DOI:
10.1039/D1RA09043G
(Paper)
RSC Adv., 2022,
12, 1797-1806
External-stimulus-triggered conformational inversion of mechanically self-locked pseudo[1]catenane and gemini-catenanes based on A1/A2-alkyne–azide-difunctionalized pillar[5]arenes†
Received
14th December 2021
, Accepted 4th January 2022
First published on 12th January 2022
Abstract
Herein, we report a methodology for constructing mechanically self-locked molecules (MSMs) through the efficient intramolecular copper(I)-catalyzed alkyne–azide cycloaddition (CuAAC) of self-threaded A1/A2-azido-propargyl-difunctionalized pillar[5]arenes. The obtained monomeric “pseudo[1]catenane” and dimeric “gemini-catenane” were isolated and fully characterized using mass spectrometry, nuclear magnetic resonance (NMR) spectroscopy, and X-ray crystallography. Upon investigation by 1H NMR spectroscopy in chloroform, the observed motion for the threaded ring in the pseudo[1]catenane was reversibly controlled by the temperature, as demonstrated by variable-temperature 1H NMR studies. Two gemini-catenane stereoisomers were also isolated in which the two pillar[5]arene moieties threaded by two decyl chains were aligned in different topologies. Furthermore, the conformational inversion of pseudo[1]catenane and the gemini-catenanes triggered by solvents and guests was investigated and probed using 1H NMR spectroscopy, isothermal titration calorimetry, and single-crystal X-ray analysis.
Introduction
Mechanically interlocked molecules (MIMs) such as catenanes, rotaxanes, pseudo-rotaxanes, and molecular knots (knotanes) have been extensively synthesized and studied in recent decades, and they have found many applications in the design of molecular machines, chemical probes, molecular catalysts, and drug delivery systems.1–10 Macrocyclic molecules such as crown ethers, cyclodextrin, cucurbituril, and calixarene are mainly used in the construction of MIM-based pseudo-rotaxanes, rotaxanes, and catenanes.11 In addition, pillar[n]arenes have recently attracted considerable interest due to their relative ease of formation, their facile functionalization, and their exceptional ability to selectively encapsulate different kinds of guest molecules.12,13 Taking advantage of their excellent host–guest properties, the pillar[5]arene-based [2]rotaxane was first produced through end-capping by the reaction between a diamine axle and a bulky aldehyde stopper; however, the product yield was low.14 Subsequently, many examples of pillar[5]arene-based [2]rotaxanes were reported based on the improved encapsulation of the axle and an optimized capping procedure.15 In addition, pillar[5]arene-based [1]rotaxanes in which the rotor and the axle are bound covalently to form a macrocyclic compound were synthesized by capping the self-inclusion axle of the pseudo[1]rotaxane.15 Moreover, a simple [2]catenane, in which two interlocked macrocycles were present, was prepared from a guest pyridinium derivative bearing alkene groups at both ends followed by a ring-closing metathesis reaction.16
Mechanically self-locked molecules (MSMs), where one of the covalently linked macrocycles is threaded into the other macrocycle, have been found to offer fascinating molecular architectures and topological features.17 Pseudo[1]catenanes or MSMs in which the guest alkyl cyclic chain is connected to one pillar[5]arene were initially produced from difunctionalized alkyne groups at the A1/A2 positions of the macrocycle and a 1,12-diazidododecane guest via a copper(I)-catalyzed alkyne–azide 1,3-dipolar cycloaddition “click” (CuAAC) reaction.18 Another example includes the preparation of a pseudo[1]catenane by a reaction between 1,4-dicyanobutane and 1,8-diaminooctane guests (i.e., bearing different lengths of diaminoalkane chains) with difunctionalized amino or carboxy groups present at the A1/A2 positions of the pillar[5]arene, respectively.19,20 More recently, pseudo[1]catenanes based on the pillar[5]thiacrown and pillar[5]azacrown structures, wherein planar chiral inversion is triggered by a metal cation or acid and is controlled by an anion or a base, have been reported.21,22 In addition, the chirality switching of planar chiral pseudo[1]catenanes has been reported to be regulated by guest molecules.23
Since the early discovery of pillararenes, the CuAAC reaction has been employed in their functionalization to construct a wide variety of molecular architectures in high yields. Importantly, this reaction exhibits an excellent functional group tolerance, in addition to being compatible with a wide range of substrates.24–33 Thus, we report the synthesis of a pseudo[1]catenane base of self-inclusion A1/A2-asymmetric difunctionalized pillar[5]arenes. In solution, stable pseudo[1]rotaxanes that are formed from the self-inclusion of the straight-chain bromodecyl group on the pillar[5]arene rim, enable the construction of pseudo[1]catenane and gemini-catenane structures via the conversion of bromine to an azide functional group followed by intramolecular CuAAC reaction. The role of external stimuli (i.e., a solvent or guest) in governing the conformational changes from self-included to dethreaded structures via host–guest interactions, which can consequently trigger conformational inversion, are probed by nuclear magnetic resonance (NMR) spectroscopy, isothermal titration calorimetry (ITC), and X-ray single-crystal diffraction. To the best our knowledge, this is the first report of intramolecular self-locked pseudo[1]catenane and 1
:
1 gemini-catenane structures based on A1/A2-asymmetric difunctionalized pillar[5]arenes and their conformational inversion.
Results and discussion
Synthesis
Many pseudo[1]rotaxane based pillar[n]arenes have been reported in recent years due to their ability for self-inclusion when a long chain substituent is present on the macrocyclic rim. Recently, we reported the synthesis of a series of A1/A2-asymmetric-defunctionalized pillar[5]arenes and their concentration-dependent supramolecular self-assembly behaviors.34 Based on such systems, precursor compound 1-(10-bromodecyloxy)-4-propargyloxybenzene was synthesized via a two-step reaction starting from hydroquinone. An A1/A2-asymmetric difunctionalized pillar[5]arene bearing propargyl and bromo functional groups (Pillar-1) was then synthesized by the condensation of an asymmetric hydroquinone derivative and 1,4-dimethoxybenzene in a 1
:
4 ratio in the presence of paraformaldehyde and BF3·OEt2, as shown in Scheme 1. The azide derivative, Pillar-2, was obtained following the reaction of bromo-derivative Pillar-1 with sodium azide (NaN3) in DMF at room temperature (Scheme 1).
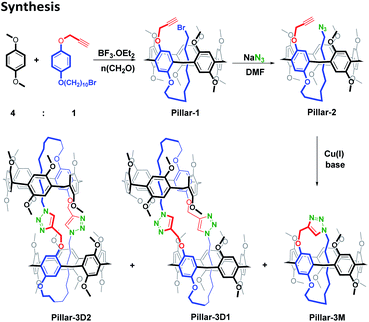 |
| Scheme 1 Synthesis of a self-locked pseudo[1]catenane (Pillar-3M) and a gemini-catenane (Pillar-3D1-2) via the copper(I)-catalyzed alkyne–azide 1,3-dipolar cycloaddition (CuAAC) reaction. | |
The 1H NMR spectrum of Pillar-1 (Fig. S6†) shows a high upfield shift for the methylene proton resonances of the 10-bromodecyloxy arm, thereby indicating the successful formation of the pseudo[1]rotaxane. Moreover, the self-inclusion of the long alkyl chain inside the pillar[5]arene cavity was not affected when the 1H NMR spectrum of Pillar-1 was recorded at a higher temperature of 313 K (Fig. S6†). To investigate whether the inclusion behavior of the 10-bromodecyloxy chain in the pillar[5]arene cavity involved the self-inclusion of a double-threaded dimer (i.e., an interpenetration structure) or an n-mer, 1H NMR spectroscopy was carried out at a range of Pillar-1 concentrations (1–64 mM) in chloroform-d at 298 K (Fig. S7†). The insignificant changes observed in the chemical shift data clearly demonstrate that the behavior of Pillar-1 is concentration independent, suggesting that Pillar-1 exists predominately in its single aggregate form (i.e., the pseudo[1]rotaxane). Further evidence was obtained from two-dimensional diffusion-ordered 1H NMR (DOSY) experiments. More specifically, the DOSY spectra of Pillar-1 at concentrations of 2 and 50 mM revealed a single set of signals, confirming the presence of a single aggregate form in solution. In the case where multiple aggregate sizes exist, a change in the diffusion coefficient (D) would be expected in the DOSY spectrum because larger aggregates possess larger hydrodynamic radii (R), and R is inversely proportional to D according to the Strokes–Einstein equation [D = kBT/(6πηR)], where T denotes the temperature, kB is the Boltzmann constant, and η is the dynamic viscosity of the solvent. As the concentration of Pillar-1 was increased from 2 to 50 mM, the weight-averaged diffusion coefficient (D) decreased from 6.72 × 10−10 to 5.62 × 10−10 m2 s−1, which was insufficient to suggest variation in the average aggregate dimensions at increasing concentrations (D2/D50 = 1.19). Similarly, the azide derivative Pillar-2 showed an upfield shift for the protons of the long alkyl chain, thereby further indicating that self-inclusion of the alkyl chain took place inside the pillar[5]arene cavity.
Subsequently, azide derivative Pillar-2 containing the long alkyl chain was subjected to the CuAAC reaction at room temperature as outlined in Scheme 1. Thin-layer chromatography confirmed the consumption of the starting material, and three distinct spots were separated by column chromatography using a dichloromethane/ethyl acetate mixture for elution (80
:
20, v/v). The first spot gave a high-resolution mass spectrometry (HRMS) signal at m/z 942.4905 [M + H]+, which is similar to the mass of Pillar-2 (i.e., m/z = 941.4823 [M]+). However, the 1H NMR spectra of Pillar-2 and the unknown compound corresponding to the first spot differed significantly (Fig. S9†) as did their melting points, i.e., 135–136 °C for Pillar-2, and 209–210 °C for the unknown. It should be noted here that the latter melting point corresponds to that of Pillar-3M. Thus, the notable upfield chemical shift observed for the methylene protons of the alkyl chain along with higher splitting pattern that reflects their magnetically nonequivalent environments indicate the formation of a threaded self-locked pseudo[1]catenane, Pillar-3M, and precludes the formation of an endo-spirocyclic-like structure (Fig. 1a). Formation of the aromatic triazole moiety in this structure was confirmed by the downfield shift of the methine H12 proton from 2.16 to 5.65 ppm, in addition to the newly formed peaks observed at 124.5 and 140.6 ppm in the 13C NMR spectrum of Pillar-3M (Fig. S35†).
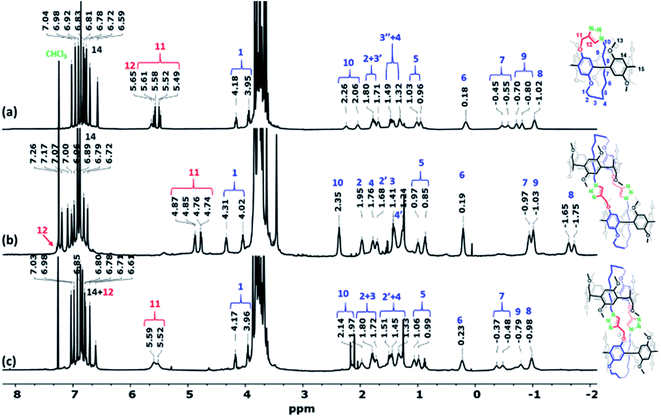 |
| Fig. 1 1H NMR spectra (600 MHz, CDCl3, 298 K) of (a) pseudo[1]catenane Pillar-3M, and gemini-catenanes (b) Pillar-3D1, and (c) Pillar-3D2. | |
HRMS analysis of the isolated second (Pillar-3D1, vide infra) and third (Pillar-3D2, vide infra) spots showed similar m/z values of 1883.9242 [M + H]+ and 1883.9063 [M + H]+, respectively, which represent double the mass of Pillar-3M. It was therefore suggested that these dimeric products are either nonthreaded 1
:
1 dimer or self-locked gemini-catenanes.19 The presence of a partially reacted dimer was ruled out since the 1H NMR data indicated the absence of a resonance corresponding to a propargyl group. Moreover, the unusual chemical upfield shift of the decamethylene protons excluded the presence of a nonthreaded dimer (Fig. 1). Close inspection of the 1H NMR spectra shown in Fig. 1 indicates significant differences in the chemical shifts for both the aliphatic and aromatic regions of the spectrum that was assumed to correlate to Pillar-3D1 (Fig. 1b) when compared to the spectrum of Pillar-3M (Fig. 1a), whereas the spec-trum that was considered to correlate to Pillar-3D2 (Fig. 1c) generally exhibited similar resonances to the 1H NMR spectrum of Pillar-3M. For example, the methylene H11 protons of Pillar-3D1 split into two sets of signals with noticeably higher upfield shifts (i.e., 4.74 and 4.85 ppm) compared to the same methylene signal in the Pillar-3M pseudo[1]catenane (5.49 and 5.58 ppm) and in the gemini-catenane-type structure Pillar-3D2 (5.52 and 5.59 ppm). Similarly, H8 split into two sets of signals with higher upfield shifts of −1.75 and −1.65 ppm, whereas the H8 protons of Pillar-3M and Pillar-3D2 appeared as single signals at lower upfield shifts of −1.02 and −1.03 ppm, respectively. These significant Fig. 2 Different views of the X-ray single-crystal structure of the Pillar-3D1 gemini-catenane, in (a) CDCl3 and (b) CD2Cl2. Red, grey/green/light blue, and purple represent oxygen, carbon, and nitrogen, respectively. Hydrogen atoms are omitted for clarity.
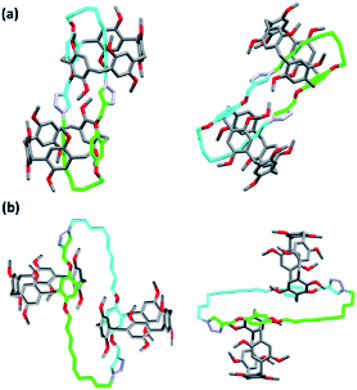 |
| Fig. 2 Different views of the X-ray single-crystal structure of the Pillar-3D1 gemini-catenane, in (a) CDCl3 and (b) CD2Cl2. Red, grey/green/light blue, and purple represent oxygen, carbon, and nitrogen, respectively. Hydrogen atoms are omitted for clarity. | |
Differences in the chemical shifts observed for Pillar-3D1 suggests that the two pillar[5]arene rings are aligned in opposite directions (i.e., C2-symmetric, erythro), whereas Pillar-3D2 presents as the S2-symmetric isomer (achiral, ‘thero’). All our attempts to separate the stereoisomers of Pillar-3M and Pillar-3D1 using chiral HPLC were unsuccessful at this point. The opposite alignment of the pillararene rings in Pillar-3D1 was con-firmed by single-crystal X-ray diffraction measurements following the growth of a suitable crystal from CHCl3 by the slow evaporation method (Fig. 2a). Unfortunately, all attempts to grow suitable crystals of Pillar-3M and Pillar-3D2 were unsuccessful. The melting points of the compounds isolated from these two spots were also dif-ferent, i.e., 252–253 °C (second spot, Pillar-3D1) and 233–234 °C (third spot, Pillar-3D2).
The unsymmetrical nature of the threaded cyclic system in Pillar-3M enables the molecular motions to be monitored by simple 1H NMR spectroscopy. Interestingly, when the 1H NMR spectrum was recorded at 298 K in chloroform-d immediately after the preparation of Pillar-3M, the H12 methine hydrogen atom of the triazole moiety appeared at 6.25 ppm, whereas the H9 and H10 methylene protons of the octyl chain appeared as two sets of signals at −0.64 and −0.52 ppm and at 2.65 and 3.05 ppm, respectively (Fig. 3a). After allowing the NMR solution to stand for 24 h, the H9, H10, and H12 protons moved upfield, whereas the remainder of the aliphatic methylene protons shifted downfield (Fig. 3b). In addition, the H11 methylene protons shifted slightly downfield due to the effect of the deshielding region of the pillararene system. A similar movement of the proton signals was observed after 48 h (Fig. 3c), while beyond 48 h, the system was in equilibrium and no further shifts were detected. These results indicate that the triazole moiety moves inside the pillar[5]arene cavity and that the alkyl methylene chain moves out of the cavity due to aromatic donor–acceptor interactions between the triazole moiety and the aromatic groups in the pillararene ring. Upon drying and redissolving the sample in chloroform-d, the protons signals reset back to their original positions (Fig. 3a).
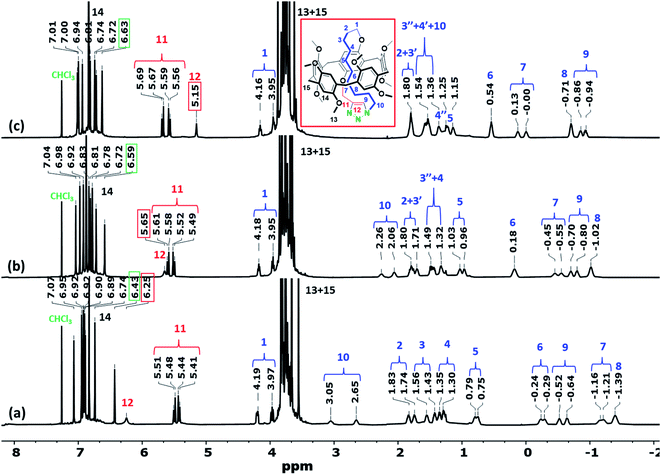 |
| Fig. 3 1H NMR spectra (600 MHz, CDCl3, 298 K) of Pillar-3M (a) immediately after preparation, (b) after 24 h, and (c) after 48 h. | |
The observed molecular motion of the threaded cyclic ring in CDCl3 was further investigated by variable-temperature (VT) 1H NMR experiments (Fig. 4). Most notably, the chemical shift of the H12 methine proton of the triazole ring shifted gradually upfield from 6.49 to 5.15 ppm when the temperature was increased from −40 to 50 °C. In contrast, a downfield shift was observed for the H8 methylene protons, i.e., from −1.40 to −0.63 ppm. These results indicate that increasing the temperature led to movement of the triazole fragment inside the pillar[5]arene cavity, while at lower temperatures, the triazole ring was able to move outside of the pillararene cavity. The observed molecular motion of the threaded chain was therefore confirmed to be a reversible process induced by changes in temperature. No temperature-induced conformer inversion was observed during the VT-NMR study.
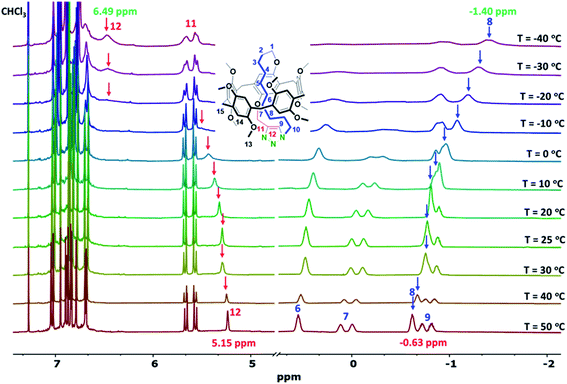 |
| Fig. 4 Variable-temperature 1H NMR spectra (600 MHz, CDCl3) of the Pillar-3M pseudo[1]catenane. | |
Conformational inversion studies. The chirality switching of chiral-planar pseudo[1]catenanes triggered by solvents,18 metal cations,21 acids,22 and guest molecules23 has been well documented in the literature. Such inversion occurs when one of the incorporated macrocycles in the bicyclic structure is dethreaded by aromatic ring tumbling. Thus, we screened a range of solvents (i.e., DMSO-d6, pyridine-d5, THF-d10, DMF-d7, and CD2Cl2) for their ability to trigger the inversion of the Pillar-3M pseudo[1]catenane. The 1H NMR spectra recorded in DMSO-d6, pyridine-d5, and THF-d10 showed no significant changes in chemical shifts for the proton signals of Pillar-3M, indicating that dethreading did not take place (Fig. S10†). In contrast, the proton signals of the decylene chain coalesced and shifted downfield when the 1H NMR spectra were recorded in DMF-d7 and CD2Cl2 (Fig. S11†). For comparison, the stacked 1H NMR spectra of Pillar-3M in CDCl3 and CD2Cl2 at 298 K are shown in Fig. S12.† In the case of the CDCl3 solvent system, the location of the methylene proton signals of the decylene chain in the far upfield region indicates that Pillar-3M adopted a self-included conformation (pseudo[1]catenane) as a result of the shielding effect of the pillar[5]arene cavity (Fig. S12a†). In contrast, the 1H NMR spectrum of Pillar-3M in CD2Cl2 revealed the presence of a dethreaded conformer due to peak coalescence and the downfield chemical shift of the decylene chain proton signals (Fig. S12b†). On the other hand, solvent trigger conformational inversion of gemini-catenanes from self-included “in” to the de-threading “out” conformers involve simultaneous tumbling of the functionalized aromatic units. Stacked 1H NMR spectra for gemini-catenane, Pillar-3D1 in CDCl3 and CD2Cl2 are shown in Fig. S13.†Similarly, the threaded conformer Pillar-3D1 in CDCl3, show high upfield chemical shift for the methylene proton signals for the decylene chain caused by the shielding effect of pillar[5]arene cavity (Fig. S13a†), while in CD2Cl2, the decylene chain lactated outside the macrocyclic cavity as indicated by the downfield chemical shift and the coalesce of the methylene proton signals (Fig. S13b†). 1H NMR recorder in CD2Cl2 for Pillar-3D2 shows similar behavior to Pillar-3D1 (Fig. S20†). The conformational assignment of the de-threaded conformer of Pillar-3D1 was confirmed by single-crystal X-ray diffraction measurement when suitable crystal for analysis obtained from CH2Cl2/DMF solvent mixture (Fig. 2b).
Based on previous literature, it was considered that in CD2Cl2, the observed conformational change from self-included to dethreaded conformers was driven by host–guest complexation between the pillar[5]arene cavity and the CD2Cl2 solvent.23 To further investigate the influence of host–guest complexation on the conformational change, adiponitrile was selected as a guest due to its ability to form highly stable 1
:
1 host–guest complexes with pillar[5]arenes, and because of its effectiveness in the conformational inversion of pseudo[1]catenane-based pillar[5]arenes.19,23 Thus, Fig. 5 shows the 1H NMR spectra recorded for the complexation of Pillar-3M with different concentrations of the adiponitrile guest G in CDCl3. Upon the addition of 1 molar equivalent of G to Pillar-3M, significant downfield shifts were observed for the methylene proton signals of the decylene chain, and the methylene proton signals of G appeared as highly shielded signals (Fig. 5c) as a result of de-threading of the alkyl chain and guest encapsulation inside the pillar[5]arene cavity. At a lower guest concentration, signals corresponding to both the self-included and dethreaded conformers were observed in the 1H NMR spectrum (Fig. 5b).
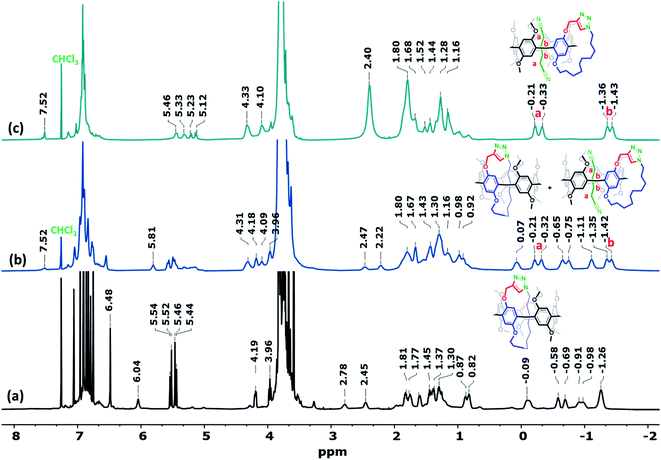 |
| Fig. 5 1H NMR spectra (600 MHz, CDCl3, 298 K) of (a) the Pillar-3M pseudo[1]catenane at a concentration of 25 mM, and (b) and (c) Pillar-3M (25 mM) in the presence of the adiponitrile guest G at concentrations of (b) 7.5 mM and (c) 25 mM. | |
The conformational inversion of gemini-catenanes Pillar-3D1 and Pillar-3D2 promoted by the adiponitrile guest was then studied by 1H NMR spectroscopy. More specifically, inspection of 1H NMR spectra recorded in CDCl3 upon the incremental addition of adiponitrile revealed that the inclusion of a single guest molecule in one pillar[5]arene cavity was sufficient to tumble the functionalized aromatic units of the gemini-catenane and trigger chiral inversion. These results are supported by the crystal structure of Pillar-3D1 obtained after crystallization from CH2Cl2/DMF, which shows that only one pillar[5]arene cavity was occupied by a DMF molecule (Fig. S5†). The 1H NMR spectra of Pillar-3D1 obtained at different concentrations of adiponitrile are shown in Fig. 6. At a guest concentration of less than one equivalent, proton resonances corresponding to the self-included and dethreaded Pillar-3D1 conformers were observed in the 1H NMR spectrum, as can be seen from the highly shielded signals of the host and guest components. Upon increasing the host-to-guest ratio to 1
:
1, no signals corresponding to the threaded dimer were observed, and the addition of two equivalents of adiponitrile consolidated the conformational change to the dethreaded conformer.
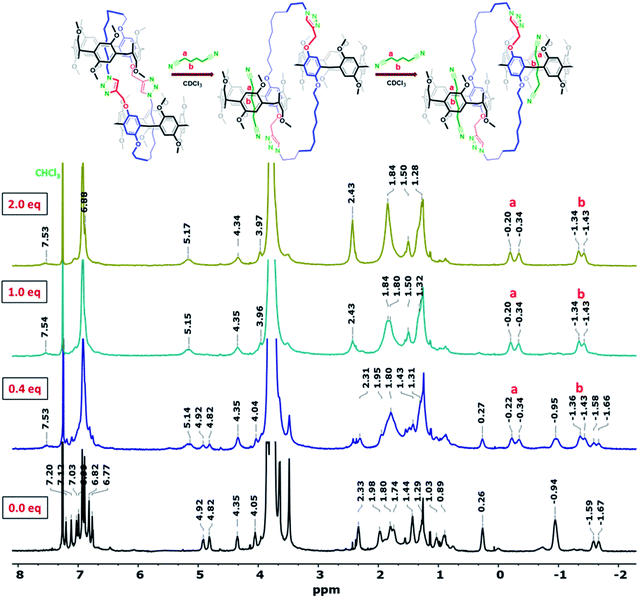 |
| Fig. 6 1H NMR spectra (600 MHz, CDCl3, 298 K) of the Pillar-3D1 gemini-catenane (10 mM) in the presence of different concentrations of the adiponitrile guest G. | |
The isothermal titration calorimetry (ITC) technique has been successfully employed to study various reversible noncovalent supramolecular interactions in solution.34–36 Quantitative information concerning the binding affinity and the thermodynamic parameters of the conformational change from the self-included to dethreaded conformers of Pillar-3M (10 mM) triggered by the adiponitrile guest were therefore obtained using ITC measurements. The experimentally-obtained binding molar ratio “n” of 0.91 is close to unity, which indicates a 1
:
1 host-to-guest complexation ratio, wherein the association constant, K11, was determined to be 4.93 ± 0.24 × 103 M−1. The negative enthalpy change (ΔH° = −11.86 kJ mol−1) and positive entropy change (TΔS° = 5.26 kJ mol−1) for this process indicate that the guest-promoted conformational inversion of Pillar-3M was driven by both enthalpically and entropically favorable changes, and thus, it is a spontaneous process (i.e., ΔG° = −17.12 kJ mol−1). A similar trend was observed for the Pillar-3D1 and Pillar-3D2 gemini-catenanes, with an increased contribution of favorable entropy changes being determined (Table 1). In both cases, the experimental binding molar ratio “n” was close to 2, which indicates a 1
:
2 host-to-guest complexation ratio with association constants, K12, of 1.52 ± 0.08 ×103 (K11 = 1.43 ± 0.11 ×104) and 1.44 ± 0.14 × 103 (K11 = 1.26 ± 0.09 ×104) being determined for Pillar-3D1 and Pillar-3D2, respectively. For comparison, an analogous ITC host–guest complexation experiment was carried out using the permethylated DMP5 pillar[5]arene macrocycle at 10 mM in the presence of adiponitrile as the guest. The calculated thermodynamic parameters show that the molecular binding behavior is driven by van der Waals interactions, with a major contribution also being present from a particularly favorable enthalpy change (ΔH° = −23.23 kJ mol−1; TΔS° = 0.25 kJ mol−1). The measured association constant for the complexation of DMP5 with adiponitrile (K11 = 1.30 ± 0.07 × 104 M−1) was found to be approximately one order of magnitude higher than Pillar-3M due to the more readily accessible macrocyclic cavity that can easily accommodate the guest molecule.
Table 1 ITC thermodynamic parameters associated with the adiponitrile guest-triggered conformational inversion of the pseudo[1]catenane and gemini-catenanes based the pillar[5]arene structurea
Host |
ΔH° (kJ mol−1) |
TΔS° (kJ mol−1) |
Ka (M−1) |
Binding molar ratio (n) |
All titrations were carried out in chloroform at 25 °C with a fixed host concentration of 10 mM and varying guest concentrations. Association constant K12. |
DMP5 |
−23.23 |
0.25 |
1.30 ± 0.07 × 104 |
0.85 |
Pillar-3M |
−11.86 |
5.26 |
4.93 ± 0.24 × 103 |
0.91 |
Pillar-3D1 |
−10.67 |
6.90 |
1.52 ± 0.08 × 103b |
1.94 |
Pillar-3D2 |
−9.55 |
8.48 |
1.44 ± 0.14 × 103b |
2.13 |
Experimental
Materials and methods
Nuclear magnetic resonance (NMR) spectroscopy was carried out on a Bruker Avance II 600 MHz spectrometer (Bruker, Germany). Electron impact ionization (EI) mass spectrometry was performed using a Thermo Scientific DFS High-Resolution GC/MS mass spectrometer (Thermo Scientific, USA). Electro-spray ionization in high-resolution mode was carried out using a Waters Xevo G2-S Qtof LC MS/MS mass spectrometer (Waters, USA). Single-crystal data analysis was carried out using a Bruker X8 Prospector diffractometer (Bruker, Germany). Data were collected at −123 °C (Oxford Cryosystems, UK). Flash column chromatography was performed using silica gel (Silica gel 60, 40–60 mesh ASTM, EMD Millipore, Merck KGaA, Germany). The DMF, acetonitrile, and dichloroethane solvents were distilled prior to use. All other reagents and solvents were of reagent grade purity and were used without further purification. Permethylated-pillar[5]arene (DMP5) was synthesized according to the literature.37
Preparation of single crystals for X-ray diffraction
Suitable single crystals of the synthesized inclusion complex Pillar-3D1 were grown by the slow solvent evaporation method from chloroform and a dichloromethane/DMF mixture. The data were collected on a Bruker X8 prospector diffractometer (Bruker, Germany) using Cu-Kα radiation at −123 °C. The reflection frames were then integrated with the Bruker SAINT Software package using a narrow-frame algorithm. Finally, the structure was solved using the Bruker SHELXTL Software Package and refined using SHELXL-2017/1. All non-hydrogen atoms were refined anisotropically and hydrogen atoms were refined using the riding model. CCDC 2049467 and 2081939.†
ITC measurements
All ITC studies were carried out on an Affinity ITC system (TA Instruments, USA). The data were analyzed using NanoAnalyze, Version 3.10.0. For the ITC host–guest complexation experiments, the DMP5, Pillar-3M, Pillar-3D1, and Pillar-3D2 hosts were dissolved in CHCl3 (300 μL, 10 mM) and added to the reaction cell with CHCl3 in the reference cell. A 100 mM adiponitrile solution was taken up into a syringe, and the titration was performed over 40 injections with a volume of 2 μL per injection and a time interval of 240 s between injections.
4-Propargyloxyphenol. Hydroquinone (6.60 g, 60 mmol) was dissolved in DMF (30 mL) at room temperature, and to this solution, potassium carbonate (12.42 g, 90 mmol) was added. This solution was stirred for 30 min and then stirred further at 80 °C overnight after the addition of propargyl bromide (4.64 mL, 60 mmol). Thereafter, the solvent was removed by evaporation under reduced pressure, and the crude product was extracted with dichloromethane. The desired compound was then purified by column chromatography using a dichloromethane/hexane mixture (75
:
25, v/v) and was obtained as a white solid following solvent evaporation (5.8 g, 65%). 1H NMR (600 MHz, CDCl3) δ: 2.52 (t, J = 2.4 and 2.4 Hz, 1H), 4.65 (d, J = 2.4 Hz, 2H), 6.79 (m, 2H), 6.89 (m, 2H). 13C NMR (150 MHz, CDCl3) δ: 56.9, 75.6, 79.1, 116.3, 116.6. HRMS: (m/z): calcd for [M]+ 1480519 (for C9H8O2); found 148.0518.
1-(10-Bromodecyloxy)-4-propargylbenzene. 4-Propargyloxyphenol (2.20 g, 15 mmol) was dissolved in dry DMF (20 mL) at room temperature and potassium carbonate (2.80 g, 20 mmol) was added. The resulting solution was stirred for 30 min and then stirred further at 80 °C overnight after adding 1,10-dibromodecane (4.5 mL, 20 mmol). Then, the reaction mixture was poured into ice-cold water (250 mL) and the precipitate was filtered and washed with water. The desired compound was further purified by column chromatography using a dichloromethane/hexane mixture (60
:
40, v/v) and collected as a white solid after solvent evaporation (2.9 g, 53%). 1H NMR (600 MHz, CDCl3) δ: 1.37 (m, 8H), 1.47 (m, 4H), 1.77 (m, 2H), 1.86 (m, 2H), 2.52 (t, J = 2.4 and 2.4 Hz, 1H), 3.42 (t, J = 7.2 and 6.6 Hz, 2H), 3.92 (t, J = 6.6 and 6.6 Hz, 2H), 4.65 (d, J = 2.4 Hz, 2H), 6.85 (m, 2H), 6.93 (m, 2H). 13C NMR (150 MHz, CDCl3) δ: 26.2, 28.4, 29.0, 29.6, 29.7, 33.0, 34.3, 56.9, 68.8, 75.5, 79.2, 115.5.116.3. HRMS: (m/z): calcd for [M]+ 366.1189 (for C19H27O2Br); found 366.1189.
Pillar-1. Paraformaldehyde (0.93 g, 30 mmol) was added to a solution of 1,4-dimethoxybenzene (1.10 g, 8.0 mmol) and 1-(10-bromodecyloxy)-4-propargylbenzene (0.734 g, 2 mmol) in dichloroethane (30 mL) under a nitrogen atmosphere. Boron trifluoride diethyl etherate (BF3·OEt2) (1.25 mL, 10 mmol) was then added to the solution and the mixture was stirred at 0 °C for 1 h. Thereafter, MeOH (200 mL) was poured into the reaction mixture and the solution was concentrated and dissolved in CH2Cl2 (100 mL). The solution was then washed with aqueous NaHCO3 (2 × 50 mL) and H2O (50 mL). Finally, the organic layer was dried over anhydrous Na2SO4, concentrated under vacuum, and subjected to silica gel chromatography (65
:
35 v/v, CH2Cl2/hexane) to give Pillar-1 as a white solid (705 mg, 36%). Mp 161–162 °C. 1H NMR (600 MHz, CDCl3) δ: −0.77 (s, 2H), −0.53 (s, 2H), −0.07 (s, 2H), 0.71 (s, 4H), 1.14 (m, 2H), 1.36 (m, 2H), 1.56 (m, 2H), 1.90 (m, 2H), 2.40 (s, 1H), 3.77 (m, 9H), 3.82 (m, 25H), 3.96 (t, J = 6.6 and 6.0 Hz, 2H), 4.64 (m, 2H), 6.86 (m, 4H), 6.93 (s, 1H), 6.95 (m, 2H), 6.96 (s, 1H), 6.98 (m, 2H). 13C NMR (150 MHz, CDCl3) δ: 26.0, 27.9, 28.1, 29.2, 29.3, 29.3, 29.3, 29.8, 29.9, 31.6, 33.8, 55.5, 55.5, 55.5, 55.6, 55.7, 55.9, 56.2, 68.3, 74.7, 79.7, 113.3, 113.4, 113.5, 113.9, 114.3, 114.8, 128.0, 128.1, 128.2, 128.3, 128.3, 128.5, 128.6, 128.7, 148.8, 150.3, 150.4, 150.5, 150.6, 150.7. HRMS: (m/z): calcd for [M]+: 978.3912 (for C56H67O10Br1); found 978.3910.
Pillar-2. Pillar-1 (200 mg, 0.20 mmol) and sodium azide (200 mg, 3 mmol) were dissolved in dry DMF (10 mL) and stirred at room temperature for 12 h. After this time, the reaction mixture was poured into ice-cold water (50 mL) and the precipitate was filtered and washed with water (2 × 10 mL). The desired compound was then recrystallized from dichloromethane/methanol as a white solid (174 mg, 90%). Mp 135–136 °C. 1H NMR (600 MHz, CDCl3) δ: −0.15 (s, 2H), 0.05 (s, 2H), 0.22 (s, 4H), 0.72 (m, 2H), 1.04 (m, 2H), 1.23 (m, 2H), 1.43 (m, 2H), 1.75 (m, 2H), 2.07 (m, 3H), 3.64 (m, 22H), 3.69 (m, 12H), 3.84 (t, J = 6.6 and 6.0 Hz, 2H), 4.42 (d, J = 2.4 Hz, 2H), 6.70 (s, 1H), 6.73 (m, 3H), 6.79 (m, 4H). 13C NMR (150 MHz, CDCl3) δ: 26.1, 26.1, 27.9, 28.5, 29.1, 29.4, 29.5, 29.6, 29.6, 29.7, 29.8, 30.2, 51.3, 55.6, 55.7, 55.7, 55.7, 55.8, 55.9, 56.3, 68.5, 74.8, 79.4, 113.7, 113.8, 113.8, 113.9, 114.1, 115.0, 115.1, 128.0, 128.3, 128.3, 128.4, 128.4, 128.4, 128.5, 128.6, 128.7, 128.9, 148.9, 150.6, 150.6, 150.7, 150.7, 150.7, 150.8, 150.9. HRMS: (m/z): calcd for [M]+: 941.4821 (for C56H67O10N3); found 941.4823.Copper(I)-catalyzed alkyne–azide 1,3-dipolar cycloaddition reaction of 1-(10-bromodecyloxy)-4-propargylpillar[5]arene Pillar-3M and Pillar-3D(1–2).14,25 Pillar-2 (100 mg, 0.1 mmol) was dissolved in dry acetone (30 mL) and degassed for 5 min. Thereafter, a degassed solution of tetrakis(acetonitrile)copper(I) hexafluorophosphate, Cu(CH3CN)4PF6 (18.4 mg, 0.048 mmol) and tris[(1-benzyl-1H-1,2,3-triazol-4-yl)methyl]amine (TBTA, 26.4 mg, 0.048 mmol) in acetonitrile (1.5 mL) was added. The mixture was then stirred at room temperature under a nitrogen atmosphere for 24 h and concentrated under vacuum. The desired products were subsequently separated from the reaction mixture by silica gel column chromatography using a dichloromethane/ethyl acetate mixture (80
:
20, v/v).
Pillar-3M. White solid. Yield (42 mg, 42%). Mp: 209–210 °C. 1H NMR (600 MHz, CDCl3) δ: −1.39 (s, 2H), −1.20 (m, 2H), −0.65 (s, 1H), −0.52 (s, 1H), −0.27 (m, 2H), 0.77 (m, 2H), 1.32 (m, 1H), 1.39 (m, 1H), 1.45 (m, 1H), 1.59 (m, 1H), 1.73 (m, 1H), 1.84 (m, 1H), 2.52 (s, 1H), 2.65 (s, 1H), 3.80 (m, 34H), 3.97 (m, 1H), 4.19 (m, 1H), 5.51 (m, 2H), 6.25 (s, 1H), 6.48 (s, 1H), 6.77 (s, 1H), 6.82 (s, 1H), 6.85 (s, 1H), 6.89 (s, 1H), 6.93 (s, 3H), 6.97 (s, 1H), 7.08 (s, 1H). 13C NMR (150 MHz, CDCl3) δ: 22.5, 25.1, 26.1, 26.2, 26.5, 26.7, 27.0, 27.0, 28.0, 28.7, 28.8, 29.5, 30.1, 49.8, 55.3, 55.4, 55.7, 56.0, 56.3, 56.7, 57.0, 57.1, 60.3, 67.0, 113.4, 113.5, 113.6, 113.8, 114.1, 114.2, 114.4, 114.6, 115.5, 117.0, 124.6, 127.9, 128.1, 128.1, 128.4, 128.4, 128.5, 129.1, 129.4, 129.4, 129.5, 140.7, 146.0, 150.5, 150.6, 150.6, 150.7, 150.7, 150.9, 151.0, 151.1. HRMS: (m/z): calcd for [M + H]+: 942.4905 (for C56H68O10N3); found 942.4905.
Pillar-3D1. White solid. Yield (15 mg, 15%). Mp: 252–253 °C. 1H NMR (600 MHz, CDCl3) δ: −1.70 (d, 2H), −0.10 (m, 4H), 0.19 (s, 2H), 0.91 (m, 2H), 1.24 (m, 1H), 1.41 (m, 2H), 1.68 (m, 1H), 1.76 (m, 1H), 1.95 (m, 1H), 2.35 (m, 2H), 3.50 (s, 4H), 3.77 (m, 30H), 4.02 (m, 1H), 4.31 (s, 1H), 4.75 (m, 1H), 4.86 (m, 1H), 6.78 (s, 1H), 6.83 (s, 2H), 6.91 (m, 4H), 7.01 (m, 1H), 7.05 (s, 1H), 7.13 (s, 1H), 7.21 (s, 1H). 13C NMR (150 MHz, CDCl3) δ: 23.0, 25.2, 26.0, 26.3, 26.6, 27.4, 27.7, 29.0, 29.1, 29.4, 29.4, 29.9, 30.1, 49.5, 55.2, 55.6, 56.0, 56.0, 56.2, 57.2, 63.1, 67.3, 112.8, 113.2, 113.3, 114.0, 114.7, 114.9, 116.2, 116.5, 128.2, 128.3, 128.4, 128.5, 128.6, 128.8, 129.0, 129.1, 129.6, 149.6, 150.1, 150.2, 150.4, 150.6, 150.8, 151.0, 151.1. HRMS: (m/z): calcd for [M + H+]: 1883.9731 (for C112H135O20N6); found 1883.9242.
Pillar-3D2. White solid. Yield (12 mg, 12%). Mp: 233–234 °C. 1H NMR (600 MHz, CDCl3) δ: −0.98 (s, 2H), −0.79 (m, 2H), −0.48 (s, 1H), −0.37 (s, 1H), 0.23 (m, 2H), 0.99 (s, 1H), 1.06 (s, 1H), 1.33 (m, 1H), 1.48 (m, 2H), 1.76 (m, 2H), 1.80 (m, 1H), 1.97 (m, 1H), 2.14 (m, 1H), 3.77 (m, 34H), 3.96 (m, 1H), 4.17 (m, 1H), 5.55 (m, 2H), 6.67 (s, 1H), 6.72 (s, 1H), 6.78 (s, 2H), 6.80 (m, 2H), 6.85 (m, 3H), 6.98 (s, 1H), 7.03 (s, 1H). 13C NMR (150 MHz, CDCl3) δ: 22.5, 25.0, 26.2, 26.5, 26.9, 27.1, 28.1, 28.6, 28.8, 29.3, 29.5, 29.9, 30.2, 55.4, 55.5, 55.8, 56.0, 57.0, 67.0, 113.4, 113.5, 113.7, 113.8, 114.2, 114.5, 114.7, 115.5, 117.0, 127.8, 128.1, 128.2, 128.4, 128.5, 128.6, 129.2, 129.3, 129.5, 129.6, 146.0, 150.5, 150.5, 150.6, 150.7, 150.7, 150.9, 151.0, 151.0, 151.1. HRMS: (m/z): calcd for [M + H]+: 1883.9731 (for C112H135O20N6); found 1883.9063.
Conclusions
A1/A2-difunctionalized pillar[5]arenes bearing 10-bromodecyloxy and propargyl substituents were synthesized by the co-cyclization of their corresponding hydroquinone derivatives. The 10-bromodecyloxy substituent in the pillar[5]arene rim (Pillar-1) undergoes self-inclusion, as evidenced by the unusual high upfield shift of the proton signals during nuclear magnetic resonance (NMR) spectroscopy. 1H NMR concentration experiments and diffusion-ordered spectroscopy measurements demonstrated that the inclusion behavior of the long chain 10-bromodecyloxy substituent was concentration-independent and resulted in the formation of a relatively stable pseudo[1]rotaxane. In addition, the pseudo[1]catenane and 1
:
1 gemini-catenanes were successfully synthesized by an intramolecular copper(I)-catalyzed alkyne–azide 1,3-dipolar cycloaddition reaction following conversion to the A1/A2-difunctional azido-propargyl pseudo[1]rotaxane derivative (Pillar-2). In a chloroform solution, the molecular motion of the threaded ring in the unsymmetrical pseudo[1]catenane was induced by changes in the temperature, as demonstrated by variable-temperature 1H NMR experiments. Furthermore, the conformational inversion triggered by solvents and a guest molecule was demonstrated by NMR, ITC, and X-ray analyses. The obtained data provide a molecular-level picture of the conformational inversion of the pseudo[1]catenane and gemini-catenanes triggered by an external solvent or guest stimulus. The calculated thermodynamic parameters obtained from the ITC measurements show that the conformational changes of the pseudo[1]catenane and the gemini-catenanes, i.e., from threaded to dethreaded conformers, are driven by favorable enthalpy and entropy changes. Further studies into chirality switching are currently underway in our laboratories, and the results will be presented in due course.
Conflicts of interest
There are no conflicts to declare.
Acknowledgements
The support received from the Kuwait University, made available through research Grant no. SC08/19, and the facilities of the RSPU (grant no. GS 01/03 and GS 03/08) are gratefully acknowledged. The authors also thank Fatemeh H. Alipour for carrying out the melting point analyses.
Notes and references
- J. F. Stoddart, Mechanically Interlocked Molecules (MIMs) – Molecular Shuttles, Switches, and Machines, Angew. Chem., Int. Ed., 2017, 56, 11094–11125 CrossRef CAS PubMed.
- Y. Liu, Y. Chen and H.-Y. Zhang, Handbook of Macrocyclic Supramolecular Assembly, Springer, Singapore, 2019, pp. 1–25, DOI: DOI:10.1007/978-981-13-1744-6_5-1.
- C. Cheng and J. F. Stoddart, Wholly Synthetic Molecular Machines, ChemPhysChem, 2016, 17, 1780–1793 CrossRef CAS PubMed.
- A. C. Fahrenbach, C. J. Bruns, D. Cao and J. F. Stoddart, Ground-State Thermodynamics of Bistable Redox-Active Donor–Acceptor Mechanically Interlocked Molecules, Acc. Chem. Res., 2012, 45(9), 1581–1592 CrossRef CAS PubMed.
- J. M. Abendroth, O. S. Bushuyev, P. S. Weiss and C. J. Barrett, Controlling Motion at the Nanoscale: Rise of the Molecular Machines, ACS Nano, 2015, 9(8), 7746–7768 CrossRef CAS PubMed.
- G. Yu, B. C. Yung, Z. Zhou, Z. Mao and X. Chen, Artificial Molecular Machines in Nanotheranostics, ACS Nano, 2018, 12(1), 7–12 CrossRef CAS PubMed.
- L. Fang, S. Basu, C.-H. Sue, A. C. Fahrenbach and J. F. Stoddart, Syntheses and Dynamics of Donor-Acceptor [2]Catenanes in Water, J. Am. Chem. Soc., 2011, 133, 396–399 CrossRef CAS PubMed.
- A. Martinez-Cuezva, A. Saura-Sanmartin, M. Alajarin and J. Berna, Mechanically Interlocked Catalysts for Asymmetric Synthesis, ACS Catal., 2020, 10(14), 7719–7733 CrossRef CAS.
- A. W. Heard and S. M. Goldup, Simplicity in the Design, Operation, and Applications of Mechanically Interlocked Molecular Machines, ACS Cent. Sci., 2020, 6(2), 117–128 CrossRef CAS PubMed.
- H. Y. Au-Yeung, C.-C. Yee, A. W. H. Ng and K. Hu, Strategies to Assemble Catenanes with Multiple Interlocked Macrocycles, Inorg. Chem., 2018, 57(7), 3475–3485 CrossRef CAS PubMed.
- G. Li, L. Wang, L. Wu, Z. Guo, J. Zhao, Y. Liu, R. Bai and X. Yan, Woven Polymer Networks via the Topological Transformation of a [2]Catenane, J. Am. Chem. Soc., 2020, 142(33), 14343–14349 CrossRef CAS PubMed.
- M. Xue, Y. Yang, X. Chi, X. Yan and F. Huang, Development of Pseudorotaxanes and Rotaxanes: From Synthesis to Stimuli-Responsive Motions to Applications, Chem. Rev., 2015, 115(15), 7398–7501 CrossRef CAS PubMed.
- F. Guo, Y. Sun, B. Xi and G. Diao, Recent Progress in the Research on the Host-Guest Chemistry of Pillar[n]arenes, Supramol. Chem., 2018, 30, 81–92 CrossRef CAS.
- T. Ogoshi, T. Yamagishi and Y. Nakamoto, Pillar-Shaped Macrocyclic Hosts Pillar[n]arenes: New Key Players for Supramolecular Chemistry, Chem. Rev., 2016, 116, 7937–8002 CrossRef CAS PubMed.
- T. Ogoshi, D. Yamafuji, T. Aoki and T. A. Yamagishi, Thermally Responsive Shuttling Behavior of a Pillar[6]arene-Based [2]Rotaxane, Chem. Commun., 2012, 48, 6842–6844 RSC.
- K. Yang, S. Chao, F. Zhang, Y. Pei and Z. Pei, Recent Advances in the Development of Rotaxanes and Pseudorotaxanes Based on Pillar[n]arenes: From Construction to Application, Chem. Commun., 2019, 55, 13198–13210 RSC.
- K. Kitajima, T. Ogoshi and T. Yamagishi, Diastereoselective Synthesis of a [2]Catenane from a Pillar[5]arene and a Pyridinium Derivative, Chem. Commun., 2014, 50, 2925–2927 RSC.
- T. J. Hubin and D. H. Busch, Template Routes to Interlocked Molecular Structures and Orderly Molecular Entanglements, Coord. Chem. Rev., 2000, 200, 5–52 CrossRef.
- T. Ogoshi, T. Akutsu, D. Yamafuji, T. Aoki and T. Yamagishi, Solvent- and Achiral-Guest-Triggered Chiral Inversion in a Planar, Angew. Chem., Int. Ed., 2013, 52, 8111–8115 CrossRef CAS PubMed.
- S.-H. Li, H.-Y. Zhang, X. Xu and Y. Liu, Mechanically Self-Locked Chiral Gemini-Catenanes, Nat. Commun., 2015, 6, 7590–7596 CrossRef PubMed.
- M. Cheng, Q. Wanga, Y. Cao, Y. Pan, Z. Yang, J. Jiang and L. Wanga, Two Pillar[5]arene-Based Mechanically Self-Locked Molecules (MSMs): Planar Chirality in Crystals and Conformer Inversion in Solutions, Tetrahedron Lett., 2016, 57, 4133–4137 CrossRef CAS.
- E. Lee, H. Ju, I.-H. Park, J. H. Jung, M. Ikeda, S. Kuwahara, Y. Habata and S. Lee, Pseudo[1]catenane-Type Pillar[5]thiacrown Whose Planar Chiral Inversion Is Triggered by Metal Cation and Controlled by Anion, J. Am. Chem. Soc., 2018, 140(30), 9669–9677 CrossRef CAS PubMed.
- H. Liang, B. Hua, F. Xu, L.-S. Gan, L. Shao and F. Huang, Acid/Base-Tunable Unimolecular Chirality Switching of a Pillar[5]azacrown Pseudo[1]catenane, J. Am. Chem. Soc., 2020, 142(46), 19772–19778 CrossRef CAS PubMed.
- Y.-F. Yang, W.-B. Hu, L. Shi, S.-G. Li, X.-L. Zhao, Y. A. Liu, J.-S. Li, B. Jiang and W. Ke, Guest-Regulated Chirality Switching of Planar Chiral Pseudo[1]catenanes, Org. Biomol. Chem., 2018, 16, 2028–2032 RSC.
- H. Guo, J. Ye, Z. Zhang, Y. Wang, X. Yuan, C. Ou, Y. Ding, C. Yan, J. Wang and Y. Yao, Pillar[5]arene-Based [2]Rotaxane: Synthesis, Characterization, and Application in a Coupling Reaction, Inorg. Chem., 2020, 59(17), 11915–11919 CrossRef CAS PubMed.
- N. L. Strutt, H. Zhang, M. A. Giesener, J. Leia and J. F. J. Stoddart, A Self-Complexing and Self-Assembling Pillar[5]arene, Chem. Commun., 2012, 48, 1647–1649 RSC.
- G. Yu, Y. Ma, C. Han, Y. Yong, G. Tang, Z. Mao, C. Gao and F. Huang, A Sugar-Functionalized Amphiphilic Pillar[5]arene: Synthesis, Self-Assembly in Water, and Application in Bacterial Cell Agglutination, J. Am. Chem. Soc., 2013, 135, 10310–10313 CrossRef CAS PubMed.
- J. Bi, X. Zeng, D. Tian and H. Li, Temperature-Responsive Switch Constructed from an Anthracene-Functionalized Pillar[5]arene-Based Host–Guest System, Org. Lett., 2016, 18(5), 1092–1095 CrossRef CAS PubMed.
- G. Yu, Z. Zhang, J. He, Z. Abliz and F. Huang, Cavity-Extended Pillar[5]arenes: Syntheses and Host–Guest Complexation with Paraquat and Bispyridinium Derivatives, Eur. J. Org. Chem., 2012, 30, 5902–5907 CrossRef.
- H. Deng, X. Shu, X. Hua, J. Li, X. Jia and C. Li, Synthesis of a Fully Functionalized Pillar[5]arene by ‘Click Chemistry’ and Its Effective Binding toward Neutral Alkanediamines, Tetrahedron Lett., 2012, 53, 4609–4612 CrossRef CAS.
- M. Cheng, Q. Wanga, Y. Cao, Y. Pan, Z. Yang, J. Jiang and L. Wanga, Two Pillar[5]arene-Based Mechanically Self-Locked Molecules (MSMs): Planar Chirality in Crystals and Conformer Inversion in Solutions, Tetrahedron Lett., 2016, 57, 4133–4137 CrossRef CAS.
- M. Wanga, X. Dua, H. Tiana, Q. Jiaa, R. Denga, Y. Cuia, C. Wangb and K. Meguellati, Design and Synthesis of Self-Included Pillar[5]arene-Based Bis-[1]rotaxanes, Chin. Chem. Lett., 2019, 30, 345–348 CrossRef.
- T. Kakuta, T. Yamagishia and T. Ogoshi, Supramolecular Chemistry of Pillar[n]arenes Functionalized by a Copper(I)-Catalyzed. Alkyne–Azide Cycloaddition “Click” Reaction, Chem. Commun., 2017, 53, 5250 RSC.
- T. Ogoshi, R. Shiga, M. Hashizume and T. Yamagishi, “Clickable” Pillar[5]arenes, Chem. Commun., 2011, 47, 6927–6929 RSC.
- T. F. Al-Azemi and M. Vinodh, Pillar[5]arene-Based Self-Assembled Linear Supramolecular Polymer Driven by Guest Halogen–Halogen Interactions in Solid and Solution States, Polym. Chem., 2020, 11, 3305–3312 RSC.
- T. F. Al-Azemi and M. Vinodh, Pillar[5]arene-Based Self-Assembled Linear Supramolecular Polymer Driven by Guest Halogen–Halogen Interactions in Solid and Solution States, Polym. Chem., 2020, 11, 3305–3312 RSC.
- T. Ogoshi, T. Aoki, K. Kitajima, S. Fujinami, T. A. Yamagishi and Y. Nakamoto, Facile, Rapid, and High-Yield Synthesis of Pillar[5]arene from Commercially Available Reagents and Its X-ray Crystal Structure, J. Org. Chem., 2011, 76, 328–331 CrossRef CAS PubMed.
Footnote |
† Electronic supplementary information (ESI) available. CCDC 2049467 and 2081939. For ESI and crystallographic data in CIF or other electronic format see DOI: 10.1039/d1ra09043g |
|
This journal is © The Royal Society of Chemistry 2022 |