylski, D. Jaworska, B. Gaillard-Martinie and V. Santé-Lhoutellier, Food Res. Int., 2011, 44, 3160–3166 CrossRef CAS
. - S. Y. Wen, G. H. Zhou, L. Li, X. L. Xu, X. B. Yu, Y. Bai and C. B. Li, J. Agric. Food Chem., 2015, 63, 250–261 CrossRef CAS PubMed
. - C. Contreras, M. Benlloch-Tinoco, M. Rodrigo and N. Martinez-Navarrete, Impact of microwave processing on nutritional, sensory, and other quality attributes, in The microwave processing of foods, ed. M. Regier, K. Knoerzer and H. Schubert, CRC Press, UK, 2017, pp. 65–99 Search PubMed
. - M. Yarmand and A. Homayouni, Meat Sci., 2010, 86, 451–455 CrossRef CAS PubMed
.
|
This journal is © The Royal Society of Chemistry 2022 |
Click here to see how this site uses Cookies. View our privacy policy here.