DOI:
10.1039/D1RA09275H
(Paper)
RSC Adv., 2022,
12, 6463-6475
Effective utilization of magnetic nano-coupled cloned β-xylanase in saccharification process
Received
22nd December 2021
, Accepted 16th February 2022
First published on 24th February 2022
Abstract
The β-xylanase gene (DCE06_04615) with 1041 bp cloned from Thermotoga naphthophila was expressed into E. coli BL21 DE3. The cloned β-xylanase was covalently bound to iron oxide magnetic nanoparticles coated with silica utilizing carbodiimide. The size of the immobilized MNPs (50 nm) and their binding with β-xylanase were characterized by Fourier-transform electron microscopy (FTIR) (a change in shift particularly from C–O to C–N) and transmission electron microscopy (TEM) (spherical in shape and 50 nm in diameter). The results showed that enzyme activity (4.5 ± 0.23 U per mL), thermo-stability (90 °C after 4 hours, residual activity of enzyme calculated as 29.89% ± 0.72), pH stability (91% ± 1.91 at pH 7), metal ion stability (57% ± 1.08 increase with Ca2+), reusability (13 times) and storage stability (96 days storage at 4 °C) of the immobilized β-xylanase was effective and superior. The immobilized β-xylanase exhibited maximal enzyme activity at pH 7 and 90 °C. Repeated enzyme assay and saccharification of pretreated rice straw showed that the MNP-enzyme complex exhibited 56% ± 0.76 and 11% ± 0.56 residual activity after 8 times and 13 times repeated usage. The MNP-enzyme complex showed 17.32% and 15.52% saccharification percentage after 1st and 8th time usage respectively. Immobilized β-xylanase exhibited 96% residual activity on 96 days' storage at 4 °C that showed excellent stability.
Introduction
Currently enzymes are mostly being used as biocatalysts in various industries such as the energy, food and drug industries and also have a crucial role in the protection of the environment. Since enzymes reveal numerous important properties such as specificity, selectivity, lack of secondary reactions and low toxicity, their use is considered to be more valuable over traditional chemical procedures.1
Enzymes can find applications in different industrial procedures and fields, such as biomedicine, nanotechnology, biosensors, protein engineering and pharmaceuticals, and the main reason for such a broad application series is the enzyme stability. Free enzymes are generally not stable in the buffer solutions, but after immobilization (covalent attachment, simple adsorption or encapsulation), their stability can improve significantly.2,3 Enzyme immobilization reduces several limitations via improving enzyme stability, specificity or selectivity, and even eliminates enzyme inhibition processes and also improves the controlling nature of the enzymes.4,5
β-Xylanase is important industrial enzyme that has received much attention in recent years because of its wide applications in the wastewater treatment, feed stock improvement, food and beverage industries and conversion of lignocelluloses biomass into bioethanol productions.6,79,80 However, the industrial applications of such enzymes in different fields are mostly limited due to their low operational stability, sensitivity to the environment conditions, short lifetime; high production costs bring about the problems in recovery and reusability.7 β-Xylanase enzyme has many applications in paper and pulp industry, baking and brewing industry and in food and feed industry. It is not easy to produce β-xylanase enzyme at several times, mostly in large-scale experiments like saccharification process etc.
To reduce such a problems, immobilization of enzymes onto different synthetic and natural supports is deliberated to be an effective and impressive approach to improve the stability and recovery of enzyme from the reaction mixture and ease in recycling for reusability.8
Immobilization of various biomolecules (protein, antibodies enzymes etc.) onto insoluble supports like magnetic nanoparticles suggests different benefits that consist of improved stability, easy separation from reaction mixture and prevention of microbial growth.9 In recent years, attention of utilizing nano-sized magnetic particles gain attention in several fields such as environmental and biomedical applications, associated to their size, magnitude, good reusability, low toxicity, large enzyme loading capacity, easy manipulation of surface modification and large surface area.10–12
Fe3O4 magnetic nanoparticles are one of the mostly used magnetic materials because of the super-paramagnetic materials bearing magnetic properties and biocompatibility.13 Use of magnetic nanoparticles (MNPs) is gaining outstanding attention for enzyme immobilization because of the ability of MNPs to carry maximum enzymes due to the accessibility of high surface area and better enzyme stability.14 Such a MNPs used in enzyme and protein immobilization,15 protein purification,16 hyperthermia,17 enhancement of contrast in magnetic resonance imaging18 and drug delivery.19
Whereas, some other fields that obtained benefits from utilization of magnetic nanoparticles are waste water processing20,21 and textile.22 The binding of bio-molecules like proteins, enzymes and antibodies onto magnetic nanoparticles are generally performed through covalent bonding, surface adsorption, inclusion in a gel phase, encapsulation and cross-linking by bi-functional reagents.23–25 A recent method comprising carbodiimide activation has gain popularity because of its high efficiency and simplicity.26
Although the recovery of immobilized enzyme is limited in industrial projects, magnetic nanoparticles have an excellent feature because of their strong magnetic material. Enzymes immobilized on magnetic nanoparticles easily separated from the reaction mixture using a simple magnetic without using expensive liquid chromatography systems, centrifugation, filtrations etc.27
Among bioprocessing of lignocellulosic plant residues, the focus is on using enzymes for hydrolysis and degradation of agro-industrial waste. The conversion of biomass to monosaccharides or other chemicals by saccharification and then after fermentation their conversion to bioethanol have an important role. Reusability of the immobilized MNPs is also a cost effective process to avoid the repeatedly production of enzyme.28–31
Therefore, it is required to utilize suitable support for immobilization of enzymes that can be reused several times. Some methods of enzymes immobilization include the binding of enzyme via covalent bonding using carbodiimide (EDC). A reaction between an amine group on the nanoparticle surface and amid carboxyl group of the enzyme produced the bond to develop immobilization.26 The magnetic core facilitates the selective binding of protein with carbodiimide and easy recovery of magnetic particles from media using magnets and can be repeatedly reused for several times.
The objectives of this work was to immobilize the recombinant purified β-xylanase enzyme from Thermotoga naphthophila onto magnetic nanoparticles (Fe3O4) coated with silica via carbodiimide for recurrence re-use to make the process economical. Furthermore, immobilization of β-xylanase on MNPs was characterized. Effect of temperature, pH and metal ions was extensively examined to achieve the outstanding results. Further, its stability at room temperature as well as at 4 °C was also determined. Additionally, reusability of immobilized MNPs was also determined by performing saccharification process at optimized conditions.
Methodology
Cloning and expression of β-xylanase gene in pET-21a(+)
The cloning of β-xylanase gene (DCE06_04615) into pET-21a(+) and production of recombinant β-xylanase enzyme was carried out using standard procedures as described by Hamid et al.32 Double restricted β-xylanase and plasmid pET-21a(+) with NdeI and HindIII were ligated with T4 DNA ligase enzyme. The ligated product was transformed into the E. coli BL21 (DE3) competent cells. The transformed cells were then transferred to LB agar plates supplemented with ampicillin. The colonies obtained were marked randomly and tested for the presence of ligated product (pET 21a(+)/β-xylanase gene) through colony PCR. Recombinant plasmid was isolated from the positive colonies and double restricted with NdeI and HindIII to further confirm the ligation of β-xylanase gene with plasmid. E. coli BL21 (DE3) bearing β-xylanase (Tnap_0700) gene was cultured in 100 ml LB broth (1.0% tryptone, 0.5% yeast extract and 0.5% NaCl) supplemented with containing ampicillin (100 mg ml−1) in 250 ml flask at 37 °C and 200 rpm. E. coli cells were induced by adding 0.5 mM IPTG in the flask. The cells were then incubated at 37 °C for 4 hours. The contents of the flask were centrifuged at 10
000 rpm for 10 minutes. The supernatant was discarded and cells stored at −20 °C for further usage. Sodium citrate phosphate buffer (50 mM, pH 7.0) was used to suspend the E. coli cells and later treated with 30 cycles of sonication, each cycle with 60% amplitude in a sonication system at 4 °C (Bandelin, Sonoplus). The sonicated product was placed on ice for 30 minutes and then centrifuged at 10
000 rpm for 15 minutes at 4 °C. Finally, β-xylanase enzyme was purified by utilizing heat treatment method and immobilized metal ion affinity chromatography. The purified enzyme thus obtained was utilized to determine the β-xylanase enzyme activity and immobilization of purified β-xylanase with the magnetic nanoparticles (MNPs).
Immobilization of β-xylanase onto magnetic nanoparticles
Magnetic nanoparticles were obtained from Dr Muller, Germany. β-xylanase enzyme was immobilized onto MNPs by following the method of Jordan et al.26 with some modifications. For β-xylanase binding, almost 50 mg magnetic nanoparticles was added into a 5 ml solution containing 8 mg ml−1 1-(3-dimethylaminopropyl)-3ethylcarbodiimide hydrochloride (EDC). The reaction was sonicated for 3 minutes and incubates on ice for 30 minutes. The reaction contents was incubated for 2 hours at 4 °C and followed by sonication for 3 minutes after every 1 hour intervals in a way to assure uniform dispersion. After 2 hours, the reaction mixture was sonicated and then heated for 10 minutes at 30 °C. β-xylanase bound magnetic nanoparticles were removed with the help of strong magnet source. MNPs were washed with distilled water and enzyme activity and stability was determined.
Characterization of recombinant thermostable immobilized β-xylanase enzyme
After enzyme immobilization, determination of enzyme activity and protein were characterized.
The enzyme activity was calculated after xylose production during a reaction of nanoparticles-bound-β-xylanase with xylan from beechwood as a substrate. After binding, 50 μl solution containing β-xylanase bound magnetic nanoparticles was added to 100 μl xylan substrate (citrate phosphate buffer (50 mM), pH 7.5), incubated at 50 °C for 10 minutes. The magnetic nanoparticles were recovered using strong magnet source and 2 ml DNS was added to terminate the process. The reaction test tubes were kept into the boiling water for 5 minutes. The reducing sugar was calculated at 540 nm by spectrophotometer. The controls were also run in the same way by using substrate and magnetic nanoparticles, without addition of enzyme. All reactions were run in triplicate. Magnetic nanoparticles immobilized with β-xylanase enzyme were repeatedly utilized to determine its reusability potential. Activity of the β-xylanase enzyme was observed up to 13 times using xylan as substrate.
The effect of temperature on immobilized β-xylanase enzyme was determined by incubating the enzyme at 40, 50, 60, 70, 80 and 90 °C for 3 hours. The stability of the enzyme was determined after performing the enzyme assay. The effect of initial pH on immobilized β-xylanase enzyme was determined by incubating the enzyme at pH values of 4.0, 5.0, 6.0, 7.0, 8.0 and 9.0 for 3 hours. The stability of the enzyme was determined by enzyme assay. The effect of different metal ions on immobilized β-xylanase enzyme was determined by incubating the enzyme with different metal ions (Mg2+, Cu2+, K1+, Ca2+, Na1+, Mn2+, Ni2+) at different concentrations ranging from 1–10 mM for 1–3 hours. The stability of the enzyme was determined using enzyme assay.
Characterization of magnetic nanoparticles
Transmission electron microscopy (TEM). The morphology and size of the magnetic nanoparticles were evaluated using TEM (JEOL JEM-1010). In this analysis, the AEI copper grids having 900 mesh were first coated with a thin layer of 25–30 nm carbon film as a support media. A suspension of magnetic nanoparticles was prepared in ethanol after sonication for five minutes. Prepared sample was loaded on copper grid and air dried and then examined under TEM machine that was operating at 80 kV. Electron micrographs were captured and labeled.
Fourier transform infrared spectroscopy (FTIR). Based on basic mechanism of Fourier transform infrared spectroscopy (FTIR) that involve absorbance of different frequencies for the bonds between various elements, it utilizes a device known as interferometer (IRAffinity-1, SHIMADZU) to identify samples after generating an optical signal with all IR frequencies encoded and calculated the values. The signal was decoded using Fourier transform, a map of the spectral information was produced by a computer-generated process. The graph was the spectrum and was identified by different reference database houses. Various immobilized MNPs with β-xylanase, MNPs alone and β-xylanase were analyzed by FTIR.
Pretreatment of rice straw. The pretreatment of rice straw was performed by following the method given by Hoşgün et al.33 with some modifications. Pretreatment of rice straw was carried out by using 10% (w/v) NaOH at 30 °C for 72 hours. The rice straw was weighed (about 2 g) and placed in an Erlenmeyer flask (250 ml) dipped in a solution of 10% (w/v) NaOH and incubated at 30 °C for 72 hours. After the incubation period, biomass was filtered, washed with double distilled water and allowed to dry at room temperature for two days.
Reusability of immobilized magnetic nanoparticles in saccharification process. The procedure of saccharification was performed in a 250 ml flask. Immobilized β-xylanase magnetic nanoparticles were added along with pretreated plant biomass (rice straw) and incubated at 50 °C. Experimental flasks were kept into a shaking incubator at 150 rpm for 6 hours. β-xylanase units immobilized onto magnetic nanoparticles were used to hydrolyze 0.05 g of pretreated rice straw biomass. To avoid contamination of microorganisms, tetracycline (10 μg/ml) was added with immobilized β-xylanase magnetic nanoparticles. The production of reducing sugars in the saccharification procedure was analyzed by method of Miller.34 After the process, magnetic nanoparticles were removed with the help of strong magnet. β-xylanase-MNPs were used again in saccharification process with new pre-treated biomass. The β-xylanase-MNPs were used repeatedly in saccharification process. The process was stopped until enzyme action was significantly reduced.
Results
Expression of recombinant enzyme
The recombinant β-xylanase enzyme activity was calculated in the intracellular fraction, β-xylanase activity was estimated to be 4.5 ± 0.23 U per ml. Total proteins were determined and estimated to be 4.25 ± 0.27 mg ml−1.
Immobilization of β-xylanase onto magnetic nanoparticles
β-xylanase enzyme was immobilized onto magnetic nanoparticles via development of covalent bond between magnetic nanoparticles and enzyme via 1-(3-dimethylaminopropyl)-3ethylcarbodiimide hydrochloride (EDC). The functional groups present in primary structure of the enzymes bound to magnetic nanoparticles as these functional groups have no effective impact on the catalytic activity. The binding mechanism of enzyme to magnetic nanoparticles carried out on standard conditions that do not cause any reduction in the enzyme activity, and the active site of the enzyme remained stable. However, these magnetic nanoparticles showed an extra advantage as these particles can be retrieved easily by external magnetic source.
Characterization of immobilized β-xylanase
To describe the properties of the immobilized β-xylanase enzyme activity, the enzyme activity as well as reusability assay was performed repeatedly using standard enzyme assay.
Determination of enzyme activity and protein
Enzyme activity of immobilized β-xylanase enzyme was determined after calculating the reducing sugar produced by action of immobilized enzyme with xylan (beechwood) substrate as mentioned in methodology section. The enzyme activity of recombinant β-xylanase immobilized on MNPs was calculated to be 6.5 ± 0.34 U per ml. Total proteins were determined and estimated to be 5.25 ± 0.18 mg ml−1. All assays were performed in triplicate.
Reusability assay
Reusability feature of the immobilized β-xylanase enzyme was one of the important properties of this complex that was determined in this work. The immobilized β-xylanase enzyme was used in the enzymatic assay for 13 consecutive times. After 5 times usage, the enzyme-MNPs activity was retained by 89% ± 0.76. However, the enzyme-MNPs complex retained 70% ± 0.76 residual activity after 8 re-uses. Further repeated enzyme assays for 13th time resulted in the drop of residual activity to 55% ± 0.56 (Fig. 1a and b).
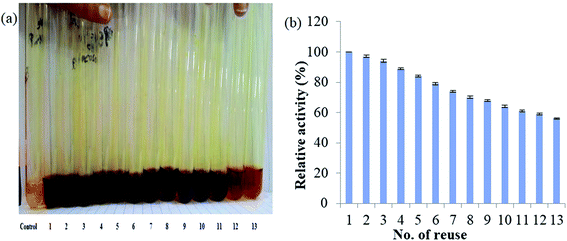 |
| Fig. 1 (a) Repeated assays to determine the activity of immobilized β-xylanase performed at 50 °C; (b) residual activity of immobilized β-xylanase enzyme after repeated enzyme assays. | |
Effect of thermostability on immobilized β-xylanase
To determine the thermostability, the immobilized magnetic nanoparticle (with β-xylanase enzyme) was incubated at different temperatures ranging from 50–90 °C. After 1 hour, the immobilized MNPs showed 99.5% ± 0.75, 98.7% ± 0.63 and 98.1% ± 0.57 after 50 °C, 60 °C and 70 °C respectively. The immobilized MNPs showed 80% ± 0.91 residual activity at 80 °C after 4 hours of incubation (Fig. 2A). Reasonably significant residual activity was demonstrated by immobilized β-xylanase 3 hours at 60 °C and 70 °C, resulted in 96% ± 0.58 and 95% ± 0.65 respectively. At 90 °C, the immobilized β-xylanase activity was reduced to 64.89% ± 0.56 after 2 hours of incubation. Similarly, at 90 °C after 4 hours of incubation, the immobilized β-xylanase enzyme activity was reduced to 29.89% ± 0.72. No significant reduction in immobilized β-xylanase activity was observed at 50 °C and 60 °C and 70 °C after 3 hours of incubation (Fig. 2A).
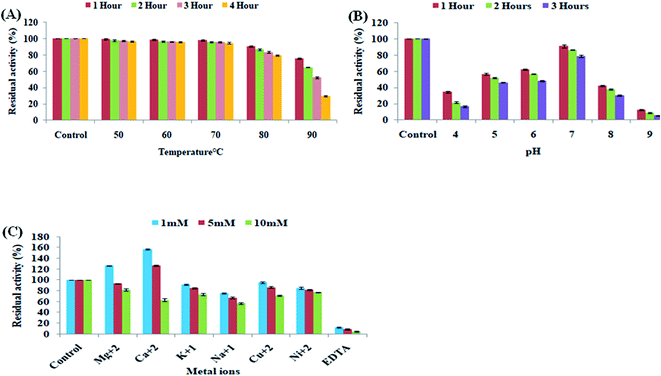 |
| Fig. 2 Characterization (A). Determination of thermostability of immobilized MNPs (50 °C to 90 °C from 1–4 hours) (B). Determination of pH stability of immobilized β-xylanase enzyme (pH values (4–9) after 1, 2 and 3 hours). (C) Effect of different concentrations (1–10 mM) of metal ions and EDTA on immobilized β-xylanase enzyme (1–10 mM for 1–3 hours). | |
Effect of pH stability on immobilized β-xylanase
To determine the pH stability, the immobilized magnetic nanoparticle (with β-xylanase enzyme) was incubated at different pH value ranging from 4.0–9.0. At pH 4, the immobilized MNPs were reduced to 34% ± 1.24, 21% ± 1.10 and 16% ± 1.01 after 1, 2 and 3 hours. Reasonable residual activity of immobilized β-xylanase was observed at pH values 5, 6 and 8 (Fig. 2B). The maximum residual activity of immobilized β-xylanase was determined to be 91% ± 1.91 at pH 7 after 1 hour of incubation. Similarly, high residual activity of 86% ± 0.48 and 78% ± 1.48 was observed after 2 and 3 hours respectively. At pH 6 the residual activity of immobilized MNPs was reduced to 56% ± 0.65 after 2 hours of incubation. Similarly, at pH 8 after 3 hours of incubation the immobilized MNPs residual activity was reduced to 30% ± 0.67. However, at pH 9, after 2 and 3 hours of incubation, the residual activity of immobilized β-xylanase was reduced to 8.9% ± 0.55 and 5.5% ± 0.38 respectively.
Effect of different metal ions on immobilized β-xylanase
To determine the EDTA and metal ions effect, the immobilized β-xylanase with MNPs was incubated with different metal ions (Mg2+, Cu2+, K1+, Ca2+, Na1+, Mn2+, Ni2+) at different concentrations ranging from 1–10 mM. The immobilized β-xylanase enzyme showed 57% ± 1.08 and 26% ± 1.02 increase in residual activity with Ca2+ and Mg2+ ions when incubated at 1 mM concentration but dropped to 62% ± 2.38 and 81% ± 1.98 at 10 mM (Fig. 2C). The residual activity of immobilized MNPs was calculated to be 91% ± 1.34, 75% ± 1.12, 95% ± 1.01, 85% ± 2.31 and 11% ± 0.95 when incubated with 1 mM K1+, Na1+, Cu2+, Ni2+ and EDTA respectively. At higher concentration (10 mM) of Mg2+, Cu2+, K1+, Ca2+, Na1+,and Ni2+, a drop in residual activity was observed that reached to 81% ± 1.98, 62 ± 1.31 73% ± 2.11, 57% ± 2.31, 71% ± 0.851 and 76% ± 0.78respectively (Fig. 2C). The effect of EDTA on Immobilized MNPs was very detrimental and residual activity was reduced to 11% ± 0.951, 8% ± 1.31 and 4% ± 0.85 at 1 mM, 5 mM and 10 mM EDTA respectively.
Determination of binding of β-xylanase on magnetic nanoparticles by TEM analysis
The binding of the recombinant β-xylanase enzyme onto magnetic nanoparticles was analyzed by transmission electron microscope. Transmission electron microscopy (TEM) showed the dimensions of β-xylanase magnetic nanoparticles. MNPs demonstrated spherical in shape with diameter of approximately 50 nm. The comparison of TEM images of MNPs at two different scales represents the accurate magnification and size of these particles. However, some of the agglutination of these MNPs is because of their magnetic nature. The small size of magnetic nanoparticles provides more surface to volume ratio and effective enzyme immobilization (Fig. 3).
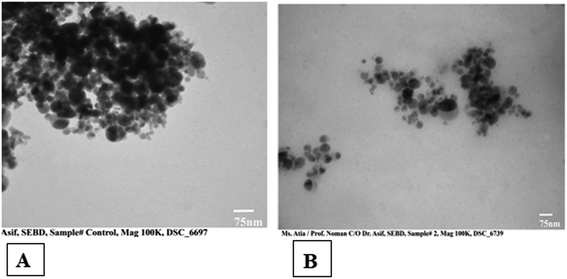 |
| Fig. 3 TEM Analysis (A). Pure MNPs (B) MNPs-immobilized with β-xylanase enzyme (25–30 nm carbon film, 80 kV). | |
Determination of binding of β-xylanase on magnetic nanoparticles by FTIR analysis
β-Xylanase binding on top of CC-Fe3O4@SiO2 and presence of surface functional groups on magnetic nanoparticles were detected by FTIR spectroscopy. The FTIR spectra of the (Fig. 4A) MNPs (Fe3O4@SiO2) (Fig. 4B) β-xylanase (Fig. 4C) and immobilized β-xylanase on MPNs (β-xylanase-MNPs) are shown in Fig. 6. The absorption bands nearly 1088 cm−1 and 808 cm−1 were assigned to the symmetric stretching of Si–O–Si and Si–OH, respectively, suggesting the existence of silica shell on MNPs. However, the FTIR spectrum of the β-xylanase and β-xylanase-MNPs (Fig. 4B and C) demonstrated characteristic bands at 3346(O–H), 3288(N–H), 2920 (C–H aliphatic band) 1630 (C
O) and 1585(C–N) cm−1. The main characteristic frequencies associated with the functional groups (–OH, –NH, C–H (aliphatic), C
O, C–N etc.) present in β-xylanase enzyme and the linker 1-ethyl-3-(3-dimethylaminopropyl) carbodiimide (EDC) are also reflected in the magnetic nanoparticles having immobilized β-xylanase enzyme. The appearance of absorbance frequencies associated with the main functional groups of β-xylanase enzyme in β-xylanase bound magnetic nanoparticles confirms the attachment of the enzyme on magnetic nanoparticles. The primary structure analysis of β-xylanase proteins reveals the amino acid configuration of various proteins with five predominant amino acids as threonine (9.50%), preceded by glycine (8.80%), alanine (8.20%), serine (7.90%) and aspartic acid (6.54%). The specific frequencies associated with these segments are mostly characterized by –N–H, C
O, C–O and C–H (aliphatic) groups. A change in the frequency on the immobilized β-xylanase enzyme is mostly due to the formation of the amide bond. Use of EDC suggests that covalent bond was generated between a carboxyl group on the β-xylanase enzyme and an amine group of the linker EDC on the magnetic nanoparticles surface (specifically, C–O to C–N). The pure magnetic nanoparticles also showed some particular IR frequencies. These peaks are not found in immobilized magnetic nanoparticles, showing the formation of the amide bond.
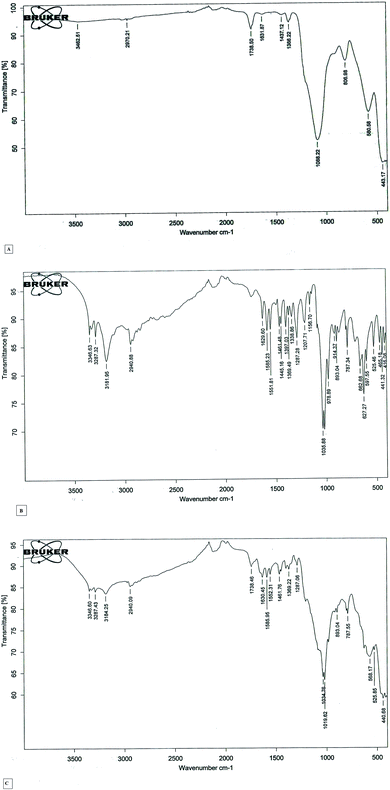 |
| Fig. 4 (A) FTIR spectra of pure magnetic nanoparticles (B) FTIR spectra of free β-xylanase enzyme (C) FTIR spectra of immobilized magnetic nanoparticles with β-xylanase enzyme (50–100% transmittance, 3500–500 wavenumber cm−1), comparison of FTIR spectra of these three samples showing bond shift particularly from C–O to C–N. | |
SEM analysis of rice straw
The rice straw was pre-treated as mentioned in methodology section. Scanning electron microscopy was used to analyze the structures of pre-treated rice straw. The electron micrograph of native sample revealed the woody, regular, compact and smooth surface, signifying the highly well-arranged surface structure, whereas the pretreated sample showed an irregular and uneven structure and exhibited loosened inner zones of hemicellulose and cellulose content (Fig. 5A and B). Pretreatment method increased the surface area of rice straw to provide enzyme better accessibility for hydrolysis of cellulytic contents of plant biomass.
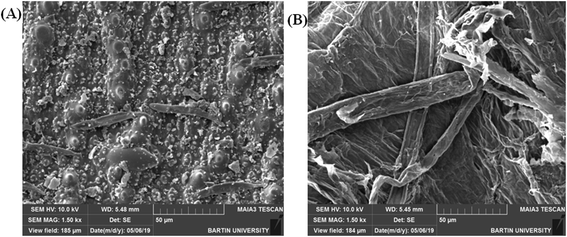 |
| Fig. 5 SEM analysis of (A) control (without pre-treated) (SEM HV: 10.0 KV, SEM MAG: 1.50 KX, view field: 185 μm, WD: 5.48 mm, diameter 50 μm) (B) pre-treated samples of rice straw (SEM HV: 10.0 KV, SEM MAG: 1.50 KX, view field: 184 μm, WD: 5.45 mm, diameter 50 μm). | |
Reusability of immobilized magnetic nanoparticles in saccharification process
Reusability of the enzymatic activity of β-xylanase immobilized with magnetic nanoparticle was determined to estimate the hydrolysis process in the pre-treated rice straw. For this purpose, weighed rice straw was incubated with immobilized magnetic nanoparticles along with Tris-Cl buffer (pH 8). Tetracycline (10 μg μL−1) was also added in order to stop any microbial contamination. After the hydrolysis at optimized conditions, reducing sugar was calculated by DNS method. The reusability of the immobilized magnetic nanoparticles (with β-xylanase) was evaluated. It was found that immobilized magnetic nanoparticles can be reused for 8 times for saccharification process (Fig. 6). The residual activity up to 78% ± 0.98 was observed after 4 times of usage of β-xylanase immobilized MNPs. However, 56% ± 1.06 residual activity was retained after 5th time usage and residual activity was dropped to 10% ± 0.38 after 8th usage. The saccharification potential of immobilized MNPs was calculated to be 17.32% after 5th time of usage. However, a sight change in the saccharification potential was might be because of washing of the immobilized MNPs to start up the new saccharification reaction. Table 1 shows the residual activity and saccharification potential of β-xylanase enzyme immobilized onto MNPs.
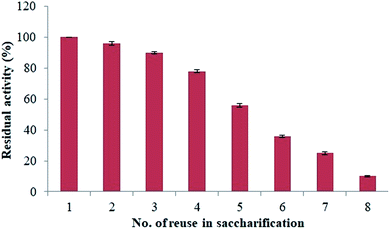 |
| Fig. 6 Determination of the reusability of catalytic activity of β-xylanase immobilized with magnetic nanoparticles in saccharification of pretreated rice straw (at 50 °C, 150 rpm for 6 hours). | |
Table 1 Saccharification potential of immobilized MNPs
Resuse of immobilized β-xylanase in saccharification |
Residual activity (%) |
Saccharification (%) |
Reducing sugar (mg ml−1) |
1st cycle |
100 |
17.32 |
3.85 |
2nd cycle |
96 |
17.14 |
3.73 |
3rd cycle |
90 |
16.87 |
3.65 |
4th cycle |
78 |
17.06 |
3.77 |
5th cycle |
56 |
16.61 |
3.59 |
6th cycle |
36 |
16.13 |
3.62 |
7th cycle |
27 |
16.02 |
3.47 |
8th cycle |
11 |
15.52 |
3.14 |
Discussion
In this research work, recombinant thermostable β-xylanase enzyme was cloned from Thermotoga naphthophila and expressed in E. coli BL21 (DE3) after IPTG induction.21 After the successful expression of recombinant β-xylanase enzyme, further purification was carried out by two different strategies i.e. heat treatment method and immobilized metal ion affinity chromatography. After purification step, the β-xylanase was covalently bound to magnetic nanoparticles by carbodiimide (EDC) activation. The activation was because of the formation of an amide bond resulting from the reaction between the carboxyl group on the β-xylanase enzyme and an amine group on the nanoparticle surface (specifically, the C–O to C–N conversion). After immobilization of enzyme, catalytic activity was analyzed by DNS method. Further, reusability of the immobilized enzyme to determine saccharification potential of pretreated rice straw was also determined.
Thermostable enzymes have an advantage as they are being used as catalyst in different industrial processes, as high temperatures often promote better enzyme penetration and cell-wall disorganisation of the raw materials. However, enzyme production is costly and at certain temperature enzyme activity was also reduced. These properties have fascinated scientists to discover some support materials or carriers for immobilization of enzymes.35–38 In various industrial processes, enzymes are mostly used in bulk and sometimes because of high cost of enzyme production strategy it's not possible to produce enzymes in bulk. To overcome this problem, enzymes are immobilized on solid supports to increase reusability and stability. Now-a-days, magnetic nanoparticles (MNPs) prepared by iron oxides, gain most importance because of the different characteristics like small size, biocompatibility and super paramagnetism.39
Previously, several enzymes have been shown to immobilize onto MNPs using EDC. EDC is being used to develop the covalent bond during immobilization of antimicrobial peptides on biomaterial surfaces.40 Cellulase enzyme was also immobilized onto MNPs using the carbodiimide method and it showed the improvement in enzyme activity and stability.26 Recently, production of recombinant carbonic anhydrase enzyme and its immobilization onto MNPs that in decline of cost of enzyme production was demonstrated.41 In another study, the immobilization of α-amylase was done onto MNPs via covalent attachment using carbodiimide (CDI) molecules.42
In the present study, covalent binding of the β-xylanase with MNPs showed 13 consecutive times repeated use of immobilized enzyme, which can significantly reduce the cost of the industrial processing. The residual activity of the immobilized β-xylanase was 56% ± 0.56 of the initial enzyme activity even after 13 times re-usage (Fig. 1a and b). Our results were similar to the findings of a previous study,43 which had revealed the immobilization of Mn-SOD onto supermagnetic nanoparticles and repetitively used for 10th time with a reduction in Superoxide dismutase enzyme activity. The loss of immobilized enzyme activity possibly because of leakage of the enzyme from the carriers and also because of the conformational changes in the enzyme structure. The reduction of enzyme activity to 56% ± 0.56 might be due to the failure of the enzyme activity because of aggregation and loss of some magnetic nanoparticles during the washing process, recovery and protein denaturation.44 In another study, the relative activity of laccase enzyme that was immobilized on MNPs was dropped to 40% after the 8th cycle of reusage.45 Various strategies were employed for the immobilization of different enzymes on MNPs. In recent studies, Fe3O4 MNPs were used for immobilization of cellulose chitosinase and β-glucosidase enzymes through covalent bonding utilizing EDC, APTES, gluteraldehyde and PEG46–48 (Table 2). In another strategy, chitosan coated MNPs were used for immobilization of xylanase and laccase enzymes via gluteraldehyde.49,50 APTES method was used to immobilize cellulose enzyme on the Cu-aminofunctionlized MNPs.47 The immobilized enzyme after 5th cycle of reusability retained 73% residual activity. Table 2 shows the summary of several enzymes immobilized onto MNPs.
Table 2 Different enzyme immobilization onto MNPs
S. no. |
Enzyme |
Nanoparticle type |
Reagent used for immobilization |
References |
1 |
Cloned β-xylanase |
Fe3O4 MNPs coated with SiO2 |
EDC (carbodiimide) |
This study |
2 |
Xylanase |
Chitosan-coated magnetic particles |
Gluteraldehyde |
49 |
3 |
Cellulase |
Fe3O4 MNPs |
EDC (carbodiimide) |
32 |
4 |
Chitosinase |
Fe3O4 MNPs |
APTES (3-aminopropyltriethoxysilane) |
69 |
5 |
Lacasse |
Chitosan(C)-MNPs |
Gluteraldehyde |
50 |
6 |
Cellulase |
Cu-aminofunctionlized MNPs |
APTES (3-aminopropyltriethoxysilane) |
47 |
7 |
β-Glucosidase |
Fe3O4MNPs |
Gluteraldehyde |
77 |
8 |
Cellulase |
Fe2O3MNPs |
PEG (polyethylene glycol) |
48 |
This study showed that immobilized β-xylanase enzyme exhibited the 96% of residual activity after 30 days of storage at 4 °C, however retained 90% residual activity when stored at room temperature for 30 days that indicate excellent stability and durability of enzyme-MNPs complex. This is due to the covalent bonding that attaches enzymes onto the magnetic nanoparticles was able to provide the protection to the enzymes from denaturalization.51 The immobilization of pectinase on chitosan MNPs using dextran as a polyaldehyde cross-linker. They showed that immobilized enzyme retained up to 89% of relative activity after storage of 15th days.52 Laccase enzyme concludes that it maintained 70% of its initial activity at 25 °C up to 12th day from the first day of storage.53 Many recent studies has showed that α-amylase and xylanase enzymes remained stable and retained 65%, 93% and 60% residual activity after immobilization with MNPs for up to 60 days at 4 °C.54–56 Similarly, alcohol dehydrogenase and pectinase enzymes showed 75% and 87% residual activities when stored at 4 °C for 21 days and 30 days respectively.57,58 However, one study demonstrated the stability of peroxidase enzyme at 25 °C for 60 days and hydrolase 76% for 60 days.59,60 Table 3 show the summary of stability of various enzymes immobilized with MNPs at different temperatures and time duration.
Table 3 Storage stability of various immobilized enzymes
S. no. |
Enzyme-nanoparticle |
Storage |
Residual activity |
References |
1 |
Cloned β-xylanase |
90 days (4 °C) |
96% |
This study |
15 days (room temperature) |
73% |
2 |
Pectinase |
15 days |
89% |
52 |
3 |
Laccase |
12 days (25 °C) |
70% |
53 |
4 |
α-Amylase |
Upto 8 weeks (4 °C) |
65% |
54 |
5 |
Xylanase |
Upto 6 weeks (4 °C) |
93% |
55 |
6 |
Hydrolase |
60 days (4 °C) |
76% |
60 |
7 |
Peroxidase |
60 days (25 °C) |
77.2% |
59 |
8 |
α-Amylase |
Upto 6 weeks (4 °C) |
60% |
56 |
9 |
Alcohol dehydrogenase |
21 days (4 °C) |
75% |
57 |
10 |
Pectinase |
30 days (4 °C) |
87% |
58 |
In the present study, a very important characteristic feature of immobilized β-xylanase with MNPs was thermostability at high temperature ranging from 50–90 °C. High thermostability of the enzyme was observed at temperature 80 °C and 90 °C. Enhancement in the thermostability of the immobilized enzyme was due to the reason that magnetic affinity of the MNPs provides proper protection to slow down the heat transfer of the immobilized β-xylanase-MNPs structure at high temperature.61 80% ± 0.91 residual activity was observed when enzyme was incubated at 80 °C for 4 hours (Fig. 2A). The development of many covalent bonds between the support and the enzyme reduces conformational flexibility, thermal vibrations, and enzyme mobility, while also preventing the unfolding of the enzyme protein.62 This is because the three-dimensional structure of the protein was protected from thermal denaturation by the restriction of the enzyme mobility upon immobilization. The loss in enzyme activity may be because of different factors like the denaturation of protein due to its repeated use at high temperature or as a result of the inhibition of enzyme activity by end product of the reaction.63,64 The insignificant loss of enzyme activity was because of the leakage of very small amount of enzyme during its consecutive usage. The laccase enzyme immobilized onto magnetic β-cyclodextrin-modified chitosan maintained 70% of its initial activity at the temperature 55 °C.53 In another study, the tri-enzyme co-immobilized MNPs demonstrate the thermostability of enzyme for 4 hours at 60 °C.65
Another unique property of this complex is the stability in wide range of pH (4.0–9.0). pH of the solution play an important role in the enzyme stability. Immobilized β-xylanase enzyme was less stable at pH 4.0, and 5.0 moderately stable at 6.0, 8.0 and significantly active at 7.0 pH (Fig. 2B). Immobilized β-xylanase enzyme showed the stability range from 6.0 to 8.0 pH. The covalent attachment of the β-xylanase enzyme with the MNPs reduces the conformational freedom of the enzyme and may be responsible for the increased stability profile.66 The pH stability could be associated to diffusion constraints or the secondary interaction between the immobilized enzyme molecules to the carriers.67 Another study described the pH stability for immobilized tyrosinase pH 7.68 Another researcher also described the tolerance range of pH of 5–10 of α-amylase produced from Thermomyces dupontii.69
Metal ions effect on immobilized β-xylanase, in this study, showed that the enzyme have much more stability in the presence of Ca2+ and Mg2+. This stability was because of the activation of Ca2+ and Mg2+ ions as cofactor for the immobilized β-xylanase enzyme (Fig. 2C). Mohammad et al. also reported the immobilization of amylase onto MNPs and the enhancement in the stability of the immobilized enzyme in the presence of metal ions.70 Xylanase from Thermomyces lanuginosus was covalently immobilized and showed the stability in the presence of Ca2+.71
Transmission electron microscopy (TEM) analysis showed the difference between magnetic nanoparticles without immobilization and after immobilization with β-xylanase enzyme. MNPs are of spherical in shape and diameter of around 50 nm (Fig. 3). In a previous study, the TEM analysis after covalent binding of β-xylanase with functional magnetic nanoparticles.72 The immobilization of cellulase enzyme onto MNPs enables the enzyme to be re-used and further characterized by TEM analysis.73 Tyrosinase enzyme revealed a semi-spherical shape with an average size of 17 nm.68
FTIR analysis confirmed enzyme binding onto MNPs. As the characteristic IR frequencies of the pure β-xylanase enzyme was also present in the FTIR analysis of immobilized MNPs. The FTIR spectra for immobilized MNPs showed the positive immobilization of β-xylanase enzyme onto MNPs (Fig. 4A–C). Results from infrared (IR) spectroscopy revealed a high affinity for β-sheet formation in the tertiary structure content of enzyme molecules. The analysis of primary structure of β-xylanase enzyme revealed the configuration of different amino acids. These amino acids have particular functional groups like (–OH, –NH, C–H (aliphatic), C
O, C–N etc.) present in β-xylanase enzyme and in the EDC. The characteristic bands at 3346(O–H), 3288(N–H), 2920 (C–H aliphatic band) 1630 (C
O) and 1585(C–N) cm−1 represents these functional groups of amino acids in the spectra of immobilized MNPs with β-xylanase enzyme leads to the positive binding of β-xylanase enzyme onto MNPs.74 The amide bond developed via the reaction of carboxyl group of enzyme with the amine group of MNPs.64 These results are similar to a study33 that had immobilized cellulase enzyme complex to MNPs and analyzed after FTIR spectra. Cellulase enzyme coated on MNPs characterized by FTIR.75 Cellulase and β-glucosidase enzymes had covalently bounded on magnetic nanoparticles to determine the stability and activity.76–78
In this research work immobilized β-xylanase in hydrolysis of pretreated rice straw showed the reusability for 8th times (Fig. 6 and Table 1) and results were better from the previous study26 which showed reused immobilized cellulase for six times, after that residual activity start to decrease.
Conclusion
In conclusion, we presented practical, easy and cost effective approach to immobilize β-xylanase enzyme onto magnetic nanoparticles using carbodiimide (EDC). The results proved the stability in pH, thermostability, metal ions, reusability and storage stability of enzyme. It is recommended that β-xylanase immobilized on MNPs by covalent binding is appropriate for practical application in pharmaceutical, chemical and food industries. Determination of successful binding of β-xylanase onto magnetic nanoparticles (MNPs) and its excellent characteristics in this research work also proves to be beneficial in the bioethanol industry. Further studies could also provide benefit after analysis of superparamagnetism and effects of binding on saturation magnetization.
Funding
This research was funded by the Higher Education Commission of Pakistan for Biofuel project no. 5535 and the Pak-Turk Researchers Mobility Program.
Author contributions
Attia Hamid: original manuscript writing, conceptualization. Asma Zafar: investigation. Iram Liaqat: formal analysis. Muhammad Sohail Afzal: validation. Liangcai Peng: resources (Provision of reagent). Muhammad Khawar Rauf: methodology, software analysis. Muhammad Nauman Aftab: supervision (writing – reviewing and editing), project administration. Asad-ur-Rehman: visualization. Sikander Ali: formal analysis. Ikram ul Haq: resources (provision of instrumentation).
Conflicts of interest
The authors declare that they have no conflict of interest.
Acknowledgements
Sincere gratitude is expressed to the Higher Education Commission of Pakistan (Project No 5535) and the Pak-Turk Researchers Mobility Program funded by Higher Education Commission Pakistan and Council of Higher Education Turkey.
References
- C. H. Goh, P. W. S. Heng and L. W. Chan, Alginates as a useful natural polymer for microencapsulation and therapeutic applications, Carbohydr. Polym., 2012, 88, 1–2, DOI:10.1016/j.carbpol.2011.11.012.
- M. Primožič, G. Kravanja, Z. Knez, A. Crnjac and M. Leitgeb, Immobilized laccase in the form of (magnetic) cross-linked enzyme aggregates for sustainable diclofenac (bio) degradation, J. Cleaner Prod., 2020, 275, 124121, DOI:10.1016/j.jclepro.2020.124121.
- G. H. Podrepšek, Z. Knez and M. Leitgeb, Activation of cellulase cross-linked enzyme aggregates (CLEAs) in scCO2, J. Supercrit. Fluids, 2019, 154, 104629, DOI:10.1016/j.supflu.2019.104629.
- R. A. Wahab, N. Elias, F. Abdullah and S. K. Ghoshal, On the taught new tricks of enzymes immobilization: An all-inclusive overview, React. Funct. Polym., 2020, 152, 104613, DOI:10.1016/j.reactfunctpolym.2020.104613.
- K. Vasić, Z. Knez and M. Leitgeb, Immobilization of alcohol dehydrogenase from Saccharomyces cerevisiae onto carboxymethyl dextran-coated magnetic nanoparticles: a novel route for biocatalyst improvement via epoxy activation., Sci. Rep., 2020, 10(1), 1–17 CrossRef PubMed.
- A. Manimaran, K. S. Kumar, K. Permaul and S. Singh, Hyper production of cellulase-free xylanase by Thermomyces lanuginosus SSBP on bagasse pulp and its application in biobleaching, Appl. Microbiol. Biotechnol., 2009, 81, 887–893, DOI:10.1007/s00253-008-1693-x.
- M. Kapoor and R. C. Kuhad, Immobilization of xylanase from Bacillus pumilus strain MK001 and its application in production of xylo-oligosaccharides, Appl. Biochem. Biotechnol., 2007, 142, 125–138, DOI:10.1007/s12010-007-0013-8.
- D. A. Cowan and R. Fernandez-Lafuente, Enhancing the functional properties of thermophilic enzymes by chemical modification and immobilization, Enzyme Microb. Technol., 2011, 49, 326–346, DOI:10.1016/j.enzmictec.2011.06.023.
- U. T. Bornscheuer, Immobilizing enzymes: how to create more suitable biocatalysts, Angew. Chem., Int. Ed., 2003, 42, 3336–3337, DOI:10.1002/anie.200301664.
- T. Zhang, X. Zhang, X. Yan, L. Kong, G. Zhang, H. Liu, J. Qiu and K. L. Yeung, Synthesis of Fe3O4@ZIF-8 magnetic core–shell microspheres and their potential application in a capillary microreactor, Chem. Eng. J., 2013, 228, 398–404, DOI:10.1016/j.cej.2013.05.020.
- C. Cui, Y. Bai, M. Jiang, S. Hu, S. Li and Q. Zhai, Well-defined bioarchitecture for immobilization of chloroperoxidase on magnetic nanoparticles and its application in dye decolorization, Chem. Eng. J., 2015, 259, 640–646, DOI:10.1016/j.cej.2014.08.074.
- M. Bilal, Y. Zhao, T. Rasheed and H. M. Iqbal, Magnetic nanoparticles as versatile carriers for enzymes immobilization: A review, Int. J. Biol. Macromol., 2018, 120, 2530–2544, DOI:10.1016/j.ijbiomac.2018.09.025.
- S. H. Huang, M. H. Liao and D. H. Chen, Direct binding and characterization of lipase onto magnetic nanoparticles, Biotechnol. Prog., 2003, 19, 1095–1100, DOI:10.1021/bp025587v.
- S. S. Patil and V. K. Rathod, Combined effect of enzyme co-immobilized magnetic nanoparticles (MNPs) and ultrasound for effective extraction and purification of curcuminoids from Curcuma longa, Ind. Crops Prod., 2022, 177, 114385 CrossRef.
- A. K. Johnson, A. M. Zawadzka, L. A. Deobald, R. L. Crawford and A. J. Paszczynski, Novel method for immobilization of enzymes to magnetic nanoparticles, J. Nanopart. Res., 2008, 10, 1009–1025, DOI:10.1007/s11051-007-9332-5.
- H. P. Khng, D. Cunliffe, S. Davies, N. A. Turner and E. N. Vulfson, The synthesis of sub-micron magnetic particles and their use for preparative purification of proteins, Biotechnol. Bioeng., 1998, 60, 419–424, DOI:10.1002/(SICI)1097-0290(19981120)60:4<419::AID-BIT3>3.0.CO;2-P.
- R. H. Hilger and W. A. Kaiser, Use of magnetic nanoparticle heating in the treatment of breast cancer. InIEE Proceedings, J. Nanobiotechnol., 2005, 152, 33–39, DOI:10.1049/ip-nbt:20055018.
- A. Ito, M. Shinkai, H. Honda and T. Kobayashi, Medical application of functionalized magneticnanoparticles, J. Biosci. Bioeng., 2005, 100, 1, DOI:10.1263/jbb.100.1.
- D. Demir, D. Güreş, T. Tecim, R. Genç and N. Bölgen, Magnetic nanoparticle-loaded electrospun poly (ε-caprolactone) nanofibers for drug delivery applications, Appl. Nanosci., 2018, 6, 1461–1469, DOI:10.1007/s13204-018-0830-9.
- M. Baalousha, A. Manciulea, S. Cumberland, K. Kendall and J. R. Lead, Aggregation and surface properties of iron oxide nanoparticles: influence of pH and natural organic matter, Environ. Toxicol. Chem., 2008, 9, 1875–1882, DOI:10.1897/07-559.1.
- S. H. Suganthi, K. V. Swathi, R. Biswas, S. Basker and K. Ramani, Co-immobilization of multiple enzymes onto surface-functionalized magnetic nanoparticle for the simultaneous hydrolysis of multiple substrates containing industrial wastes, Appl. Nanosci., 2019, 9, 1439–1457, DOI:10.1007/s13204-019-01125-y.
- P. Babinec and O. Jirsak, Microwave absorbing nonwoven textile from electrospun magnetically responsive nanofibres, Optoelectron. Adv. Mater., Rapid Commun., 2008, 2, 474–477 CAS.
- K. P. García, K. Zarschler, L. Barbaro, J. A. Barreto, W. O'Malley, L. Spiccia, H. Stephan and B. Graham, Zwitterionic-coated “stealth” nanoparticles for biomedical applications: recent advances in countering biomolecular corona formation and uptake by the mononuclear phagocyte system, Small, 2014, 10, 2516–2529, DOI:10.1002/smll.201303540.
- M. Mylkie, P. Nowak, P. Rybczynski and M. Ziegler-Borowska, Polymer-Coated Magnetite Nanoparticles for Protein Immobilization, Materials, 2021, 248, 14, DOI:10.3390/ma14020248.
- O. M. Darwesh, S. S. Ali, I. A. Matter, T. Elsamahy and Y. A. Mahmoud. Chapter Twenty – Enzymes immobilization onto magnetic nanoparticles to improve industrial and environmental applications, Methods in Enzymology, ed, C. V. Kumar, Academic Press, 2020, vol. 630, pp. 481–502, https://doi.org/ DOI:10.1016/bs.mie.2019.11.006.
- J. Jordan, C. S. Kumar and C. Theegala, Preparation and characterization of cellulase-bound magnetite nanoparticles, J. Mol. Catal. B: Enzym., 2011, 68, 139–146, DOI:10.1016/j.molcatb.2010.09.010.
- E. Ranjbakhsh, A. K. Bordbar, M. Abbasi, A. R. Khosropour and E. Shams, Enhancement of stability and catalytic activity of immobilized lipase on silica-coated modified magnetite nanoparticles, Chem. Eng. J., 2012, 179, 272–276, DOI:10.1016/j.cej.2011.10.097.
- A. Ghollobi, Z. Meshkat, K. Abnous, M. Ghayour-Mobarhan, M. Ramezani, F. H. Shandiz, K. D. Verma and M. Darroudi, Biopolymer-mediate synthesis of Fe3O4 nanoparticles and investigation of their in vitro cytotoxicity effects, J. Mol. Struct., 2017, 5, 594–599, DOI:10.1016/j.molstruc.2017.04.024.
- A. Ali, T. Shah, R. Ullah, P. Zhou, M. Guo, M. Ovais, Z. Tan and Y. Rui, Review on recent progress in magnetic nanoparticles: Synthesis, characterization, and diverse applications, Front. Chem., 2021, 9, 2296–2646, DOI:10.3389/fchem.2021.629054.
- R. Verma, S. Pathak, S. A. Kumar, S. Prawer and S. Tomljenovic-Hanic, ZnO nanomaterials: Green synthesis, toxicity evaluation and new insights in biomedical applications, J. Alloys Compd., 2021, 876, 160175, DOI:10.1016/j.jallcom.2021.160175.
- M. A. Mariño, S. Fulaz and L. Tasic, Magnetic Nanomaterials as Biocatalyst Carriers for Biomass Processing: Immobilization Strategies, Reusability, and Applications, Magnetochemistry, 2021, 7, 133, DOI:10.3390/magnetochemistry7100133.
- A. Hamid and M. N. Aftab, Cloning, purification, and characterization of recombinant thermostable β-xylanase Tnap_0700 from Thermotoga naphthophila, Appl. Biochem. Biotechnol., 2019, 189, 1274–1290, DOI:10.1007/s12010-019-03068-0.
- E. Z. Hoşgün, D. Berikten, M. Kıvanç and B. Bozan, Ethanol production from hazelnut shells through enzymatic saccharification and fermentation by low-temperature alkali pretreatment, Fuel, 2017, 196, 280–287, DOI:10.1016/j.fuel.2017.01.114.
- G. L. Miller, Use of dinitrosalicylic acid reagent for determination of reducing sugar, Anal. Chem., 1959, 31, 426–428, DOI:10.1021/ac60147a030.
- K. Endo, T. Miyasaka, S. Mochizuki, S. Aoyagi, N. Himi, H. Asahara, K. Tsujioka and K. Sakai, Development of a superoxide sensor by immobilization of superoxide dismutase, Sens. Actuators, B, 2002, 83, 30–34, DOI:10.1016/S0925-4005(01)01024-3.
- H. A. Rafiee-Pour, A. Noorbakhsh, A. Salimi and H. Ghourchian, Sensitive superoxide biosensor based on silicon carbide nanoparticles, Electroanalysis, 2010, 22, 1599–1606, DOI:10.1002/elan.200900577.
- A. Salimi, A. Noorbakhsh, H. A. Rafiee-Pour and H. Ghourchian, Direct Voltammetry of Copper, Zinc-Superoxide Dismutase Immobilized onto Electrodeposited Nickel Oxide Nanoparticles: Fabrication of Amperometric Superoxide Biosensor, Electroanalysis, 2011, 23, 683–691, DOI:10.1002/elan.201000519.
- M. Falahati, L. Ma'mani, A. A. Saboury, A. Shafiee, A. Foroumadi and A. R. Badiei, Aminopropyl-functionalized cubic Ia3d mesoporous silica nanoparticle as an efficient support for immobilization of superoxide dismutase, Biochim. Biophys. Acta, Proteins Proteomics, 2011, 1814, 1195–1202, DOI:10.1016/j.bbapap.2011.04.005.
- E. Duguet, S. Vasseur, S. Mornet and J. M. Devoisselle, Magnetic nanoparticles and their applications in medicine, Nanomedicine, 2006, 1, 157–168, DOI:10.2217/17435889.1.2.157.
- R. R. Silva, K. Y. Avelino, K. L. Ribeiro, O. L. Franco, M. D. Oliveira and C. A. Andrade, Chemical immobilization of antimicrobial peptides on biomaterial surfaces, Front. Biosci., 2016, 8, 129–142, DOI:10.2741/s453.
- R. Perfetto, S. Del Prete, D. Vullo, G. Sansone, C. M. Barone, M. Rossi, C. T. Supuran and C. Capasso, Production and covalent immobilisation of the recombinant bacterial carbonic anhydrase (SspCA) onto magnetic nanoparticles, J. Enzyme Inhib. Med. Chem., 2017, 32, 759–766, DOI:10.1080/14756366.2017.1316719.
- Z. M. Milani, R. Jalal and E. K. Goharshadi, Carbodiimide for covalent α-amylase immobilization onto magnetic nanoparticles, Int. J. Nanosci., 2017, 16, 1750015, DOI:10.1142/S0219581X17500156.
- C. Song, L. Sheng and X. Zhang, Preparation and characterization of a thermostable enzyme (Mn-SOD) immobilized on supermagnetic nanoparticles, Appl. Microbiol. Biotechnol., 2012, 96, 123–132, DOI:10.1007/s00253-011-3835-9.
- Y. Ren, J. G. Rivera, L. He, H. Kulkarni, D. K. Lee and P. B. Messersmith, Facile, high efficiency immobilization of lipase enzyme on magnetic iron oxide nanoparticles via a biomimetic coating, BMC Biotechnol., 2011, 11, 1–8, DOI:10.1186/1472-6750-11-63.
- X. Chen, B. He, M. Feng, D. Zhao and J. Sun, Immobilized laccase on magnetic nanoparticles for enhanced lignin model compounds degradation, Chin. J. Chem. Eng., 2020, 28, 2152–2159, DOI:10.1016/j.cjche.2020.02.028.
- W. Wang, N. Guo, W. Huang, Z. Zhang and X. Mao, Immobilization of chitosanases onto magnetic nanoparticles to enhance enzyme performance, Catalysts, 2018, 8, 401, DOI:10.3390/catal8090401.
- M. Abbaszadeh and P. Hejazi, Metal affinity immobilization of cellulase on Fe3O4 nanoparticles with copper as ligand for biocatalytic applications, Food Chem., 2019, 290, 47–55, DOI:10.1016/j.foodchem.2019.03.117.
- J. Alftrén and T. J. Hobley, Immobilization of cellulase mixtures on magnetic particles for hydrolysis of lignocellulose and ease of recycling, Biomass Bioenergy, 2014, 65(65), 72–78, DOI:10.1016/j.biombioe.2014.03.009.
- A. Díaz-Hernández, J. Gracida, B. E. García-Almendárez, C. Regalado, R. Núñez and A. Amaro-Reyes, Characterization of magnetic nanoparticles coated with chitosan: A potential approach for enzyme immobilization, J. Nanomater., 2018, 2018, 1–11, DOI:10.1155/2018/9468574.
- J. Sánchez-Ramírez, J. L. Martínez-Hernández, R. G. López-Campos, E. P. Segura-Ceniceros, H. Saade, R. Ramos-González, M. G. Neira-Velázquez, M. A. Medina-Morales, C. N. Aguilar and A. Ilyina, Laccase validation as pretreatment of agave waste prior to saccharification: free and immobilized in superparamagnetic nanoparticles enzyme preparations, Waste Biomass Valorization, 2018, 9, 223–234, DOI:10.1007/s12649-016-9774-z.
- C. Mateo, J. M. Palomo, G. Fernandez-Lorente, J. M. Guisan and R. Fernandez-Lafuente, Improvement of enzyme activity, stability and selectivity via immobilization techniques, Enzyme Microb. Technol., 2007, 40, 1451, DOI:10.1016/j.enzmictec.2007.01.018.
- U. V. Sojitra, S. S. Nadar and V. K. Rathod, Immobilization of pectinase onto chitosan magnetic nanoparticles by macromolecular cross-linker, Carbohydr. Polym., 2017, 157, 677–685, DOI:10.1016/j.carbpol.2016.10.018.
- R. Tarasi, M. Alipour, L. Gorgannezhad, S. Imanparast, A. Yousefi-Ahmadipour, A. Ramezani, M. R. Ganjali, A. Shafiee, M. A. Faramarzi and M. Khoobi, Laccase immobilization onto magnetic β-cyclodextrin-modified chitosan: improved enzyme stability and efficient performance for phenolic compounds elimination, Macromol. Res., 2018, 26, 755–762, DOI:10.1007/s13233-018-6095-z.
- A. R. Al-Najada, Y. Q. Almulaiky, M. Aldhahri, R. M. El-Shishtawy, S. A. Mohamed, M. Baeshen, A. F. Ammar, W. H. Abdulaal and S. A. Al-Harbi, Immobilisation of α-amylase on activated amidrazone acrylic fabric: a new approach for the enhancement of enzyme stability and reusability, Sci. Rep., 2019, 9, 1–9, DOI:10.1038/s41598-019-49206-w.
- F. A. Mostafa, A. A. El Aty, M. E. Hassan and G. E. Awad, Immobilization of xylanase on modified grafted alginate polyethyleneimine bead based on impact of sodium cation effect, Int. J. Biol. Macromol., 2019, 140, 1284–1295, DOI:10.1016/j.ijbiomac.2019.08.211.
- M. Defaei, A. Taheri-Kafrani, M. Miroliaei and P. Yaghmaei, Improvement of stability and reusability of α-amylase immobilized on naringin functionalized magnetic nanoparticles: a robust nanobiocatalyst, Int. J. Biol. Macromol., 2018, 113, 354–360, DOI:10.1016/j.ijbiomac.2018.02.147.
- K. Vasić, Ž. Knez and M. Leitgeb, Immobilization of alcohol dehydrogenase from Saccharomyces cerevisiae onto carboxymesthyl dextran-coated magnetic nanoparticles: a novel route for biocatalyst improvement via epoxy activation, Sci. Rep., 2020, 10, 1–7, DOI:10.1038/s41598-020-76463-x.
- S. S. Nadar and V. K. Rathod, A co-immobilization of pectinase and cellulase onto magnetic nanoparticles for antioxidant extraction from waste fruit peels, Biocatal. Agric. Biotechnol., 2019, 17, 470–479, DOI:10.1016/j.bcab.2018.12.015.
- M. Bilal and M. Asgher, Dye decolorization and detoxification potential of Ca-alginate beads immobilized manganese peroxidase, BMC Biotechnol., 2015, 15, 1–4, DOI:10.1186/s12896-015-0227-8.
- Q. Husain, Magnetic nanoparticles as a tool for the immobilization/stabilization of hydrolases and their applications: An overview, Biointerface. Res. Appl. Chem., 2016, 6, 1585–1605 CAS.
- F. Alnadari, Y. Xue, L. Zhou, Y. S. Hamed, M. Taha and M. F. Foda, Immobilization of β-glucosidase from Thermatoga maritima on chitin-functionalized magnetic nanoparticle via a novel thermostable chitin-binding domain, Sci. Rep., 2020, 10, 1–2, DOI:10.1038/s41598-019-57165-5.
- F. Gashtasbi, G. Ahmadian and K. A. Noghabi, New insights into the effectiveness of alpha-amylase enzyme presentation on the Bacillus subtilis spore surface by adsorption and covalent immobilization, Enzyme Microb. Technol., 2014, 64, 17–23, DOI:10.1016/j.enzmictec.2014.05.006.
- M. Akhond, K. Pashangeh, H. R. Karbalaei-Heidari and G. Absalan, Efficient immobilization of porcine pancreatic α-amylase on amino-functionalized magnetite nanoparticles: characterization and stability evaluation of the immobilized enzyme, Appl. Biochem. Biotechnol., 2016, 180, 954–968, DOI:10.1007/s12010-016-2145-1.
- A. K. El-Sayed, M. I. Abou-Dobara, A. A. El-Fallal and N. F. Omar, Heterologous expression, purification, immobilization and characterization of recombinant α-amylase AmyLa from Laceyella sp. DS3, Int. J. Biol. Macromol., 2019, 132, 1274–1281, DOI:10.1016/j.ijbiomac.2019.04.010.
- A. B. Muley, A. S. Thorat, R. S. Singhal and K. H. Babu, A tri-enzyme co-immobilized magnetic complex: Process details, kinetics, thermodynamics and applications, Int. J. Biol. Macromol., 2018, 118, 1781–1795, DOI:10.1016/j.ijbiomac.2018.07.022.
- P. Shinde, M. Musameh, Y. Gao, A. J. Robinson and I. L. Kyratzis, Immobilization and stabilization of alcohol dehydrogenase on polyvinyl alcohol fibre, Biotechnol. Rep., 2018, 19, 1–7, DOI:10.1016/j.btre.2018.e00260.
- H. Eskandarloo and A. Abbaspourrad, Production of galacto-oligosaccharides from whey permeate using β-galactosidase immobilized on functionalized glass beads, Food Chem., 2018, 251, 115–124, DOI:10.1016/j.foodchem.2018.01.068.
- K. Abdollahi, F. Yazdani and R. Panahi, Covalent immobilization of tyrosinase onto cyanuric chloride crosslinked amine-functionalized superparamagnetic nanoparticles: synthesis and characterization of the recyclable nanobiocatalyst, Int. J. Biol. Macromol., 2017, 94, 396–405, DOI:10.1016/j.ijbiomac.2016.10.058.
- Y. C. Wang, N. Zhao, J. W. Ma, J. Liu, Q. J. Yan and Z. Q. Jiang, High-level expression of a novel α-amylase from Thermomyces dupontii in Pichia pastoris and its application in maltose syrup production, Int. J. Biol. Macromol., 2019, 127, 683–692, DOI:10.1016/j.ijbiomac.2019.01.162.
- S. A. Mohamed, M. H. Al-Harbi, Y. Q. Almulaiky, I. H. Ibrahim, H. A. Salah, M. O. El-Badry, A. M. Abdel-Aty, A. S. Fahmy and R. M. El-Shishtawy, Immobilization of Trichoderma harzianum α-amylase on PPyAgNp/Fe3O4-nanocomposite: chemical and physical properties, Artif. Cells, Nanomed., Biotechnol., 2018, 46, 201–206, DOI:10.1080/21691401.2018.1453828.
- V. Mehnati-Najafabadi, A. Taheri-Kafrani, A. K. Bordbar and A. Eidi, Covalent immobilization of xylanase from Thermomyces lanuginosus on aminated superparamagnetic graphene oxide nanocomposite, J. Iran. Chem. Soc., 2019, 16, 21–31, DOI:10.1007/s13738-018-1477-x.
- A. Soozanipour, A. Taheri-Kafrani and A. L. Isfahani, Covalent attachment of xylanase on functionalized magnetic nanoparticles and determination of its activity and stability, Chem. Eng. J., 2015, 270, 235–243, DOI:10.1016/j.cej.2015.02.032.
- S. Gaikwad, A. P. Ingle, S. S. da Silva and M. Rai, Immobilized nanoparticles-mediated enzymatic hydrolysis of cellulose for clean sugar production: A novel approach, Curr. Nanosci., 2019, 15, 296–303, DOI:10.2174/1573413714666180611081759.
- B. Stuart and D. J. Ando. Modern infrared spectroscopy: analytical chemistry by open learning, Wiley, Greenwich, England, 1996 Search PubMed.
- J. Pei, Y. Huang, Y. Yang, H. Yuan, X. Liu and C. Ni, A novel layered anchoring structure immobilized cellulase via covalent binding of cellulase on MNPs anchored by LDHs, J. Inorg. Organomet. Polym. Mater., 2018, 28, 1624–1635, DOI:10.1007/s10904-018-0838-3.
- K. Khoshnevisan, A. K. Bordbar, D. Zare, D. Davoodi, M. Noruzi, M. Barkhi and M. Tabatabaei, Immobilization of cellulase enzyme on superparamagnetic nanoparticles and determination of its activity and stability, Chem. Eng. J., 2011, 171, 669–673, DOI:10.1016/j.cej.2011.04.039.
- Y. Zhou, S. Pan, X. Wei, L. Wang and Y. Liu, Immobilization of β-glucosidase onto MNPs and and evaluation of the enzymatic properties, Bioresource, 2013, 8, 2605–2619 Search PubMed . http://ncsu.edu/bioresources.
- S. Chakraborty, T. J. M. Rao and A. Goyal, Immobilization of recombinant pectate lyase from Clostridium thermocellum ATCC-27405 on magnetic nanoparticles for bioscouring of cotton fabric, Biotechnol. prog., 2016, 33, 236–244, DOI:10.1002/btpr.2379.
- L. Peng, L. Ao, W. Youmei, C. Qiuming, Y. Haizhong, L. Yuqi, P. Hao, L. Qian, W. Yanting, W. Xiaoyang, Z. Ran, T. Yuanyuan, X. Tao and P. Liangcai, Distinct Miscanthus lignocellulose improves fungus secreting cellulases and xylanases for consistently enhanced biomass saccharification of diverse bioenergy crops, Renewable Energy, 2021, 174, 799–809, DOI:10.1016/j.renene.2021.04.107.
- M. Meysam, W. Youmei, X. Chengbao, L. Peng, W. Yanting, X. Tao, T. Yuanyuan, L. Xinchun, S. Bo, Y. Xiaoe, Z. Wanbin, D. Deqiang, T. Shang-wen and P. Liangcai, Using Amaranthus green proteins as universal biosurfactant and biosorbent for effective enzymatic degradation of diverse lignocellulose residues and efficient multiple trace metals remediation of farming lands, J. Hazard. Mater., 2021, 406, 124727, DOI:10.1016/j.jhazmat.2020.124727.
Footnote |
† Both authors have equal contribution. |
|
This journal is © The Royal Society of Chemistry 2022 |