DOI:
10.1039/D1RA09440H
(Paper)
RSC Adv., 2022,
12, 7237-7252
Physiological and oxidative stress responses of Solanum lycopersicum (L.) (tomato) when exposed to different chemical pesticides†
Received
30th December 2021
, Accepted 1st February 2022
First published on 2nd March 2022
Abstract
Pesticide overuse can have negative effects on developmental processes of non-target host plants. By increasing reactive oxygen species (ROS) levels, pesticides negatively affect cellular metabolism, biochemistry and physiological machinery of plants. Considering these problems, the current study was planned to assess the effect of three different groups of pesticides, namely diazinon (DIZN), imidacloprid (IMID) and mancozeb (MNZB) on Solanum lycopersicum L. (tomato). In general, pesticides resulted in a progressive decrease in physiological and biometric parameters of S. lycopersicum (L.), which varies significantly among concentrations and species of pesticides. Among them, 200 μgMNZB mL−1 had the most severe negative impact and reduced germination rate, root biomass, chl a, chl b, total chlorophyll and carotenoids by 62, 87, 90, 88, 92 and 90%, respectively. In addition, higher doses of pesticides greatly reduced the flowering, fruit attributes and lycopene content. Furthermore, plants exposed to 200 μgDIZN mL−1 showed a progressive drop in root cell viability (54% decrease), total soluble sugar (TSS) (64% decrease) and total soluble protein (TSP) (67% decrease) content. Data analysis indicated that greater doses of pesticides dramatically raised ROS levels and induced membrane damage through production of thiobarbituric acid reactive substances (TBARS), as well as increased cell injury. To deal with pesticide-induced oxidative stress, plants subjected to greater pesticide dosages, showed a substantial increase in antioxidant levels. For instance, ascorbate peroxidase (APX), catalase (CAT), superoxide dismutase (SOD), peroxidase (POD) and guaiacol peroxidase (GPX) were maximally increased by 48, 93, 71, 52 and 94%, respectively following 200 μgMNZB mL−1 soil exposures. Additionally, under a confocal laser scanning microscope (CLSM), pesticide exposed S. lycopersicum (L.) roots stained with 2′,7′-dichlorodihydrofluorescein diacetate (2′7′-DCF) and 3,3′-diaminobenzidine, exhibited an increased ROS production in a concentration-dependent manner. Further, elevated pesticide concentrations resulted in alterations in mitochondrial membrane potential (ΔΨm) and cellular death in roots, as evidenced by increased Rhodamine 123 (Rhd 123) and Evan's blue fluorescence, respectively. These findings clearly showed that applying pesticides in excess of permissible amounts might induce oxidative stress and cause oxidative damage in non-target host plants. Overall, the current study indicates that a thorough and secure method be used before selecting pesticides for increasing production of agronomically important vegetable crops in various agro-climatic zones.
1. Introduction
Chemical pesticides have become a significant feature in contemporary agriculture for minimizing crop losses and improving food output.1 The usage of these pesticides is thought to be important in vegetable cropping systems to avoid disease and insect damage. Despite the fact that fungicides are routinely employed throughout plant growth to promote crop quantity and quality, they might be a major source of environmental pollution, posing public health risks.2 Pesticides have an effect that is not restricted to the pathogenic organisms that are being targeted. They also significantly harm the developmental and reproductive systems of non-target species such as plants. Fungicide overuse causes cellular oxidative damage in plants.3 Several studies have found that pesticide toxicity diminishes the photosynthetic pigments,4 increases reactive oxygen species (ROS),5 modifies antioxidant and detoxifying enzyme activity,6 and increases critical genes involved in the breakdown of pesticides in non-target plants.7
Apart from these, several chemical pesticide has been shown to have a variety of negative effects in various agriculturally important crop plant by-(i) reducing the pollen performance8 (ii) impairing the reproductive processes9 (iii) disrupting the growth and yield10 (iv) retarding the germination rate and11 disturbing the morphological and physiological parameters.12 The stresses induced by pesticides are reported to cause oxidative damage, often contributing the toxicity as reactive oxygen species (ROS) including H2O2 (hydrogen peroxide), O2 (superoxide) and OH˙ (hydroxyl radical).13 The chemical species created when oxygen is incompletely reduced is referred to as reactive oxygen species (ROS).14 The ROS molecules are very hazardous and may oxidize the majority of proteins, lipids, and nucleic acids, resulting in cell death owing to lipid peroxidation, membrane degradation, and enzyme deactivation.15 According to reports, increasing the ratios of nicotinamide adenine dinucleotide (NAD) and nicotinamide adenine dinucleotide phosphate (NADP) interferes with electron transport system (ETS) and raises adenosine triphosphate (ATP) levels by causing changes in enzyme systems, resulting in conservation of physiological machinery of plants. Furthermore, pesticides administration has been shown to affect the effective quantum yield of PS-II (PS-II) and maximum quantum efficiency of PS-II (Fv/Fm) of plants.16
Tomato (Solanum lycopersicum L.) is a commonly farmed vegetable that has evolved into a model species for genetic research on fruit quality, stress tolerance, and disease resistance.17 It is a prominent horticultural crop with a global output estimate of more than 120 million metric tonnes18 and a high economic value across the world. Globally, tomato consumption is steadily increasing as a result of the world's fast population growth. Tomatoes are eaten in a number of ways, like as a vegetable, salad, fruit, ketchup, and chatni, among others.19 Despite the widespread use of pesticides, various fungal infections continue to cause major output losses in tomatoes across the world.20 Among them, Alternaria solani (causing early blight disease), Fusarium oxysporum (fusarial wilt disease) and Phytophthora infestans (causing late blight disease) are major phytopathogens causing huge losses in tomato yield.21 Since, tomato crop are susceptible to significant fungal infections, a number of chemical pesticides including imidacloprid, hexaconazole, kitazin, mancozeb, diazinon, maneb and thiram etc. have been widely utilized in agricultural systems to control the fungus since 1931.22 These chemical pesticides act by killing fungal cells after the mycelium has entered in to the parenchymatous tissues, and thus, stop the dispersion or infection inside the plant organs.23 However, indiscriminate and irregular application of these chemical pesticides often causes adverse impact on soil properties as well as growth and yield of edible crops including tomatoes. In this regard, several workers have reported the harmful effect of pesticides on physiology and biochemical features of S. lycopersicum (L.). For instance, a crop-based research conducted by Shakir et al.24 showed that different group of chemical pesticides (imidacloprid, alpha cypermethrin, benzoate and emamectin) negatively affected the various biometric parameters of S. lycopersicum (L.).
The current study, which used S. lycopersicum (L.) as a test crop, was designed to address the important concerns connected with the administration of pesticides in vegetable production. The main objectives of the current finding was to-(i) assessing the biotoxic effect of chemical pesticides; diazinon, imidacloprid and mancozeb on germination efficiency, bio-chemical features (length and dry biomass) and leaf pigments of S. lycopersicum (L.) (ii) evaluating the percent phytotoxicity, survival percentage and tolerance indices of S. lycopersicum (L.) cultivated in the presence of pesticides (iii) estimating the flower parameter, fruit attributes and lycopene content in S. lycopersicum (L.) exposed to varying concentrations of pesticides (iv) determining the total soluble protein, soluble sugar and cell viability of S. lycopersicum (L.) roots treated with pesticides (v) assessing the responses of pesticides on stress biomarkers, oxidative stress and antioxidant defense enzymes (SOD, POD, CAT, APX and GPX) and (vi) evaluating the pesticide induced ROS generation, cellular death and impairment in mitochondrial membrane potential (ΔΨm) of S. lycopersicum (L.) root.
2. Materials and methods
2.1 Pesticide treatment and growth conditions
Healthy and uniform-sized seeds of S. lycopersicum L. (tomato) were procured from local market. Surface sterilization of seeds was done by dipping the seeds in 70% ethanol solution (for 3 min) and 4% sodium hypochlorite (NaOCl) (Hi-Media, Mumbai, India) solution (for 3 min), six times rinsed with six separate sterile water changes, and then dried at room temp. In earthen pots containing 5.0 kg unsterilized alluvial sandy clay loam soils, properly sterilized seeds (08 seeds per pot) were sowed. In double distilled water (DDW), the tested pesticides; diazinon (DIZN), imidacloprid (IMID) and mancozeb (MNZB) (Table 1) for application were made. Each experiment was carried out in triplicate (n = 5), with all treated and untreated pots set up in a totally randomized block pattern. Then, 15 days after emergence, pots were carefully trimmed, and three plants were preserved in each pot. The experimental pots were kept in an open field setting and watered with tap water on a daily basis. The entire study was carried out over two years, and each trial was repeated over a two-year period with the same or similar treatment to evaluate data repeatability and accuracy.
Table 1 Pesticides used
Physico-chemical properties |
Pesticides |
Diazinon |
Imidacloprid |
Mancozeb |
Common name |
Diazinon |
Imidacloprid |
Mancozeb |
Chemical formula |
C12H21N2O3PS |
C9H10ClN5O2 |
C4H6N2S4Mn·C4H6N2S4Zn |
Systematic IUPAC name |
O,O-Diethyl-O-[4-methyl-6-(propan-2-yl)pyrimidin-2-yl]phosphorothioate |
N-{1-[(6-Chloro-3-pyridyl)methyl]-4,5-dihydroimidazol-2-yl} nitroamide |
Manganese ethylenebis(dithiocarbamate) (polymeric) complex with zinc salt |
Chemical family |
Organophosphate |
Chloronicotinyl |
Dithiocarbamate |
Grade |
Commercial |
Commercial |
Commercial |
CAS no. |
333-41-5 |
138261-41-3 |
8018-01-7 |
![[thin space (1/6-em)]](https://www.rsc.org/images/entities/char_2009.gif) |
Recommended dose |
Appearance |
Colorless to dark brown liquid |
White, milky |
Grayish-yellow powder |
Molecular weight |
304.34 |
255.661 g mol−1 |
271.3 |
Solubility |
H2O |
H2O |
H2O |
Source |
Prijat Agrochemicals |
Prijat Agrochemicals |
Prijat Agrochemicals |
Chemical structure |
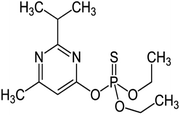 |
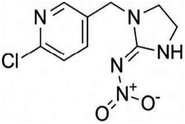 |
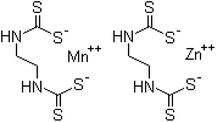 |
2.2 Effect of pesticides on germination efficiency and seedling vigor index
S. lycopersicum (L.) seeds were surface sterilized for three minutes with a solution of 3 percent (w/v) NaOCl (HiMedia, Mumbai, India), and then washed three times with DDW. Radicle emergence of one millimetre (mm) was deemed germinated seeds five days after sowing (DAS), and the germination percentage was measured as:
The seedling vigor index was calculated using the percentage of germination.25
Seedling vigor index (SVI) = [root length + shoot length] × %seed germination |
2.3 Toxic effect of pesticides on biological (length and dry biomass) and physiological parameters (leaf pigments) of S. lycopersicum
S. lycopersicum (L.) plants raised in earthen pots added with varying rates of tested pesticides were uprooted, properly cleaned, oven-dried in a ventilated oven (Yorco, York Scientific Industries, Pvt. Ltd India), and the dry matter was calculated. To determine the photosynthetic pigments accumulated in pesticides untreated/treated fresh foliage of S. lycopersicum, the method of Arnon26 was used. By macerating fresh leaves in an 80% acetone (Sisco Research Laboratory Pvt. Ltd, Mumbai, India) concentration, the pigments were extracted (see ESI section†).
2.4 Effect of pesticides on flowering and fruit attributes
At 70 DAS, the number of blossoms per plant was counted, and the quantity of fruits produced by each plant was tallied at various intervals. The average number of fruits per plant was calculated by combining the number of fruits produced on each plant and collected at different growth periods. At harvest, quantity of fruits/branch, fruit ripening, fruit diameter, fresh/dry biomass, and moisture content of the fruits were recorded.
2.5 Percent phytotoxicity, survival and tolerance indices and root: shoot length ratio of S. lycopersicum
At harvest, the phytotoxic effect tested pesticides were evaluated by means of percent phytotoxicity, tolerance and survival indices and root and shoot length ratio. The percentage of phytotoxicity was calculated according to Chou and Lin27 as:
Tolerance index (TI) was determined by the formula used by Iqbal and Rahmati.28
2.6 Determination of total soluble protein (TSP)
Bradford's method29 for determining total soluble protein (TSP) concentration included utilizing Coomassie Brilliant Blue G-250 (Sigma-Aldrich) as a dye and albumin (BSA; HiMedia, Mumbai, India) as a standard. Furthermore, for protein measurement, the Bradford reagent (a combination of 13.3 g Cu-acetate, 1.8 mL acetic acid; CH3COOH and 200 mL DDW) was utilized. Fresh leaves (100 mg) were homogenized in a 1.0 mL phosphate buffer (pH = 7.0) using a mortar and pestle. For a period of 15 min, the crude homogenate was centrifuged (at 4000 rpm). The enzyme reaction mixture consisted of DW (2.0 mL), enzyme extract (20 μL) and Bradford reagent (0.5 mL). The absorbance at 595 nm was measured (UV-visible spectrophotometer UV-2450, Shimadzu, Tokyo, Japan) using distilled water as a blank and BSA (HiMedia, Mumbai, India) as a reference. The following calculation was used to calculate total protein content:
Total soluble protein (mg g−1 FW) = C × V/Vt × W |
C = linear regression equation is used to get the absorbance coefficient (X = OD − 0.592/0.033), V = volume of phosphate buffer, Vt = volume of enzyme extract, W = plant weight.
2.7 Total soluble sugars (TSS)
The total soluble sugar concentration (TSS) was determined using a slightly modified Shields and Burnett30 technique. With the aid of a mortar and pestle, fresh and fragile plant organs (50 mg) were homogenized in liquid N and pulverized to powder. After that, add 3.0 mL of 90% ethanol and incubate for 1 hour at 60–70 °C. In a volumetric flask, the extract was combined with 90% ethanol to make a final volume of 25 mL. A 1.0 mL aliquot was combined with 1.0 mL phenol (5%) and 5.0 mL of sulphuric acid (H2SO4; Sisco Research Laboratory Pvt. Ltd, Mumbai, India). The content of TSS was calculated as mg g−1 FW by taking the OD at 485 nm using glucose as reference.
2.8 Determination of lycopene content
Tomato fruits were detached from S. lycopersicum (L.) plants (at harvest) grown in pot soils treated with different (0–200 μg mL−1) concentrations of each tested pesticides. Each fruit's pulp was sliced from under the surface, and a total of 1.0 g of sample was crushed in 2 mL DDW using a mortar and pestle. For 1 hour, the tubes holding the materials were vortexed in a water bath at 30 °C. Then, 8.0 mL of a hexane
:
acetone
:
ethanol (2
:
1
:
1) combination was added to each tube. The samples were vortexed right away and incubated for 10 minutes at room temperature. Following that, 1 mL DDW was added to each tube and vortexed. Samples were allowed to stand for 10 minutes to separate stages. The absorbance was then measured at 503 nm (UV-2450, Shimadzu, Tokyo, Japan).
2.9 Proline determination
Free proline accumulated in phyto-organs of pesticides treated S. lycopersicum plants was extracted by homogenization in 3% (w/v) sulphosalicylic acid, and measured using aninhydrin-based colorimetric test as described by Bates et al.31 Using a mortar and pestle, 500 mg of fresh samples were homogenized in 5.0 mL of 3% sulphosalycylic acid, then, centrifuged at 4000 rpm for 30 min (Remi RM-12C Micro). The 1.0 mL acid ninhydrin (prepared in 1.25 g of ninhydrin in 30 mL glacial acetic acid and 20 mL 6 M phosphoric acid) and 1.0 mL glacial acetic acid (HiMedia, Mumbai, India) were mixed along with the 1.0 mL supernatant and the mixture was maintained in the oven for an hour at 100 °C. After finishing the reaction in an ice bath, 2.0 mL toluene was added and the mixture was maintained at room conditions until two layers separated. The absorbance of the higher colored layers was measured at 520 nm using UV-visible spectrophotometer (UV-2450, Shimadzu, Tokyo, Japan). Using a previously established standard curve, the concentration of free proline was estimated.
2.10 Determination of thiobarbituric acid reactive substance (TBARSs)
Using the technique of Hodges et al.32 with minor modifications, in terms of thiobarbituric acid reactive chemicals (TBARSs), the amount of lipid peroxidation products was determined. Using a mortar and pestle, freshly detached pesticides untreated/treated roots and shoots samples weighing 200 mg were crushed in 3 mL of 0.25 percent (w/v) 2-thiobarbituric acid (TBA; HiMedia, Mumbai, India) in 10% tri-chloroacetic acid (TCA; HiMedia, Mumbai, India). Homogenate was heated to 95 °C for 30 minutes, then, promptly cooled in an ice bath before being centrifuged for 10 minutes at 10
000×g. Nonspecific turbidity was calculated by subtracting the absorbance at 600 nm from the absorbance of supernatant at 532 nm. The amount of lipid peroxidation was calculated using TBARS.
2.11 Cellular injury analysis
To determine cell damage, membrane permeability in terms of electrolyte leakage was evaluated in pesticide treated fresh root and shoot tissues of S. lycopersicum using an electrical conductivity meter as previously described by Lu et al.33 Fresh leaf and root portions of plants were placed in a boiling tube with 20 mL of DDW and incubated overnight at 10 °C to determine the amount. Freshly detached samples i.e. roots and leaf (EC-I) and autoclaved (for 15 minutes at 120 °C) plant samples (EC-II) had their electrical conductivity measured. Each sample's cell damage was determined using a formula:
Cell injury = EC − I/EC − II × 100 |
2.12 Analysis of cell viability
The triphenyl tetrazolium chloride (TTC; Sigma-Aldrich) reduction procedure was employed to measure cell viability. Fresh leaves and roots (0.2 g) were cultured for 24 hours at 32 °C in 0.6% (v/v) TTC, phosphate buffer. Before extracting samples, they were washed in distilled water with 95% ethanol for 4 hours at 65 °C. The cell viability was estimated by measuring the absorbance at 490 nm and multiplying it by the absorbance/g of fresh weight.
2.13 Antioxidant enzymes determination in foliage of pesticide treated S. lycopersicum (L.)
A-0.5 g freshly detached aerial parts (shoot samples) of pesticides treated S. lycopersicum (L.) were homogenized in 50 mM phosphate buffer having a pH value of 7.8 under cold conditions after being pulverized with a mortar and pestle. The homogenized mixture was centrifuged at 12
000×g for 10 minutes at 4 °C after being filtered through four layers of muslin cloth. These prepared samples were used for the analysis of different antioxidant enzymes viz., ascorbate peroxidase (APX; 1.11.1.11), guaiacol peroxidase (GPX, EC 1.11.1.7), catalase (CAT; 1.11.1.6), superoxide dismutase (SOD; 1.15.1.1) and glutathione reductase (GR; 1.6.4.2) (refer to ESI† section for detailed descriptions).
2.14 Assessment of mitochondrial membrane potential, ROS generation and cell death in root tissues of pesticide treated S. lycopersicum
To assess the pesticidal damages (in the form of ROS production, impairment in mitochondrial membrane potential and cell death), the seedlings of S. lycopersicum were cultivated for seven days on soft agar plates supplemented with 0–200 μg mL−1 doses of DIZN, IMID and MNZB. For ROS generation, the root samples were stained with 3,3′-diaminobenzidine (DAB; Sigma-Aldrich) and 0.25 μM 2′,7′-dichloro–dihydro–fluorescein diacetate (DCFH-DA; Sigma-Aldrich) for 15–20 minutes and placed on a glass slide after being washed with PBS for several times. Under CLSM (LSM-780, Leica Confocal microscope, Zeiss, Oberkochen, Germany), dye stained root samples were detected for increasing dead tissues as pesticide doses steadily increased.
Furthermore, in order to assess the pesticide induced visual changes in mitochondrial membrane potential of S. lycopersicum (L.) roots, pesticide treated roots of plant seedling were stained with 1.0 g mL−1 of Rhodamine 123 (Rh123; Sigma-Aldrich) for 30 minutes in the dark, and the photos were captured using a fluorescence microscope (CLSM).34
The Evans blue staining technique, as reported by Shahid et al.11 was used to measure cell viability loss. Evans blue (0.25% w/v; Sigma-Aldrich) dye was applied to pesticide-treated roots for 15 minutes before being rinsed with DDW (for 30 minutes). The CLSM was used to evaluate the samples in order to assess cell death. The fluorescence intensity of the root tip, meristematic zone, and elongation zone was quantified using the Leica application suite (LASAF lite 2.6.0) software. As a function of pesticide concentration on five roots (n = 5) per treatment, the intensity counts were averaged and displayed as mean S.D. LASAF lite's inbuilt histogram tool was used to measure three channels for a single region of interest (ROI), as well as the average intensity curves for three distinct root zones were displayed.
2.15 Statistical analysis
All experimental trials were carried out in a randomized fashion, with five replicates (n = 5) of each unique treatment. The data for S. lycopersicum plants were combined, and the mean separation among columns was calculated. The means and standard deviation (SD) of three replicates (n = 5) of each treatment are reported in all data. At p ≤ 0.05 significant level, different letters indicate a significant difference in treatment. In addition, using the Minitab 17 statistical tool, the least significant differences (LSD) between treatment means was computed using two-way analysis of variance (ANOVA) at p ≤ 0.05.
3. Results and discussion
3.1 Pesticides hindered the seed germination efficiency and vigor indices of S. lycopersicum (L.)
Germination is an important process in the life of every plant. The uttermost and most crucial property of seeds is seedling germination and vigor index. As a result, the most important physiognomies of the seeds to be employed for cultivation are seed germination and seedling vigor index. Seeds that germinate quickly and aggressively in controlled circumstances are likely to generate robust seedlings in the fields as well. Here, germination efficiency of S. lycopersicum (L.) seeds planted in soils treated with varied dosages of pesticides varies significantly. Seeds planted under regulated (untreated) circumstances germinated to a maximally. Higher doses of test pesticides had a maximum toxic effect, where, germination rate was decreased by 60, 58 and 62% when soils exposed to 200 μg mL−1 each of diazinon (DIZN), imidacloprid (IMID) and mancozeb (MNZB), respectively over untreated control (Fig. 1A). In this regard, it has been discovered that delayed germination after pesticide spraying is associated to a disturbed germination metabolism. Similar to our study, increasing concentration of chemical fungicide kitazin negatively affected the germination efficiency of P. sativum both in in vitro and in vivo conditions.11 In another study, Rajashekar and Murthy35 reported that as the concentration of pendimethalin was increased, the percentage of germination in Zea mays (L.) reduced dramatically.
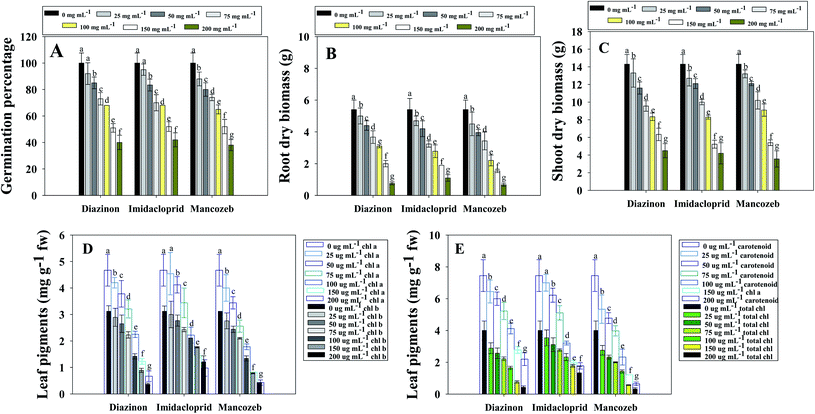 |
| Fig. 1 Effect of different concentrations of test pesticides on; germination rate (A), root dry biomass (B), shoot dry biomass (C) leaf pigments; chlorophyll a and b (D) and total chlorophyll and carotenoid content (E) of Solanum lycoperscium plants grown in sandy clay loam soils treated with different dosage of diazinon, imidacloprid and mancozeb. In this figure, bar diagrams represents the mean values of five replicates (n = 5). Corresponding error bars represents standard deviation (SD) of five replicates (SD, n = 5). | |
3.2 Pesticides negatively affect the biological and physiological features of S. lycopersicum (L.)
3.2.1 Length, dry biomass and leaf pigments. The analysis of plant growth is an important step in understanding the performance and productivity because it highlights the various methods that plants use to thrive in environments where certain characteristics are restricting. Under pesticide stress, S. lycopersicum (L.) showed a varied level of biological response. The lower concentrations of pesticides showed lesser toxic effect which, however, increased with increasing doses (25–200 μg mL−1). As an example, root length of S. lycopersicum was greatly reduced by 68, 46 and 71% when plants were cultivated in the presence of 200 μg mL−1 of each DIZN, IMID and MNZB, respectively, compared with control (Table 2). While comparing the effect of all tested pesticides, the higher doses of mancozeb had most deadly influence on growth features of tomatoes and maximally reduced (55%) the shoot length (Table 2). Mishra et al.36 observed that high concentrations of the pesticide dimethoate inhibited root and shoot growth of Vigna radiata. The tested chemical pesticides may cause various disruptions in some processes, including as nitrogen metabolism and photosynthesis, resulting in a reduction in plant development.
Table 2 Effect of varying tested concentrations of pesticides on biometric parameters (length and fresh weight), total soluble protein (TSP), total soluble sugar (TSS) and lycopene content in S. lycopersicum (L.) plants grown in pot soila
Pesticides |
Concentrations used (μg mL−1) |
Biological features |
Total soluble protein (mg g−1) |
Total soluble sugar (mg g−1 FW) |
Lycopene (mg kg−1 FW) |
Length/plants (cm) |
Fresh weight/plants (g) |
Roots |
Shoots |
Roots |
Shoots |
Values are mean of three independent replicates. ± indicates standard deviation. |
Control |
0 |
36.7 ± 4.6 (a) |
57.8 ± 6.8 (a) |
16.4 ± 5.0 (a) |
25.6 ± 7.2 (a) |
28.4 ± 4.6 (a) |
123 ± 21 (a) |
12.0 ± 2.3 (a) |
Diazinon |
25 |
34.2 ± 2.3 (a) |
56.0 ± 8.9 (a) |
15.2 ± 5.3 (a) |
25.3 ± 6.7 (a) |
27.0 ± 3.8 (a) |
119 ± 6.7 (b) |
10.3 ± 0.9 (b) |
50 |
31.5 ± 4.1 (b) |
53.4 ± 4.6 (b) |
13.2 ± 2.8 (b) |
22.4 ± 4.4 (b) |
24.8 ± 2.7 (b) |
113 ± 9.8 (c) |
9.6 ± 0.4 (c) |
75 |
27.2 ± 2.1 (c) |
49.4 ± 4.1 (b) |
11.6 ± 4.0 (c) |
19.3 ± 3.7 (b) |
22.0 ± 3.5 (b) |
105 ± 5.6 (d) |
9.0 ± 1.0 (c) |
100 |
22.0 ± 1.8 (d) |
42.7 ± 3.6 (d) |
9.3 ± 00 (d) |
17.3 ± 3.6 (c) |
19.3 ± 2.2 (c) |
97.4 ± 7.7 (e) |
8.3 ± 2.1 (d) |
150 |
17.6 ± 0.8 (f) |
38.6 ± 2.3 (e) |
6.4 ± 2.1 (e) |
13.4 ± 2.2 (d) |
16.4 ± 4.2 (d) |
92.3 ± 4.8 (f) |
7.0 ± 0.4 (e) |
200 |
11.8 ± 1.1 (g) |
32.0 ± 3.9 (f) |
4.1 ± 0.7 (f) |
9.4 ± 1.8 (e) |
13.2 ± 1.7 |
86.2 ± 7.1 (g) |
7.3 ± 0.0 (e) |
Mean |
24.05 |
45.35 |
9.96 |
17.8 |
20.4 |
102 |
9.08 |
Imidacloprid |
25 |
35.8 ± 5.5 (a) |
55.8 ± 4.9 (a) |
14.2 ± 2.4 (b) |
24.4 ± 6.3 (a) |
28.0 ± 7.2 (a) |
121 ± 9.6 (a) |
11.5 ± 1.2 (a) |
50 |
33.2 ± 4.7 (b) |
51.0 ± 7.8 (b) |
11.3 ± 4.3 (c) |
21.5 ± 5.2 (b) |
27.0 ± 5.4 (a) |
118 ± 11 (b) |
10.0 ± 0.6 (b) |
75 |
32.0 ± 3.3 (b) |
47.3 ± 6.6 (c) |
9.2 ± 1.3 (d) |
17.2 ± 3.4 (c) |
24.3 ± 2.3 (b) |
115 ± 8.8 (c) |
9.7 ± 0.87 (c) |
100 |
29.7 ± 2.6 (c) |
43.2 ± 5.8 (d) |
7.2 ± 0.8 (e) |
14.5 ± 2.7 (d) |
22.0 ± 2.8 (b) |
111 ± 7.6 (c) |
9.2 ± 0.3 (c) |
150 |
25.4 ± 2.3 (d) |
39.0 ± 3.7 (e) |
5.4 ± 1.3 (f) |
11.4 ± 2.5 (e) |
19.4 ± 00 (c) |
104 ± 5.8 (d) |
8.4 ± 1.1 (d) |
200 |
20.1 ± 3.7 (e) |
34.0 ± 4.2 (f) |
3.2 ± 0.9 |
8.5 ± 1.8 |
16.5 ± 1.2 (d) |
99.2 ± 4.8 (e) |
8.0 ± 0.6 (e) |
Mean |
29.3 |
45.05 |
8.41 |
16.2 |
22.8 |
111.3 |
9.4 |
Mancozeb |
25 |
34.0 ± 5.7 (a) |
53.8 ± 8.2 (b) |
13.2 ± 5.2 (b) |
23.4 ± 8.1 (a) |
26.9 ± 4.9 (a) |
118.3 ± 12 (b) |
11.0 ± 2.3 (a) |
50 |
31.2 ± 4.4 (b) |
50.3 ± 5.7 (b) |
11.0 ± 1.0 (c) |
20.1 ± 5.5 (b) |
23.4 ± 2.6 (b) |
114.2 ± 11 (c) |
9.3 ± 0.9 (c) |
75 |
28.2 ± 2.2 (c) |
46.4 ± 5.4 (c) |
9.1 ± 2.3 (d) |
16.4 ± 4.4 (c) |
21.4 ± 4.3 (b) |
106 ± 23 (d) |
9.2 ± 0.4 (c) |
100 |
21.3 ± 1.9 (d) |
41.0 ± 6.0 (d) |
7.2 ± 0.8 (e) |
13.2 ± 3.9 (d) |
17.4 ± 2.6 (d) |
97.3 ± 13 (e) |
8.3 ± 1.0 (d) |
150 |
16.2 ± 2.5 (f) |
32.1 ± 2.0 (f) |
5.2 ± 1.2 (f) |
10.2 ± 4.4 (e) |
13.2 ± 0.8 (e) |
86.3 ± 16 (g) |
7.3 ± 2.1 (e) |
200 |
10.8 ± 1.8 (g) |
26.6 ± 2.8 (g) |
3.1 ± 1.0 (g) |
7.4 ± 2.6 (f) |
9.5 ± 0.97 (f) |
79.0 ± 10 (h) |
6.0 ± 0.4 (f) |
Mean |
23.6 |
36.7 |
8.1 |
15.1 |
16.9 |
100.1 |
8.51 |
Similarly, as pesticide concentrations were continuously increased, dry biomass of S. lycopersicum (L.) significantly decreased. As an example, a highest decrease of 81% (16.4 g per plants to 3.1 g per plants) and 66% (25.6 g per plants to 7.4 g per plants) in roots (Fig. 1B) and shoots (Fig. 1C) dry biomass of S. lycoperscium (L.) was recorded when exposed to 200 μgMNZB mL−1 over untreated control. While calculating the correlation between the biometric parameters and biochemical features of pesticide treated S. lycopersicum, root length and root biomass was positively (R2 = 0.77) correlated (Fig. S1 panel A†). The reduction in dry biomass of S. lycopersicum (L.) crops following pesticides application is most likely to occurs as a result of the alteration/inhibition of several enzymes involved in growth expansion, metabolism and physiological activities. Pesticides are generally absorbed by plants through their root systems, from which they are transported to aerial organs. Chemical pesticides are carried to shoots via xylem or phloem, or directly to roots via phloem, after being absorbed by roots. There may be an undeniable decrease in the development of several plant organs following pesticide absorption. The possible explanation for plant growth loss might be the lack of soil organic and inorganic matter (humic acids, fulvic acids etc.) as a result of pesticidal interruption. A similar study demonstrating the phytotoxic behavior of greater pesticide concentrations on bio-features of tomato plants cultivated in pot-house condition are reported.24 Additionally, in comparable crop-based trials, it was discovered that varied pesticide concentrations had a detrimental impact on plant height and dry phytomass of greengram (V. radiata L.) as well as the growth parameters.37 Pesticides (alachlor, butachlor and oxyfluorfen) had a negative impact on the growth of groundnut (Arachis hypogaea L.) by diminishing the height, fresh weight, biomass, yield, seed features, and seed oil content at different tested concentrations.38
Chlorophylls and carotenoids (photoreceptor pigments) are essential in the synthesis of organic compounds like carbohydrates and proteins.39 Plant chlorophyll concentration and other photosynthetic pigments usually decline under abiotic stress conditions. Chlorotoluron, (a herbicide) for example, inhibited higher-order photosynthetic electron transport in plants40 and interrupt the photosystem-II (PS-II) reaction centre. In the photosynthetic pathway, one uracil-type herbicide inhibits both the Hill reaction and PS-II.41 Here, leaf pigments extracted from fresh S. lycopersicum (L.) leaf decreased continuously with an independent rise in pesticide concentration. Among tested pesticides, 200 μg mL−1 mancozeb maximally declined the chl a and chl b content by 91 and 87%, respectively, in comparison to the untreated (control) group (Fig. 1D). Furthermore, the maximum applied doses of MNZB had the greater negative effect and decreased the total chlorophyll (from 4.0 mg g−1 FW to 0.32 mg g−1 FW) and carotenoids content (7.45 mg g−1 FW to 0.65 mg g−1 FW) which is 92% and 91% reduction in terms of phytotoxicity (Fig. 1E). While calculating the correlation between the biometric parameters and biochemical features of pesticide treated S. lycopersicum, a positive correlation was observed for root length and chl a content (R2 = 0.92) (Fig. S1 panel B†), root dry biomass and lycopene content (R2 = 0.79) (Fig. S1 panel C†) and root dry biomass and carotenoid (R2 = 0.88) (Fig. S1 panel D†). Similarly, Mourad et al.42 found that applying systemic fungicide hexaconazole to Phaseolus vulgaris L. (French bean) reduced the chlorophyll and carotenoid pigments. Pesticides have a negative impact on the metabolic enzymes that produce chlorophyll and carotenoids. As a result, it is likely that pesticides employed in this study hindered the activity of photosynthetic carbon reduction enzymes. The study of many photosynthetic pigments and fluorescence characteristics in plants growing in pesticide-stressed settings confirmed that photosynthesis light responses are also extremely sensitive to chemical exposure. Likewise, in a finding, Shakir et al.43 stated that S. lycopersicum (L.) showed negative response to leaf pigments and other growth attributes when exposed to pesticides, and this could be related to a stifling of photosynthetic rate translocation and a decrease in chlorophyll concentration.
3.3 Percent phytotoxicity, percent, tolerance indices and root to shoot length ratio of S. lycopersicum (L.) under pesticide stress
While assessing the phytotoxicity (in terms of percentage), of tested pesticides used here in the present study, it was observed that greater concentrations had highest toxic effect on S. lycopersicum and among them mancozeb showed the most probable adverse effect. For instance, a-89, 83 and 94% phytotoxicity to plants was recorded when exposed to 200 μg mL−1 of each MNZB, IMID and DIZN, respectively compared with control (Fig. 2A). Tolerance indices (TI) of S. lycopersicum plants were significantly decreased with increasing pesticide rates, confirming a negative interrelationship between the tested pesticides and TI. The tolerance index of S. lycopersicum was recorded up to 0.88, 0.75, 0.52, 0.38, 0.24, and 0.12 at 25, 50, 75, 100, 150 and 200 μg mL−1 of MNZB respectively, over untreated control (Fig. 2C). Similarly, survival indices (SI) and root to shoot length ratio of S. lycopersicum (L.) plants were decreased in a pesticide dose related manner (Fig. 2B and D). Like this, a significant decrease in such parameters was observed when chickpeas were uprooted from soils contaminated with increasing concentrations of neonicotnioid groups of pesticides.44
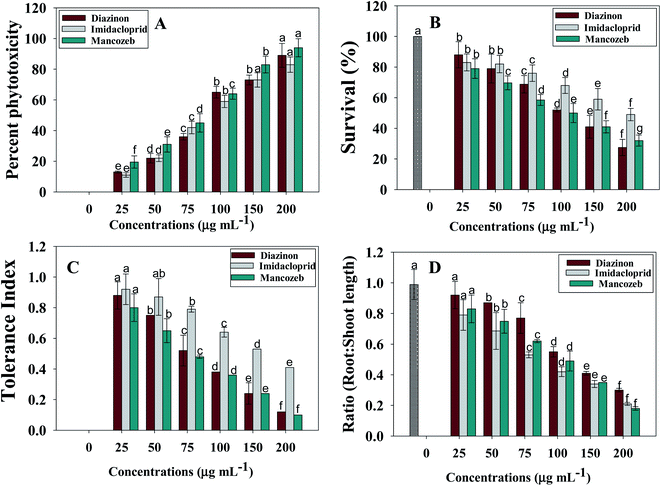 |
| Fig. 2 Percent phytotoxicity (A), survival index (B), tolerance indices (C) and root shoot length ratio (D) S. lycoperscium plants exposed to varying doses (0, 25, 50, 75, 100, 150 and 200 μg mL−1) each of diazinon, imidacloprid and mancozeb. In this figure, bar diagrams represents the mean values of five replicates (n = 5). Corresponding error bars represents standard deviation (SD) of five replicates (SD, n = 5). | |
3.4 Pesticides decreased the flower/fruit attributes of S. lycopersicum (L.)
Pesticides are generally absorbed by plants through their root systems, from which they are transported to aerial organs (flowers and fruits). In terms of public health concern and action, pesticide residues in food have historically fallen considerably behind many related risks.45 Pesticide residue contamination of food is a global issue with obvious ramifications for human health and international trade.46
Here, pesticide had a varying effect on the quantity of flowers produced per plant and fruit attributes like number of fruits/branch, number of fruits/plants, size of fruits and ripening age of fruits. Overall, all pesticides showed a dose-dependent reduction in floral and fruit characteristics. Mancozeb among tested pesticides were shown to be more toxic to floral development than diazinon and imidacloprid. For instance, mancozeb at 200 μg mL−1 significantly decreased flower number borne on per plants by 90% over those flowers formed on untreated one (Table 3). All three pesticides significantly decreased the number of tomato fruits per plant and each branch. For instance, MNZB application among tested pesticides had the maximum phytotoxic effect and decreased the fruit number branch−1, fruit number branch−1, fruit size, fruit fresh weight and fruit biomass by 67, 71, 66, 73 and 79% with respect to untreated (control) plants (Table 3). While comparing the influence of tested pesticide on fruit ripening of tomatoes, the pattern of toxicity was observed in the order: MNZB > DIZN > IMID (Table 3). While calculating the correlation between the biometric parameters and biochemical features of pesticide treated S. lycopersicum, root length and number of fruit/plants (R2 = 0.84) (Fig. S1 panel E†) and root dry biomass and fruit biomass (R2 = 0.83) (Fig. S1 panel E†) showed a positive relationship. The decline or decrease in such indicators (flower and fruit attributes) might be attributable to absorption and transport of pesticides to aerial organs, eventually resulting in cellular death. Pesticides interact with numerous cellular systems after absorption, producing cellular damage and, in certain cases, the destruction of entire plants, either gradually or rapidly. This stifling of plant development might be caused by a decrease of cellular turgor, which disrupts cell elongation. Changes in biological components like as proteins, enzymes, and nucleic acids induced by ROS generation has been linked to plant inhibition or cellular damage, in addition to injuries in cellular tissues.47
Table 3 Effect of different concentrations of test pesticides on fruit attributes of S. lycopersicum (L.) plantsa
Pesticides |
Concentrations used (μg mL−1) |
Flowers number/plant |
Number of fruits/branch |
Number of fruits/plant |
Fruit size (cm) |
Fruit fresh mass (g) |
Fruit dry biomass (g) |
Fruit ripening (unit per plant) |
Moisture content (%) |
Mean |
Values are mean of three independent replicates. |
Control |
0 |
20.0a |
3.0a |
7.0a |
3.74a |
19.4a |
2.10a |
1.10a |
94.2a |
18.7a |
Diazinon |
25 |
18.0b |
2.0b |
6.0b |
3.10a |
18.0a |
1.80b |
1.00a |
94.0a |
17.9a |
50 |
16.0c |
2.0b |
6.0b |
2.65b |
16.3b |
1.40c |
0.66b |
92.2b |
17.1a |
75 |
14.0d |
1.5b |
5.0c |
2.20b |
15.3c |
1.34d |
0.66b |
92.0b |
16.5b |
100 |
12.0d |
1.4b |
4.0d |
1.89c |
14.0c |
1.10e |
0.44c |
92.0b |
15.8b |
150 |
10.0e |
1.0d |
3.0e |
1.78c |
12.0e |
0.87f |
0.33d |
92.0b |
15.1c |
200 |
7.00f |
1.0d |
2.0f |
1.20d |
9.2 |
0.52g |
0.22e |
00 |
2.6e |
Mean |
12.8 |
1.4 |
4.3 |
2.10 |
14.1 |
1.1 |
0.5 |
77.0 |
14.1 |
Imidacloprid |
25 |
19.0b |
3.0a |
6.0b |
3.45a |
18.4a |
2.20a |
1.00a |
94.2b |
18.4a |
50 |
18.0b |
3.0a |
5.0c |
3.12a |
17.0b |
2.00a |
0.66b |
94.2b |
17.8a |
75 |
16.0c |
3.0a |
5.0c |
2.54b |
15.4c |
1.97b |
0.66b |
93.7b |
17.2a |
100 |
15.0c |
2.0b |
5.0c |
2.10c |
13.5d |
1.56c |
0.55b |
93.7b |
16.6b |
150 |
13.0d |
2.0b |
4.0d |
2.00c |
11.0e |
1.32d |
0.44c |
92.2c |
15.7b |
200 |
11.0e |
2.0v |
3.0e |
1.40d |
10.2f |
1.10e |
0.33d |
92.0c |
15.1c |
Mean |
15.3 |
2.5 |
4.6 |
2.40 |
14.2 |
1.69 |
0.6 |
93.3 |
16.8 |
Mancozeb |
25 |
18.0b |
2.5a |
5.0c |
2.40b |
17.4b |
2.00a |
1.0a |
92.0c |
17.5a |
50 |
16.0c |
2.0b |
4.5c |
2.10c |
15.7c |
1.77b |
0.66b |
92.0c |
16.8b |
75 |
14.0d |
1.5b |
4.0d |
2.00c |
13.2d |
1.54c |
0.55b |
91.7d |
16.0c |
100 |
11.0e |
1.2c |
3.5d |
1.40d |
11.4e |
1.23d |
0.44c |
91.0d |
15.1c |
150 |
7.00f |
1.0d |
2.5e |
1.20d |
9.30f |
0.78f |
0.33d |
91.0d |
14.1d |
200 |
2.00g |
1.0d |
2.0f |
1.10e |
5.30g |
0.43h |
00 |
00 |
1.4f |
Mean |
11.3 |
1.5 |
3.5 |
1.70 |
12.05 |
1.2 |
0.49 |
76.2 |
13.4 |
3.5 Pesticide decreases soluble protein, total soluble sugar (TSS) and lycopene content in S. lycopersicum (L.)
The soluble protein (TSP) content in pesticide-stressed tomato seedlings was found to be significantly decreased. Among the tested pesticides, MNZB had the most negative impacts on protein content where it reduced the TSP content by 66% over untreated control (Table 2). The fact that the toxicant created as a result of pesticide application delayed protein synthesis by modifying cytochrome oxidase activity, limiting alternative respiratory pathways, and producing succinate could explain the drop in total soluble protein levels in response to pesticide toxicity. Several stressors, including xenobiotics, have been studied to see how they alter total protein concentration in plants.48 The protein content of Vigna radiata L. (greengram) was reduced after foliar spraying of chlorpyrifos pesticide.49
Pesticide treatment at higher than allowed dosages resulted in a significant drop in sugar content, extracted from root tissues of S. lycopersicum (L.). Because root tissues of plants act as a sink for the buildup of soluble sugar generated by the process of chlorophyll formation, a delay in this process resulting in a decreased level of total soluble sugar. Plants create phenolic chemicals in reaction to stress, which can slow overall development by inhibiting the biosynthetic process of chlorophyll creation or by stimulating the breakdown pathway, which leads to lower chlorophyll generation and, eventually, photosynthesis and formation of sugar.50 Our findings support the conclusion of Parween et al.49 who reported that greater doses of chlorpyrifos reduced the quantity of soluble sugar in greengram seedlings. The amount of lycopene in tomato fruits cultivated in pesticide-treated and untreated soils was dissimilar. In general, the pesticides lowered lycopene levels in a dose–response fashion (maximally reduced by 60% at 200 μgMNZB mL−1 over untreated plants sample) (Table 2).
3.6 Pesticide application modulated the antioxidant enzymes in foliage of S. lycopersicum (L.)
Higher levels of antioxidants are frequently related with increased stress tolerance in plants subjected to diverse stressors, notably through the augmentation of antioxidant enzyme activity.51 Antioxidant defenses are developed by plants to deal with oxidative stress, which includes both antioxidant enzymes like SOD, POD, APX, GR, CAT and non-enzymatic antioxidants that scavenge free radicals, such as AsA and proline, among others and peroxide.52 Superoxide dismutase (SOD) is a crucial antioxidant enzyme which is the first line of defense against superoxide by catalyzing the dismutation of superoxide to molecular oxygen and hydrogen peroxide (H2O2) against free radicals.53 APX, POD and CAT have been linked to H2O2 scavenging.54 Non-enzymatic antioxidants include phenolic substances, carotenoids, ascorbate, and various nitrogenous metabolites such amino acids, especially proline, which has considerable antioxidant action needed to counteract the harmful effects of reactive oxygen species.55 These enzymes' activity changes suggest redox changes caused by oxidative stress.
Considering the imperative role of antioxidative defense enzymes in plant raised under changing environment, we assessed the enzymatic responses of S. lycopersicum (L.) when exposed to increasing pesticide doses. In general, activity of antioxidant enzymes increased as pesticide doses increased (in a dose-dependent manner). As an example, APX activity of S. lycopersicum (L.) was increased by 18%, 22%, 25%, 28%, 34% and 53% when plants were detached from soils polluted with 25, 50, 75, 100, 150 and 200 μgMNZB mL−1, respectively, compared with untreated control (6.1 μmol min−1 g−1 FW) (Fig. 3A). Likewise, GPX (Fig. 3B), CAT (Fig. 3E) and POD (Fig. 3C) activities were maximally and significantly (p ≤ 0.05) increased by 95%, 93% and 65% when S. lycopersicum (L.) plants were exposed to 200 μg mL−1 each of IMID, MNZB and DIZN, respectively, over untreated control. While calculating the correlation between the antioxidant enzymes and oxidative stress parameters of pesticide exposed S. lycopersicum plants, SOD activity and proline content were positively correlated (R2 = 0.79) (Fig. S2 panel A†), while, POD activity showed moderate correlation (R2 = 0.61) with total soluble sugar (Fig. S2 panel B†).
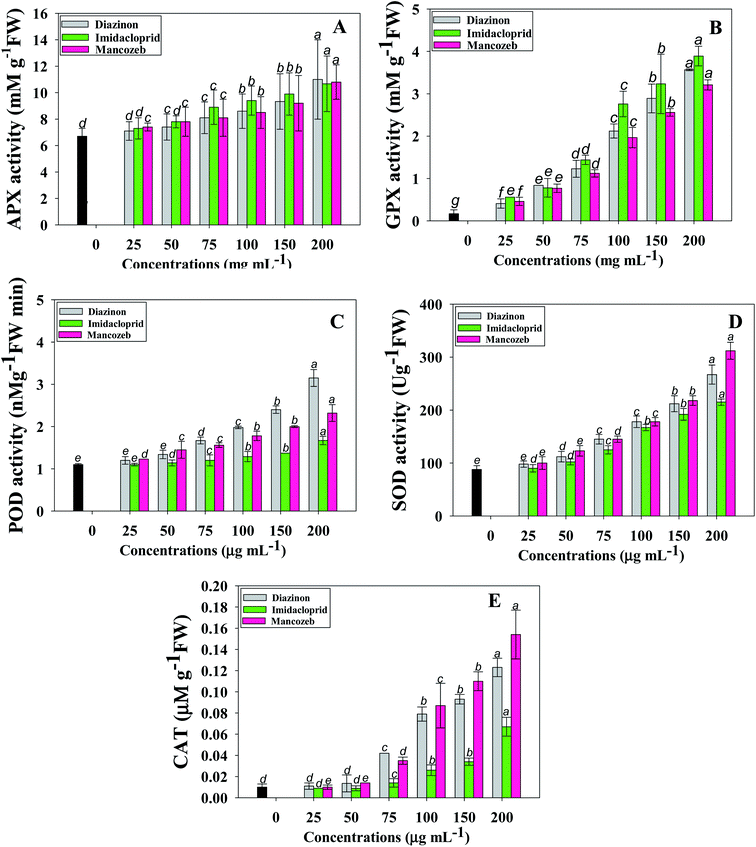 |
| Fig. 3 Antioxidant enzymes; APX (A), GPX (B), POD (C) SOD (D) and CAT (E) extracted from roots of Solanum lycoperscium plants exposed to varying doses (0, 25, 50, 75, 100, 150 and 200 μg mL−1) each of diazinon, imidacloprid and mancozeb. In this figure, bar diagrams represents the mean values of five replicates (n = 5). Corresponding error bars represents standard deviation (SD) of five replicates (SD, n = 5). | |
Similar to our study, a comparable rise in CAT activity has been documented in soybean (Glycine max L.) plants grown in soils polluted with deltamethrin herbicide.56 Similarly, significant changes in antioxidative responses including peroxidase (POX) and catalase (CAT), have been identified in Medicago sativa plants, which are required to ameliorate the oxidative damage produced by various environmental stresses.57
3.7 Pesticides exposure causes modulations in cell viability and electrolyte leakage (EL)
When plants are exposed to a stressful environment, they frequently produce reactive oxygen species (ROS).58 The buildup of H2O2 and electrolyte leakage is regarded as an effective indicator of stress-induced tissue injury.59 H2O2 is produced as a result of oxidative degradation and a hazardous reactive oxygen species (ROS) with harmful consequences. It is known to disrupt metabolism, affect cellular integrity and oxidative damage, and impair the stability of cellular membrane.60 Plants' membrane structural integrity can be predicted by changes in H2O2 levels in tissues that have been subjected to stressful conditions. The current study found that pesticide treatments had a negative impact on stress indicators (electrolyte leakage and H2O2 content) and that increasing rates substantially increased their concentration. Here, cell viability of shoots and roots were maximally and significantly decreased by 58 and 76% when tomatoes were planted in soil treated with 200 μgMNZB mL−1 and 200 μgDIZN mL−1, respectively, over untreated tomatoes (Fig. 4A). Similarly, pesticides like thiabendazole, plethora and tricyclazole etc. displayed a considerable decrease in the cellular viability of fenugreek (Trigonella foenum L.) and thus confirmed the phytotoxic potential.61 The various concentrations of DIZN, IMID and MNZB displayed a considerable and visible increase in leaf EL, with a maximum of 79, 71 and 80 percent increase measured at higher dosages (200 μg mL−1) of each pesticides, respectively, compared with control (Fig. 4B). Pesticide-exposed tomato seedlings had large levels of electrolyte leakage, indicating that higher pesticide dosages resulted in more cell damage. The seedlings of S. lycopersicum (L.) subjected to pesticides exhibited significant levels of electrolyte leakage, indicating that greater pesticide dosages resulted in more cell damage. Mancozeb, among the pesticides caused more electrolyte leakage (cell injury) than the other chemical pesticides studied, and shoot tissues were more harmed than root tissues. Abiotic stress has also been linked to an increase in cell damage in terms of electrolyte leakage and a decrease in cell viability in plants, according to previous research conducted by Daud et al.62 Glyphosate-treated pea (P. sativum L.) plants have already shown an increase in electrolyte leakage in their leaf tissues as reported by Singh et al.63 which is consistent with this work.
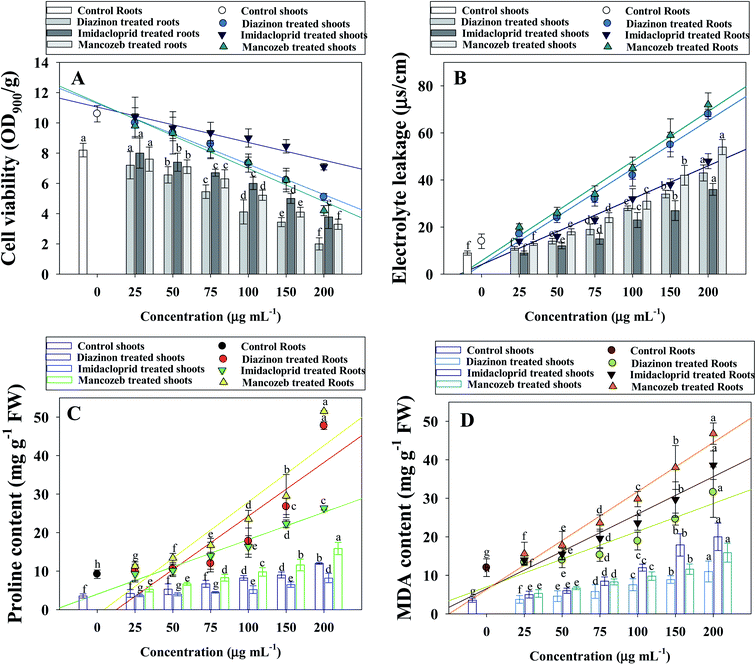 |
| Fig. 4 Cell viability (A), electrolyte leakage (B), proline (C) and MDA content (D) in roots and shoots of S. lycoperscium plants exposed to varying doses (0, 25, 50, 75, 100, 150 and 200 μg mL−1) each of diazinon, imidacloprid and mancozeb. In this figure, bar diagrams represents the mean values of five replicates (n = 5). Corresponding error bars represents standard deviation (SD) of five replicates (SD, n = 5). | |
3.8 Pesticide exposure causes increase in proline and TBARs content
Proline is a key molecule that accumulates in plant tissues to protect them from oxidative damage. The main activities of the plant proline include the detoxification of reactive oxygen species, the membrane integrity protection in plant cells, the stability of enzymes and proteins, osmo protection and increased stress tolerance. Proline production serves as a signal molecule that may be necessary for plant recovery following exposure to environmental stress. Pesticide overdose resulted in a rise in proline levels, particularly in exposed roots, in the current study. Here, the maximum induction (51.5 ± 11.3 μmoles mg g−1 fresh weight proline) of proline was extracted from roots at 200 μgMNZB mL−1 soil. Similarly, in the shoot tissues of the similar dose of MNZB treated S. lycopersicum (L.) plants showed a maximum accumulation of 15.8 ± 5.3 μmol mg g−1 FW of proline was detected (Fig. 4C). Increasing proline content might be linked to cellular dehydration as a reaction to pesticides toxicity in plants treating high levels of pesticides. Abrokwah et al.64 showed a strong relationship between proline and various dosages of acetamiprid and λ-cyhalothrin insecticides in a comparable experiment. In yet, similar investigation, an enhanced amount of proline accumulation has been discovered in plant roots grown in soil polluted with various levels of malathion.65
Inhibition of antioxidant defense mechanisms or damage to biological systems can cause oxidative stress. Among the different stress indicators, formation of TBARSs demonstrates the importance of lipid peroxidation. Here, like other oxidative stress parameters, TBARSs content were progressively increased with increasing doses (Fig. 4D). The increasing amount of TBARS in pesticide-treated S. lycopersicum (L.) seedlings revealed a significant degree of membrane damage during pesticide stress exposure. It may be linked to a larger concentration of ROS in root tissues, which caused membrane damage owing to lipid peroxidation and, as a result, the generation of TBARS. The roots tissues of Oryza sativa (L.) showed an increased production of oxidative stress and ROS (lipid peroxidation) when exposed to bisphenol A (BPA), as evidenced by raised levels of TBARS content.66 While calculating the correlation between the antioxidant enzymes and stress marker of pesticide exposed S. lycopersicum, CAT activity positively correlated (R2 = 0.81) with TBARSs (Fig. S2 panel C†). Similarly, proline content and root cell viability were positively (R2 = 0.92) correlated with each other (Fig. S2 panel D†). In a comparative assessment, Parween et al.37 showed a significant upsurge in TBARS levels in when plant were cultivated in soils exposed to increasing chlorpyrifos doses. Deltamethrin and some other chemical pesticides enhanced the TBARS concentrations in different organs (roots, shoots, and leaves tissues) of soybean.56 The occurrence of greater levels of TBARS in the this study implies that ROS-mediated plant damage may be one of the most significant phytotoxic consequences of tested pesticides.
3.9 Pesticide-induced ROS generation, changes in mitochondrial membrane potential (ΔΨm) and cellular death in S. lycopersicum (L.)
Plants go through a number of stress acclimation processes, including gene regulation involved in oxidative stress responses, which results in induction of antioxidant enzymes, to protect cells from excessive accumulation of reactive oxygen species caused by exposure to various environmental pollutants. Various types of ROS are produced in different compartments of the cell under stress circumstances. The activation of antioxidant enzyme genes in response to oxidative stress is a valuable indication for investigating plants' antioxidant defense mechanisms.
Using in vivo histo-chemical staining with fluorescent dyes, effect of various doses of test pesticides on ROS-production in S. lycopersicum roots was qualitatively evaluated. As exposed to increasing concentrations of pesticides, histochemical staining with 3,3′-diaminobenzidine (DAB) and treatment with dichloro–dihydro–fluorescein diacetate (DCFH-DA) revealed an increase in ROS production in root tissues, with higher ROS production in root tips region, compared to untreated controls (Fig. 5). Likewise, over-production of ROS in 2,4-D treated young and adult leaves of Pisum sativum (garden pea) has been reported.67 Furthermore, Erinle et al.68 showed atrazine-induced oxidative stress and ROS over production in Pennisetum. Additionally, dichlorprop (DCPP) herbicide caused production of ROS in root and leaves tissues of Arbidopsis thaliana which as clearly evidenced under confocal laser scanning microscopy.69
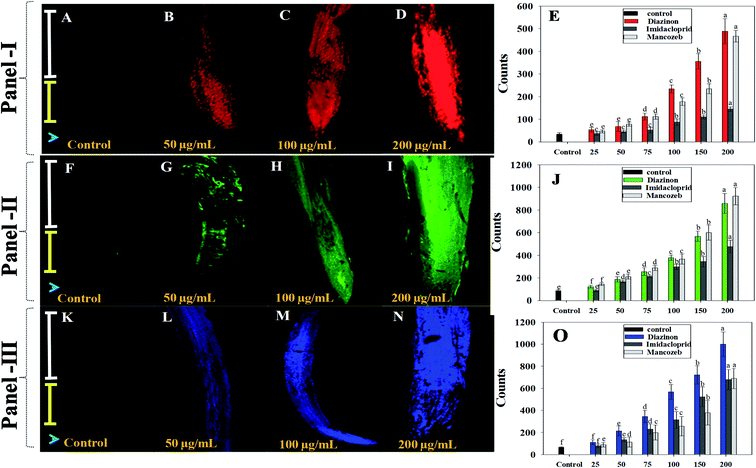 |
| Fig. 5 Here, panel-I depict the qualitative and quantitative assessment (E) of pesticide-induced changes in mitochondrial membrane potential of root tissues of S. lycoperscium. (A)–(D) shows the CLSM images of Rhodamine 123 (Rh 123) stained roots exposed to 0 (control), 50, 100 and 200 μg mL−1 of mancozeb, respectively, whereas, (E) shows the quantification of pesticide-induced changes in Rhodamine 123 (Rh 123) fluorescence. In panel-II, (F) (control), (G) (50 μg mL−1), (H) (100 μg mL−1), (I) (200 μg mL−1) and (J) (quantification of ROS) shows the reactive oxygen species generation in root tissues of S. lycoperscium plants cultivated with different rates pesticides as revealed by staining with 3,3′-diaminobenzidine (DAB) and 2′,7′ dichlorodihydrofluorescein diacetate (DCFH-DA). In panel-III, (K)–(N) depict the increase in blue fluorescence after staining with Evan's blue dye in root tissues of S. lycoperscium treated with 0, 50, 100 and 200 μg mL−1 of mancozeb, respectively. Panel O represents the spectrophotometric quantification of Evan's blue dye in root tissues. White and yellow scale bars on left side indicate root elongation and meristematic zones, respectively, while blue arrow head points towards root tip. Bar diagrams represents the mean values of five replicates (n = 5). Corresponding error bars represents standard deviation (SD) of five replicates (SD, n = 5). | |
Furthermore, as a result of exposure to increasing pesticidal concentrations, alterations in mitochondrial membrane potential were detected in root tissues of DIZN, IMID and NNZB-exposed plants, as shown by enhanced Rh123 fluorescence (Fig. 5). Because mitochondria are cell's primary source of ROS, pesticides interactions with mitochondrial electron transport system (METS) may have resulted in excessive ROS generation70 as indicated by higher Rh123 fluorescence levels, leading in a decrease in ΔΨm. Like our study, Nair and Chung71 observed that exposure of abiotic stress in rice plants caused a significant alteration in ΔΨm. Similarly, changes in mitochondrial membrane potential of S. lycopersicum (L.) seedlings (as evident by increased Rh123 fluorescence due to alterations in mitochondrial membrane permeability) under stress condition has been reported.34
When pesticides exposed S. lycopersicum (L.) roots were tagged with Evans blue dye, the loss of plasma membrane was visible. The absorption of dye by root tissues increased 2–3-fold when the concentration of pesticides was increased, resulting in plasma membrane integrity deficits. In comparison to untreated control, roots treated with 200 μg mL−1 of each pesticide showed enhanced intensity of blue color fluorescence. This clearly reflects Evans blue penetration into non-viable cells. These findings imply that root cells lost their ability to heal after being exposed to pesticides, and hence dyed blue. These data support the notion that some pesticides, when applied to roots, might cause cell rupture, resulting in cell death. Several theories have been postulated by workers as to how increased rates of pesticides delay the biological properties, which could provide greater insight into retardation and killing of cells growth, as demonstrated in this work. Pesticidal residues and other contaminants present in soil tends to reduce water and nutrient intake as they become linked to soil particles and influence nutrient uptake from the soil to the root system. Pesticide residues may have an impact on transport of micronutrient and plasma membrane of root cells, causing cation absorption to be disrupted. Likewise, increased blue fluorescence in fungicides treated P. sativum roots was observed as a result of uptake of Evan's blue dye are reported by other workers.
4. Conclusion
The current findings provide information to help better comprehend the finer elements of pesticide toxicity's molecular basis to edible vegetable crops in general and tomatoes in particular. Pesticide excesses exhibited phytotoxic impact on biological and physiological traits of S. lycopersicum L. (tomato) seedlings by inducing oxidative stress. Among tested pesticides, mancozeb showed maximum toxic effect to overall physiology of plants. A statistically significant correlation was found between physiological and oxidative stress parameters of pesticides exposed S. lycopersicum. The current findings are critical for understanding the response mechanisms of plants to pesticide stress. Since, pesticides are translocated to edible plant parts (fruits) through root systems. When these contaminated vegetables are consumed by humans via food chain, it causes several cardiovascular diseases. Therefore, pesticide use must be closely monitored and regulated in order to prevent poisonous substances from entering the environment, posing a major threat to human and animal health. Further there is an urgent need to find a molecular approach to have a better understanding of pesticide-induced phytotoxicity in edible vegetable crops including S. lycopersicum (L.). Morevore, research is needed to assess the pesticides' oxidative stress-inducing pathways in S. lycopersicum (L.) in order to lessen their harmful effects on host crops in the future. In addition, research should be directed toward identifying cell defensins and cell kinases that are activated in response to pesticide-induced toxicity. Alternative safe methods, such as the development of comparably less expensive bio-pesticides, should be supported.
Conflicts of interest
The authors have declared there is no conflict of interest.
Acknowledgements
The authors extend their appreciation to the Research Supporting Project number (RSP-2021/316) King Saud University, Riyadh, Saudi Arabia.
References
- J. Popp, K. Pető and J. Nagy, Pesticide productivity and food security. A review, Agron. Sustainable Dev., 2013, 33, 243–255 CrossRef
. - A. Sharma, V. Kumar, B. Shahzad, M. Tanveer, G. P. S. Sidhu, N. Handa, S. K. Kohli, P. Yadav, A. S. Bali, R. D. Parihar and O. I. Dar, Worldwide pesticide usage and its impacts on ecosystem, SN Appl. Sci., 2019, 1, 1–16 Search PubMed
. - A. C. Almeida, T. Gomes, K. Langford, K. V. Thomas and K. E. Tollefsen, Oxidative stress potential of the herbicides bifenox and metribuzin in the microalgae Chlamydomonas reinhardtii, Aquat. Toxicol., 2019, 210, 117–128 CrossRef CAS PubMed
. - R. Pechová, J. Kutík, D. Holá, M. Kočová, D. Haisel and A. Vičánková, The ultrastructure of chloroplasts, content of photosynthetic pigments, and photochemical activity of maize (Zea mays L.) as influenced by different concentrations of the herbicide amitrole, Photosynthetica, 2003, 41, 127–136 CrossRef
. - D. C. Bailey, C. E. Todt, A. S. Burchfield, A. S. Pressley, R. D. Denney, I. B. Snapp, R. Negga, W. L. Traynor and V. A. Fitsanakis, Chronic exposure to a glyphosate-containing pesticide leads to mitochondrial dysfunction and increased reactive oxygen species production in Caenorhabditis elegans, Environ. Toxicol. Pharmacol., 2108, 57, 46–52 CrossRef PubMed
. - M. Shahid, S. Manoharadas, H. Chakdar, A. F. Alrefaei, M. F. Albeshr and M. H. Almutairi, Biological toxicity assessment of carbamate pesticides using bacterial and plant bioassays: An in-vitro approach, Chem, 2021, 278, 130372 CAS
. - H. Shao and Y. Zhang, Non-target effects on soil microbial parameters of the synthetic pesticide carbendazim with the biopesticides cantharidin and norcantharidin, Sci. Rep., 2017, 7, 1–12 CrossRef CAS
. - C. Azpiazu, J. Bosch, E. Viñuela, P. Medrzycki, D. Teper and F. Sgolastra, Chronicoral exposure to field-realistic pesticide combinations via pollen and nectar: effects on feeding and thermal performance in a solitary bee, Sci. Rep., 2019, 9, 1–11 CAS
. - E. Adams, C. Leeb and C. A. Brühl, Pesticide exposure affects reproductive capacity of common toads (Bufo bufo) in a viticultural landscape, Ecotoxicol, 2021, 30, 213–223 CrossRef CAS
. - M. Shahid and M. S. Khan, Assessment of glyphosate and quizalofop mediated toxicity to greengram [Vigna radiata (L.) Wilczek], stress abatement and growth promotion by herbicide tolerant Bradyrhizobium and Pseudomonas species, Int. J. Curr. Microbiol. Appl. Sci., 2017, 6, 3001–3016 CrossRef
. - M. Shahid, M. S. Khan and M. Kumar, Kitazin-pea interaction: understanding the fungicide induced nodule alteration, cytotoxicity, oxidative damage and toxicity alleviation by Rhizobium leguminosarum, RSC Adv., 2019, 9, 16929–16947 RSC
. - M. Shahid and M. S. Khan, Glyphosate induced toxicity to chickpea plants and stress alleviation by herbicide tolerant phosphate solubilizing Burkholderia cepacia PSBB1 carrying multifarious plant growth promoting activities, 3 Biotech, 2018, 8, 1–17 Search PubMed
. - S. Sachdev, S. A. Ansari, M. I. Ansari, M. Fujita and M. Hasanuzzaman, Abiotic Stress and Reactive Oxygen Species: Generation, Signaling, and Defense Mechanisms, Antioxidants, 2021, 10, 277 CrossRef CAS PubMed
. - C. M. Bergamini, S. Gambetti, A. Dondi and C. Cervellati, Oxygen, reactive oxygen species and tissue damage, Curr. Pharm. Des., 2004, 10, 1611–1626 CrossRef CAS PubMed
. - R. Ahmad, S. Hussain, M. A. Anjum, M. F. Khalid, M. Saqib, I. Zakir, A. Hassan, S. Fahad and S. Ahmad, Oxidative stress and antioxidant defense mechanisms in plants under salt stress, in Plant abiot str, Springer, Cham, 2019, pp. 191–205 Search PubMed
. - S. F. Wahedally, F. A. Mamboya, T. J. Lyimo and M. Bhikajee, Short-term effects of three herbicides on the maximum quantum yield and electron transport rate of tropical seagrass Thalassodendron ciliatum, Tanz. J. Nat. Appl. Sci., 2012, 3, 458–466 Search PubMed
. - A. Rezk, M. Abhary and A. Akhkha. Tomato (Solanum lycopersicum L.) Breeding Strategies for Biotic and Abiotic Stresses, in Advances in Plant Breeding Strategies: Vegetable Crops, Springer, Cham, 2021, pp. 363–405 Search PubMed
. - F. A. O. Food and Agricultural Organization Stat, core production, 2007, http://faostat.fao.org/site/340/default.aspx.
- A. Kumar, V. Kumar, A. Gull and G. A. Nayik. Tomato (Solanum Lycopersicon), in Antioxidants in Vegetables and Nuts-Properties and Health Benefits, Springer, Singapore 2020, pp. 191–207 Search PubMed
. - A. Biondi, R. N. C. Guedes, F. H. Wan and N. Desneux, Ecology, worldwide spread, and management of the invasive South American tomato pinworm, Tuta absoluta: past, present, and future, Annu. Rev. Entomol., 2018, 63, 239–258 CrossRef CAS
. - R. Sanoubar and L. Barbanti, Fungal diseases on tomato plant under greenhouse condition, Eur. J. Biol. Res., 2017, 7, 299–308 Search PubMed
. - B. Gupta, M. Rani and R. Kumar, Degradation of thiram in water, soil and plants: a study by high-performance liquid chromatography, Biomed. Chromatogr., 2012, 26, 69–75 CrossRef CAS PubMed
. - E. V. Baibakova, E. E. Nefedjeva, M. Suska-Malawska, M. Wilk, G. A. Sevriukova and V. F. Zheltobriukhov, Modern fungicides: Mechanisms of action, fungal resistance and phytotoxic effects, Annu. Res. Rev. Biol., 2019, 1–16 Search PubMed
. - S. K. Shakir, M. Kanwal, W. Murad, Z. Rehman, S. Rehman, M. K. Daud and A. Azizullah, Effect of some commonly used pesticides on seed germination, biomass production and photosynthetic pigments in tomato (Lycopersicon esculentum), Ecotoxicol, 2016, 25, 329–341 CrossRef CAS PubMed
. - M. Shahid and M. S. Khan, Fungicide tolerant Bradyrhizobium japonicum mitigate toxicity and enhance greengram production under hexaconazole stress, J. Environ. Sci., 2019, 78, 92–108 CrossRef PubMed
. - D. I. Arnon, Copper enzymes in isolated chloroplasts. Polyphenoloxidase in Beta vulgaris, Plant Physiol., 1949, 24, 1 CrossRef CAS PubMed
. - C. H. Chou and H. J. Lin, Autointoxication mechanism of Oryza sativa I. Phytotoxic effects of decomposing rice residues in soil, J. Chem. Ecol., 1976, 2, 353–367 CrossRef CAS
. - M. Z. Iqbal and K. Rahmati, Tolerance of Albizia lebbeck to Cu and Fe application, Ekológia, 1992, 11, 427–430 Search PubMed
. - M. M. Bradford, A rapid and sensitive method for the quantization of microgram quantities of protein utilizing the principle of protein dye binding, Anal. Biochem., 1976, 72, 248–259 CrossRef CAS PubMed
. - R. Shields and W. Burnett, Determination of protein-bound carbohydrate in serum by a modified anthrone method, Anal. Chem., 1960, 32, 885–886 CrossRef CAS
. - L. S. Bates, R. P. Waldren and I. D. Teare, Rapid determination of free praline for water stress studies, Plant Soil, 1973, 39, 205–2017 CrossRef CAS
. - D. M. Hodges, J. M. DeLong, C. F. Forney and R. K. Prange, Improving the thiobarbituric acid-reactive-substances assay for estimating lipid peroxidation in plant tissues containing anthocyanin and other interfering compounds, Planta, 1991, 207, 604–611 CrossRef
. - S. Lu, Z. Yuejing, Y. Niu and Z. Bingru, Antioxidant responses of radiation-induced dwarf mutants of bermuda grass to drought stress, J. Am. Soc. Hortic. Sci., 2008, 133, 360–366 Search PubMed
. - M. Faisal, Q. Saquib, A. A. Alatar, A. A. Al-Khedhairy, A. K. Hegazy and J. Musarrat, Phytotoxic hazards of NiO-nanoparticles in tomato: a study on mechanism of cell death, J. Hazard. Mater., 2013, 250, 318–332 CrossRef PubMed
. - N. Rajashekar and T. S. Murthy, Seed germination and physiological behavior of maize (cv. NAC-6002) seedlings under abiotic stress (pendimethalin) condition, Asian J. Crop Sci., 2012, 4, 80–85 CrossRef
. - V. Mishra, G. Srivastava, S. M. Prasad and G. Abraham, Growth, photosynthetic pigments and photosynthetic activity during seedling stage of cowpea (Vigna unguiculata) in response to UV-B and dimethoate, Pestic. Biochem. Physiol., 2008, 92, 30–37 CrossRef CAS
. - T. A. Parween, S. Jan and T. Fatma, Evaluation of oxidative stress in Vigna radiata L. in response to chlorpyrifos, Int. J. Environ. Sci. Technol., 2012, 9, 605–612 CrossRef CAS
. - S. K. Sahoo, J. Pradhan, V. B. Kuruwanshi, A. Guhey, G. R. Rout and R. Dash, Phytotoxic effect of pre-emergence herbicides on oil content and yield components of groundnut (Arachis hypogeae), Int. J. Curr. Microbiol. Appl. Sci., 2017, 6, 1738–1748 CrossRef
. - J. Gross. Pigments in vegetables: chlorophylls and carotenoids, Spring Sci. Bus. Med., 2012 Search PubMed
. - E. P. Fuerst and M. A. Norman, Interactions of herbicides with photosynthetic electron transport, Weed Sci., 1991, 39, 458–464 CrossRef CAS
. - P. Barry, A. J. Young and G. Briton, Photo-destruction in higher plants by herbicide action, J. Exp. Bot., 1990, 41, 123–126 CrossRef CAS
. - B. Mourad, B. Baha-Eddine and B. Mokhtar, The impact of a hexaconazole fungicide on agronomic, biochemical parameters and yield components of green beans PhaseolusVulgaris cv. Djedida, Int. J. Adv. Eng. Manag., 2107, 2, 146–152 CrossRef
. - S. K. Shakir, S. Irfan, B. Akhtar, S. ur Rehman, M. K. Daud, N. Taimur and A. Azizullah, Pesticide-induced oxidative stress and antioxidant responses in tomato (Solanum lycopersicum) seedlings, Ecotoxicol, 2018, 27, 919–935 CrossRef CAS PubMed
. - S. Khan, M. Shahid, M. S. Khan, A. Syed, A. H. Bahkali, A. M. Elgorban and J. Pichtel, Fungicide-tolerant plant growth-promoting rhizobacteria mitigate physiological disruption of white radish caused by fungicides used in the field cultivation, Int. J. Environ. Res. Public Health, 2020, 17, 7251 CrossRef PubMed
. - Y. Lu, S. Song, R. Wang, Z. Liu, J. Meng, A. J. Sweetman, A. Jenkins, R. C. Ferrier, H. Li, W. Luo and T. Wang, Impacts of soil and water pollution on food safety and health risks in China, Environ. Int., 2015, 77, 5–15 CrossRef CAS PubMed
. - D. J. Ecobichon, Pesticide use in developing countries, Toxicol, 2021, 160, 27–33 CrossRef
. - T. Karuppanapandian, J. C. Moon, C. Kim, K. Manoharan and W. Kim, Reactive oxygen species in plants: their generation, signal transduction, and scavenging mechanisms, Aust. J. Crop Sci., 2011, 5, 709–725 CAS
. - A. Alayat, L. Souiki, N. Grara, M. R. Djebar, Z. E. Boumedris, S. Benosmane, R. Amamra and H. Berrebbah, Effects of cadmium on water content, soluble protein, proline changes and some antioxidant enzymes in wheat (Triticum durum desf.) leaves, Annu. Res. Rev. Biol., 2014, 3835–3847 CrossRef
. - T. Parween, S. Jan and T. Fatma, Assessing the impact of chlorpyrifos on growth, photosynthetic pigments and yield in Vigna radiata L. at different phenological stages, Afr. J. Agric. Res., 2011, 6, 4432–4440 Search PubMed
. - C. M. Yang, C. N. Lee and C. H. Chou, Effects of three allelopathic phenolics on chlorophyll accumulation of rice (Oryza sativa) seedlings: I. Inhibition of supply-orientation, Bot. Bull. Acad. Sin., 2002, 43, 299–304 CAS
. - M. A. Ahanger, N. S. Tomar, M. Tittal, S. Argal and R. M. Agarwal, Plant growth under water/salt stress: ROS production; antioxidants and significance of added potassium under such conditions, Physiol. Mol. Biol. Plants, 2019, 23, 731–744 CrossRef PubMed
. - S. S. Gill and N. Tuteja, Reactive oxygen species and antioxidant machinery in abiotic stress tolerance in crop plants, Plant Physiol. Biochem., 2010, 48, 909–930 CrossRef CAS PubMed
. - O. M. IghodarO and O. A. Akinloye, First line defence antioxidants-superoxide dismutase (SOD), catalase (CAT) and glutathione peroxidase (GPX): Their fundamental role in the entire antioxidant defence grid, Alex. J. Med., 2018, 54, 287–293 Search PubMed
. - R. Y. Yordanova, K. N. Christov and L. P. Popova, Antioxidative enzymes in barley plants subjected to soil flooding, Environ. Exp. Bot., 2004, 51, 93–101 CrossRef CAS
. - A. M. Abbasi, M. H. Shah and M. A. Khan, Phytochemicals and nutraceuticals, in Wild Edible Vegetables of Lesser Himalayas, Springer, Cham, 2015, pp. 31–65 Search PubMed
. - F. Bashir, T. O. Siddiqi and M. O. Iqbal, The antioxidative response system in Glycine max (L.) Merr. exposed to deltamethrin, a synthetic pyrethroid insecticide, Environ. Poll., 2007, 147, 94–100 CrossRef CAS PubMed
. - K. Kodin, Effect of drought stress on the oxidative enzyme activity in Medicago sativa, University of Nebraska at Kearney, 2017 Search PubMed
. - A. R. Devireddy, S. I. Zandalinas, Y. Fichman and R. Mittler, Integration of reactive oxygen species and hormone signaling during abiotic stress, Plant J., 2021, 105, 459–476 CrossRef CAS PubMed
. - L. Y. Wang, J. L. Liu, W. X. Wang and Y. Sun, Exogenous melatonin improves growth and photosynthetic capacity of cucumber under salinity-induced stress, Photosyn, 2016, 54, 19–27 CrossRef CAS
. - D. R. Janero, D. Hreniuk and H. M. Sharif, Hydrogen peroxide-induced oxidative stress to the mammalian heart-muscle cell (cardiomyocyte): Lethal peroxidative membrane injury, J. Cell. Physiol., 1991, 149, 347–364 CrossRef CAS PubMed
. - K. Mahapatra, S. De, S. Banerjee and S. Roy, Pesticide mediated oxidative stress induces genotoxicity and disrupts chromatin structure in fenugreek (Trigonella foenum-graecum L.) seedlings, J. Hazard. Mater., 2019, 369, 362–374 CrossRef CAS PubMed
. - S. Daud, P. Nambiar, M. Z. Hossain, M. R. A. Rahman and M. M. Bakri, Changes in cell density and morphology of selected cells of the ageing human dental pulp, Gerodontol, 2012, 33, 315–321 CrossRef PubMed
. - H. Singh, N. B. Singh, A. Singh, I. Hussain and V. Yadav, Physiological and biochemical roles of nitric oxide against toxicity produced by glyphosate herbicide in Pisum sativum, Russ. J. Plant Physiol., 2017, 64, 518–524 CrossRef CAS
. - F. K. Abrokwah, M. Matthe, P. C. Dans and A. A. Affoh AA, Study on the effects of acetamiprid and lambda-cyhalothrin application on some biochemical parameters of onion and tomato, Int. Res. J. Food Nut., 2019, 1, 10–17 Search PubMed
. - A. K. Srivastava and D. Singh, Assessment of malathion toxicity on cytophysiological activity, DNA damage and antioxidant enzymes in root of Allium cepa model, Sci. Rep., 2020, 10, 1–10 CrossRef PubMed
. - I. Ali, B. Liu, M. A. Farooq, F. Islam, A. Azizullah, C. Yu, W. Su and Y. Gan, Toxicological effects of bisphenol A on growth and antioxidant defense system in Oryza sativa as revealed by ultrastructure analysis, Ecotoxicol. Environ. Saf., 2016, 124, 277–284 CrossRef CAS PubMed
. - D. M. Pazmino, M. Rodríguez-Serrano, Romero-Puertasmc, A. -R. Angustias, L. A. Del Rio and L. M. Sandalio, Differential response of young and adult leaves to herbicide 2, 4-dichlorophenoxyacetic acid in pea plants: role of reactive oxygen species, Plant, Cell Environ., 2011, 34, 1874–1889 CrossRef CAS PubMed
. - K. . O. Erinle, Z. Jiang, B. Ma, J. Li, Y. Chen, K. Ur-Rehman, A. Shahla and Y. Zhang, Exogenous calcium induces tolerance to atrazine stress in Pennisetum seedlings and promotes photosynthetic activity, antioxidant enzymes and psbA gene transcripts, Ecotoxicol. Environ. Saf., 2016, 132, 403–412 CrossRef CAS PubMed
. - H. Chen, Y. Qin, J. Pu, J. Hu and Y. Wen, Phytotoxicity of the chiral herbicide dichlorprop: Cross-talk between nitric oxide, reactive oxygen species and phytohormones, Sci. Total Environ., 2021, 788, 147866 CrossRef CAS PubMed
. - N. Yao and J. T. Greenberg, Arabidopsis accelerated cell death modulates programmed cell death, Plant Cell, 2006, 18, 397–411 CrossRef CAS PubMed
. - P. M. G. Nair and I. M. Chung, Physiological and molecular level effects of silver nanoparticles exposure in rice (Oryza sativa L.) seedlings, Chemosphere, 2014, 112, 105–113 CrossRef CAS PubMed
.
Footnote |
† Electronic supplementary information (ESI) available. See DOI: 10.1039/d1ra09440h |
|
This journal is © The Royal Society of Chemistry 2022 |
Click here to see how this site uses Cookies. View our privacy policy here.