DOI:
10.1039/D2RA00010E
(Paper)
RSC Adv., 2022,
12, 3569-3572
Nickel-catalysed cross-electrophile coupling of aryl bromides and primary alkyl bromides†
Received
2nd January 2022
, Accepted 11th January 2022
First published on 26th January 2022
Abstract
The structure of primary alkylated arenes plays an important role in the molecular action of drugs and natural products. The nickel/spiro-bidentate-pyox catalysed cross-electrophile coupling of aryl bromides and primary alkyl bromides was developed for the formation of the Csp2–Csp3 bond, which provided an efficient method for the synthesis of primary alkylated arenes. The reactions could tolerate functional groups such as ester, aldehyde, ketone, ether, benzyl, and imide.
Introduction
Numerous attractive synthesis approaches to primary alkylated arenes have been developed, primarily based on the transition metal-catalysed addition of nucleophiles to electrophiles.1 Cross-electrophile coupling represents an important field in these modern organic synthesis reactions.2 In general, the direct use of electrophiles is convenient because electrophiles are more accessible and easier to use than nucleophiles in the coupling reactions. The cross electrophile coupling reactions catalysed by nickel metal catalysts can provide effective methods for the construction of new C–C bonds in drugs and natural products.2b,3 Previous studies have established that 5-HT2A agonists have been implicated in cardiovascular function4 (Scheme 1). A class of histone deacetylase inhibitors has been generated from N-(2-amino-4-pyridyl)benzamide derivatives, which could be applied in the treatment of cancer, leukemia, and diseases related to differentiation and proliferation.5 Clobenpropit, a histamine H3-receptor antagonist, shows good activity in vitro at subnanomolar concentrations.6 Additionally, there are other alkylated alkanes derived from biologically important molecules such as estrones.7 Therefore, the development of a tremendous cross-electrophile coupling reaction between alkyl bromides and aryl bromides is still widely sought.
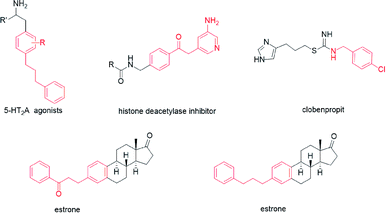 |
| Scheme 1 Drugs with the structure of alkylated arenes. | |
Recently, Charboneau et al.8 used a dual metal catalytic system for cross-electrophile coupling reactions between aryl halides and alkyl halides (Scheme 2a). Perkins et al.9 demonstrated that the cross-electrophile coupling reaction catalysed by nickel catalyst could be achieved under metal-reductant-free electrochemical conditions (Scheme 2b). As part of an effort to develop nickel-catalysed cross-electrophile coupling reactions, we previously achieved a direct cross-electrophile coupling of cyclic secondary alkyl bromides with aryl bromides.10 Owing to the good catalytic effect of spiro-bidentate-pyox ligands, we reasoned that they may also have a good catalytic effect in other types of cross-electrophile coupling reactions, which could lead to alkylated arenes (Scheme 2c).
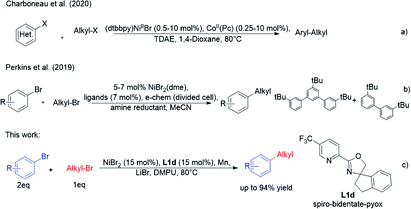 |
| Scheme 2 Ni-catalysed cross-electrophile coupling between aryl bromides and primary alkyl bromides. | |
Results and discussion
To identify whether the primary alkyl bromides and aryl bromides were competent, the coupling reaction of 4-bromobenzoate 1a with 1-bromo-3-phenylpropane 2a was surveyed (Table 1). Initial tests of nickel salts as metal catalysts for the cross-electrophile couplings were carried out using manganese as the reductant and N-methylpyrrolidone (NMP) as the solvent. Good catalytic performance was achieved using NiBr2 as the metal catalyst (entry 1). When NiBr2 was replaced by NiI2, NiBr2·glyme, or NiCl2, the yield of the desired cross-coupling product 3a decreased (entries 2–4). The desired product was not detected when the organic nickel catalyst Ni(acac)2 was used (entry 5). In order to optimize the reaction with different structures of the catalysts, we synthesized ligands with a substituted group on the pyridyl and phenyl ring L1b–L1d. Using NiBr2 as the metal catalyst, ligand L1d with an electron-withdrawing group at the C5-position on the pyridine ring gave the cross-coupling product 3a in 72% yield, which is superior to the ligands L1a and L1c with electron-neutral or electron-donating groups as well as ligand L1b with a substituent group on the phenyl ring (entries 6–8). Other bidentate ligands, like L2–L5, did not yield better results (entries 9–12). Decreasing the temperature from 80 °C to 60 °C produced 3a only in 39% yield (entry 13). Raising the temperature to 100 °C also resulted in a dramatic decrease in the yield (entry 14). In addition, no desired product was detected without NiBr2 or L1d, which implied that the metal and ligand were indispensable for the coupling reactions (entries 15–16).
Table 1 Scope of metal catalysts, ligands, and temperaturea,b
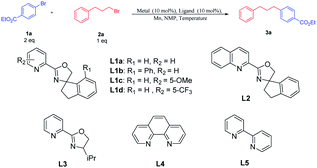
|
Entry |
Metal |
Ligand |
Temperature |
Yield (%) |
Reaction conditions: 1a (0.40 mmol), 2a (0.20 mmol), Mn (0.60 mmol), ligand (0.02 mmol), metal (0.02 mmol), NMP (1 mL). Isolated yield. No reaction. |
1 |
NiBr2 |
L1a |
80 °C |
67 |
2 |
NiI2 |
L1a |
80 °C |
62 |
3 |
NiBr2·glyme |
L1a |
80 °C |
56 |
4 |
NiCl2 |
L1a |
80 °C |
Trace |
5 |
Ni(acac)2 |
L1a |
80 °C |
NRc |
6 |
NiBr2 |
L1b |
80 °C |
35 |
7 |
NiBr2 |
L1c |
80 °C |
25 |
8 |
NiBr2 |
L1d |
80 °C |
72 |
9 |
NiBr2 |
L2 |
80 °C |
Trace |
10 |
NiBr2 |
L3 |
80 °C |
10 |
11 |
NiBr2 |
L4 |
80 °C |
55 |
12 |
NiBr2 |
L5 |
80 °C |
35 |
13 |
NiBr2 |
L1d |
60 °C |
49 |
14 |
NiBr2 |
L1d |
100 °C |
39 |
15 |
— |
L1d |
80 °C |
0 |
16 |
NiBr2 |
— |
80 °C |
0 |
Following the evaluation of the metal catalysts, ligands, and temperatures, other reaction conditions were screened. Among the amide solvents,11 NMP can reach 72% yield under the reaction conditions (Table 2, entry 1). When N,N-dimethylformamide (DMF) and N,N-dimethylacetamide (DMA) were used as solvents, the yields were both decreased (entries 2 and 3). 1,3-Dimethyltetrahydropyrimidin-2(1H)-one (DMPU) proved to be the best solvent; the yield could reach 76% (entry 4). Catalyst loading screening found that the yield of 3a was promoted to 84% when the amounts of NiBr2 and L1d were both increased to 15 mol% (entry 6), but the yield of 3a decreased when the loading of the metal and ligand were increased to 20 mol% or decreased to 7.5 mol% (entries 5 and 7). Further optimization found that the use of zinc instead of manganese as a reductant led to low yield (entry 8). The addition of one equivalent of lithium bromide boosted the yield to 91%;12 however, lithium chloride was not as beneficial as we previously observed,10 and other additives did not generate better yields (entries 9–12). The screening of different loading of lithium bromide did not furnish a better result (entries 13–14).
Table 2 Optimization of reaction conditionsa,b

|
Entry |
Solvent |
Additive |
Temperature |
Reductant |
Yield (%) |
Reaction conditions: 1a (0.40 mmol), 2a (0.20 mmol), additive (0.20 mmol), reductant (0.60 mmol), L1d (0.02 mmol), NiBr2 (0.02 mmol), solvent (1 mL). Isolated yield. x = 7.5. x = 15. x = 20. Additive (0.15 mmol). Additive (0.30 mmol). |
1 |
NMP |
— |
80 °C |
Mn |
72 |
2 |
DMF |
— |
80 °C |
Mn |
40 |
3 |
DMA |
— |
80 °C |
Mn |
66 |
4 |
DMPU |
— |
80 °C |
Mn |
76 |
5c |
DMPU |
— |
80 °C |
Mn |
78 |
6d |
DMPU |
— |
80 °C |
Mn |
84 |
7e |
DMPU |
— |
80 °C |
Mn |
80 |
8 |
DMPU |
— |
80 °C |
Zn |
54 |
9 |
DMPU |
LiCl |
80 °C |
Mn |
61 |
10 |
DMPU |
LiBr |
80 °C |
Mn |
91 |
11 |
DMPU |
NaI |
80 °C |
Mn |
77 |
12 |
DMPU |
MgCl2 |
80 °C |
Mn |
26 |
13f |
DMPU |
LiBr |
80 °C |
Mn |
80 |
14g |
DMPU |
LiBr |
80 °C |
Mn |
90 |
Using the optimal reaction conditions, a range of substituted aryl bromides 1 were examined for the coupling reaction with 2a, furnishing a series of alkylated arenes 3 (Table 3). The challenging steric-hindered aryl bromide substrates 1b are reasonably tolerated, and the coupling product 3b was obtained in a slightly low yield. Additionally, aryl bromide with ester substituent at the meta-position of the benzene ring was obtained in moderate yield, providing the product 3c in the yield of 52%. Among the functional groups, the reactions were successfully observed in the presence of esters 3a–d, naphthyl 3e, aldehyde 3f, and ketones 3g, 3h. It should be noted that 3f is the intermediate product of 5-HT2A.4c The electron-rich substrate 3i could also be coupled with 2a in good yield. However, the reaction was not effective for aryl bromides bearing electron-rich reagents like 4-bromoanisole.
Table 3 Scope of aryl bromides in XECa,b
Reaction conditions: 1 (0.40 mmol), 2a (0.20 mmol), LiBr (0.20 mmol), Mn (0.60 mmol), L1d (0.03 mmol), NiBr2 (0.03 mmol), DMPU (1 mL). Isolated yield. |
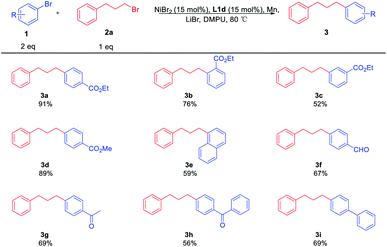 |
Then, the scope of the reaction with respect to primary alkyl bromides 2 was subsequently investigated. The results were shown in Table 4. All reactions provide the alkylated cross-coupling products smoothly. The functional groups, such as the ether 3j and benzyloxy 3h, all worked efficiently under the standard conditions. This catalytic method was also compatible with nitrogen-containing electrophiles, as demonstrated by the coupling of 2-(1-bromopropan-2-yl)isoindoline-1,3-dione 2l and N-(2-bromoethyl)phthalimide 2m with 1a in 61% and 62% yield, respectively. In the synthesis of the relatively longer-chain product 3n, it was found that the coupling product was obtained in satisfactory yield. Besides, a simple shorter-chain alkyl substrate 2o was converted to 3o smoothly. These results further showed that it did not significantly influence the reaction performance when changing the alkyl chains.
Table 4 Scope of alkyl bromides in XECa,b
Reaction conditions: 1a (0.40 mmol), 2 (0.20 mmol), LiBr (0.20 mmol), Mn (0.60 mmol), L1d (0.03 mmol), NiBr2 (0.03 mmol), DMPU (1 mL). Isolated yield. |
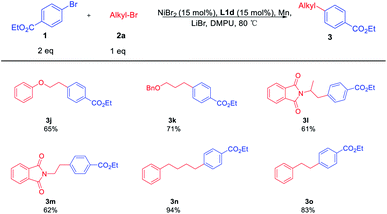 |
To further understand the mechanism of this reaction, the radical experiment was subsequently carried out (Scheme 3). Under the standard conditions, in the presence of the radical scavenger 2,2,6,6-tetramethyl-1-piperidinyloxy (TEMPO), the cross-coupling reaction was completely blocked and no alkylated arene 3a was recorded, which implies that this transformation might undergo a free radical pathway (Scheme 3a). To further demonstrate that the alkyl bromide might follow the radical pathway, a radical clock experiment was carried out.13,12d We used the cyclopropylmethyl bromide 2q as a coupling reagent under the standard conditions, and the cross-coupling product 3qa was not detected (Scheme 3b). The reaction resulted in a mixture of products; it was found that the reaction generates the ring-opened products 3qb, 3qc and 3qd in a total 51% yield, and the ratio of 3qb
:
3qd
:
3qe is about 2
:
0.4
:
2.4. These results further point towards the involvement of alkyl radical intermediate generation in the coupling process of alkyl bromides.
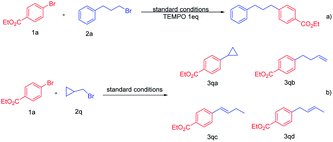 |
| Scheme 3 Mechanistic studies. | |
The current mechanistic hypothesis is outlined in Scheme 4. The reaction begins with the initial reduction of the NiII precatalyst, which can furnish a catalytically active Ni0Ln species. Subsequent oxidative addition to the aryl bromide forms an aryl-NiII intermediate. Then, it reacts with an alkyl radical to give an aryl alkyl NiIII intermediate. Reductive elimination occurs to give the aryl–alkyl cross-coupling product along with generating the NiI species. The radical generation gives the NiII intermediate, which is subjected to reduction by manganese and regenerates the Ni0Ln species to complete the catalytic cycle.
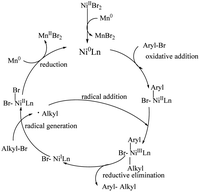 |
| Scheme 4 The plausible mechanism. | |
Conclusions
In conclusion, by using a NiBr2/L1d catalyst, the cross-electrophile coupling reactions of aryl bromides and primary alkyl bromides were developed. A variety of alkylated arene products with various functional groups could be obtained in moderate to excellent yields. The result further demonstrated that the nickel/spiro-bidentate-pyox catalytic cross-electrophile coupling protocol afforded an effective method for the synthesis of alkylated arene products. Further investigation to extend this catalytic protocol to other coupling reactions and a detailed mechanistic study are ongoing and will be reported in due course.
Conflicts of interest
There are no conflicts to declare.
Acknowledgements
The authors thank professor Longjiang Huang for the MS analysis and Dr Bo Sun for the help in experimental discussion.
Notes and references
-
(a) X. Qian, A. Auffrant, A. Felouat and C. Gosmini, Angew. Chem., Int. Ed., 2011, 50, 10402 CrossRef CAS PubMed
;
(b) A. Krasovskiy, C. Duplais and B. H. Lipshutz, Org. Lett., 2010, 12, 4742 CrossRef CAS PubMed
;
(c) X. L. Yu, T. Yang, S. L. Wang, H. L. Xu and H. G. Gong, Org. Lett., 2011, 13, 2138 CrossRef CAS PubMed
;
(d) D. A. Everson, R. Shrestha and D. J. Weix, J. Am. Chem. Soc., 2010, 132, 920 CrossRef CAS PubMed
;
(e) M. R. Prinsell, D. A. Everson and D. J. Weix, Chem. Commun., 2010, 46, 5743 RSC
;
(f) R. Jana, T. P. Pathak and M. S. Sigman, Chem. Rev., 2011, 111, 1417 CrossRef CAS PubMed
;
(g) L. Ackermann, J. Org. Chem., 2014, 79, 8948 CrossRef CAS PubMed
;
(h) Q. Cao, J. L. Howard, E. Wheatley and D. L. Browne, Angew. Chem., Int. Ed., 2018, 57, 11339 CrossRef CAS PubMed
;
(i) S. Biswas, B. Qu, J. N. Desrosiers, Y. Choi, N. Haddad, N. K. Yee, J. J. Song and C. H. Senanayake, J. Org. Chem., 2020, 85, 8214 CrossRef CAS PubMed
;
(j) M. Amatore and C. Gosmini, Chem.–Eur. J., 2010, 16, 5848 CrossRef CAS PubMed
;
(k) R. J. Perkins, D. J. Pedro and E. C. Hansen, Org. Lett., 2017, 19, 3755 CrossRef CAS PubMed
;
(l) J. A. Milligan, J. P. Phelan, S. O. Badir and G. A. Molander, Angew. Chem., Int. Ed., 2019, 58, 6152 CrossRef CAS PubMed
;
(m) K. E. Poremba, S. E. Dibrell and S. E. Reisman, ACS Catal., 2020, 10, 8237 CrossRef CAS PubMed
. -
(a) S. S. Wu, W. J. Shi and G. Zou, New J. Chem., 2021, 45, 11269 RSC
;
(b) D. A. Everson, B. A. Jones and D. J. Weix, J. Am. Chem. Soc., 2012, 134, 6146 CrossRef CAS PubMed
;
(c) K. A. Johnson, S. Biswas and D. J. Weix, Chem.–Eur. J., 2016, 22, 7399 CrossRef CAS PubMed
;
(d) P. Zhang, C. C. Le and D. W. C. MacMillan, J. Am. Chem. Soc., 2016, 138, 8084 CrossRef CAS PubMed
;
(e) A. Paul, M. D. Smith and A. K. Vannucci, J. Org. Chem., 2017, 82, 1996 CrossRef CAS PubMed
;
(f) Y. X. Jin and C. Wang, Angew. Chem., Int. Ed., 2019, 58, 6722 CrossRef CAS PubMed
;
(g) Y. X. Jin, H. Yang and C. Wang, Org. Lett., 2020, 22, 2724 CrossRef CAS PubMed
;
(h) Y. Lan and C. Wang, Commun. Chem., 2020, 3, 1 CrossRef
;
(i) D. A. Everson and D. J. Weix, J. Org. Chem., 2014, 79, 4793 CrossRef CAS PubMed
;
(j) D. J. Weix, Acc. Chem. Res., 2015, 48, 1767 CrossRef CAS PubMed
;
(k) X. Wang, Y. J. Dai and H. G. Gong, Top. Curr. Chem., 2016, 374, 43 CrossRef PubMed
;
(l) E. Richmond and J. Moran, Synthesis, 2018, 50, 499 CrossRef CAS
;
(m) N. T. Kadunce and S. E. Reisman, J. Am. Chem. Soc., 2015, 137, 10480 CrossRef CAS PubMed
;
(n) J. Sheng, H. Q. Ni, H. R. Zhang, K. F. Zhang, Y. N. Wang and X. S. Wang, Angew. Chem., Int. Ed., 2018, 57, 7634 CrossRef CAS PubMed
. -
(a) A. H. Cherney, N. T. Kadunce and S. E. Reisman, J. Am. Chem. Soc., 2013, 135, 7442 CrossRef CAS PubMed
;
(b) Y. Zhao and D. J. Weix, J. Am. Chem. Soc., 2014, 136, 48 CrossRef CAS PubMed
;
(c) C. L. Zhao, X. Jia, X. Wang and H. G. Gong, J. Am. Chem. Soc., 2014, 136, 17645 CrossRef CAS PubMed
;
(d) M. Parasram, B. J. Shields, O. Ahmad, T. Knauber and A. G. Doyle, ACS Catal., 2020, 10, 5821 CrossRef CAS PubMed
;
(e) A. C. Wotal and D. J. Weix, Org. Lett., 2012, 14, 1476 CrossRef CAS PubMed
;
(f) G. A. Molander, S. R. Wisniewski and K. M. Traister, Org. Lett., 2014, 16, 3692 CrossRef CAS PubMed
;
(g) S. Y. Kim, T. Iwai, S. Fujii, K. Ueno and M. Sawamura, Chem.–Eur. J., 2021, 27, 2289 CrossRef PubMed
;
(h) S. Y. Kim, M. J. Goldfogel, M. M. Gilbert and D. J. Weix, J. Am. Chem. Soc., 2020, 142, 9902 CrossRef CAS PubMed
;
(i) J. W. Wang, J. H. Zhao and H. G. Gong, Chem. Commun., 2017, 53, 10180 RSC
;
(j) E. J. Barreiro, A. E. Kümmerle and C. A. M. Fraga, Chem. Rev., 2011, 111, 5215 CrossRef CAS PubMed
. -
(a) D. Hoyer, D. E. Clarkena and P. P. A. Humphrey, Pharmacol. Rev., 1994, 46, 157 CAS
;
(b) I. V. Wijngaarden and W. Soudijn, 5-HT2A, 5-HT2B and 5-HT2C Receptor Ligands, in Pharmacochemistry Library, ed. B. Oliver, I. V. Wijngaarden and W. Soudijn, Elsevier, 1997, vol. 27, pp. 161–197 Search PubMed
;
(c) C. S. Dowd, K. Herrick-Davis, C. Egan, A. DuPre, C. Smith, M. Teitler and R. A. Glennon, J. Med. Chem., 2000, 43, 3074 CrossRef CAS PubMed
. - J. Q. Li, J. Feng, W. P. Cai, Z. X. Zhang and T. Yin, WO 2010125908 A1, 2010
. - A. Sasse, K. Kiec-Kononowicz, H. Stark, M. Motyl, S. Reidemeister, C. R. Ganellin, X. Ligneau, J.-C. Schwartz and W. Schunack, J. Med. Chem., 1999, 42, 593 CrossRef CAS PubMed
. -
(a) L. Y. Lv, Z. H. Qiu, J. B. Li, M. X. Liu and C. J. Li, Nat. Commun., 2018, 9, 4739 CrossRef PubMed
;
(b) M. L. Zhang, R. Ruzi, J. W. Xi, N. Li, Z. K. Wu, W. P. Li, S. Y. Yu and C. J. Zhu, Org. Lett., 2017, 19, 3430 CrossRef CAS PubMed
. - D. J. Charboneau, E. L. Barth, N. Hazari, M. R. Uehling and S. L. Zultanski, ACS Catal., 2020, 10, 12642 CrossRef CAS PubMed
. - R. J. Perkins, A. J. Hughes, D. J. Weix and E. C. Hansen, Org. Process Res. Dev., 2019, 23, 1746 CrossRef CAS
. - N. X. Gao, Y. S. Li, G. R. Cao and D. W. Teng, New J. Chem., 2021, 45, 16477 RSC
. - L. L. Anka-Lufford, K. M. M. Huihui, N. J. Gower and D. J. Weix, Chem.–Eur. J., 2016, 22, 11564 CrossRef CAS PubMed
. -
(a) Y. Q. Li, Y. H. Fan and Q. F. Jia, Chin. J. Org. Chem., 2019, 39, 350 CrossRef CAS
;
(b) L. Huang, A. M. Olivares and D. J. Weix, Angew. Chem., Int. Ed., 2017, 56, 11901 Search PubMed;
(c) W. J. Scott and J. K. Stille, J. Am. Chem. Soc., 1986, 108, 3033 CrossRef CAS
;
(d) Y. Sumida, T. Sumida and T. Hosoya, Synthesis, 2017, 49, 3590–3601 CrossRef CAS
;
(e) T. Kamikawa and T. Hayashi, Tetrahedron Lett., 1997, 38, 7087 CrossRef CAS
. - T. Z. Lin, Y. Y. Gu, P. C. Qian, H. X. Guan, P. J. Walsh and J. Y. Mao, Nat. Commun., 2020, 11, 5638 CrossRef CAS PubMed
.
Footnote |
† Electronic supplementary information (ESI) available. See DOI: 10.1039/d2ra00010e |
|
This journal is © The Royal Society of Chemistry 2022 |
Click here to see how this site uses Cookies. View our privacy policy here.