DOI:
10.1039/D1RA07657D
(Review Article)
RSC Adv., 2022,
12, 7689-7711
Tannic acid: a crosslinker leading to versatile functional polymeric networks: a review
Received
16th October 2021
, Accepted 22nd February 2022
First published on 10th March 2022
Abstract
With the thriving of mussel-inspired polyphenol chemistry as well as the demand for low-cost analogues to polydopamine in adhesive design, tannic acid has gradually become a research focus because of its wide availability, health benefits and special chemical properties. As a natural building block, tannic acid could be used as a crosslinker either supramolecularly or chemically, ensuring versatile functional polymeric networks for various applications. Up to now, a systematic summary on tannic-acid-based networks has still been waiting for an update and outlook. In this review, the common features of tannic acid are summarized in detail, followed by the introduction of covalent and non-covalent crosslinking methods leading to various tannic-acid-based materials. Moreover, recent progress in the application of tannic acid composites is also summarized, including bone regeneration, skin adhesives, wound dressings, drug loading and photothermal conversion. Above all, we also provide further prospects concerning tannic-acid-crosslinked materials.
1. Introduction
Natural-resource-rich building blocks that can polymerize into functional composites have long been a source of scientific inspiration.1,2 When it comes to complex superstructures, obtained from the assembly of simple naturally derived building blocks, they have gained widespread interest for engineering materials with improved and synergistic properties.3,4 With the aim of meeting complex biological requirements, the charm of natural materials lies in the exploitation of organic and inorganic building blocks to prepare hybrid materials with synergistic properties.5 Plant polyphenols, classified as a family of naturally derived compounds, contain a high concentration of dihydroxyphenyl (catechol) or trihydroxyphenyl (pyrogallol) moieties that link to versatile biological functions such as corrosion resistance, coloration, mechanical reinforcement, and prevention of radiation damage.6,7 A person who has a high dietary intake of polyphenols generally has a lower incidence of tumor diseases, osteoporosis, and diabetes as well as neurodegenerative and cardiovascular diseases.8 The rising interest in polyphenols over the past few decades is attributable to their broad spectrum of molecular tectonics and chemical properties.9
Tannic acid (TA) is a natural polyphenol, which exists in a wide variety of plants as a colourless to pale yellow solid with an astringent taste (Fig. 1). TA could be exacted from natural sources such as the bark of oak, chestnut, hemlock, and mangrove and the leaves of certain sumacs and plant galls.10 With multiple ways of obtaining it, TA is the most abundant natural compound after cellulose, hemicellulose and lignin, serving as a crucial commercial raw material and has traditionally been utilized as a tanning agent in leather, coating, adhesive, surgery, pharmaceutical and food industrial applications.11 Since tannic acid contains a complex compound of polyphenols, which contain numerous catechol and pyrogallol aromatic ring structures, a series of unique chemical properties can be listed. (i) The water solubility of TA: TA has a solubility of 300 g L−1 in water, which is suitable for chemical modification in a green way. Moreover, the TA aqueous solution is stable in air and can be stored in the lab for more than 30 days.12,13 (ii) The biocompatibility of TA: TA is a natural polyphenol that is cheap in price, environmentally harmless, widely available, and generally biocompatible.14 Furthermore, TA is hydrolysable as well as biodegradable under certain hydrolytic conditions, in which the breakage of the ester linkage between two pyrogallol groups plays a significant role.15 (iii) Universal adhesiveness of TA: the various intermolecular reactions between TA and various nucleophiles (amide bonds, thiols and amines) on a protein-rich tissue surface endow a TA-derived material with superior skin affinity and underwater adhesion capacity.16 (iv) The metal–chelating properties of TA: TA can participate in biological processes through significant functional metal chelation, which emerged as a facile method for constructing new frameworks and sensors.17,18 Moreover, with a bunch of o-dihydroxy and trihydroxy aromatic rings, this naturally extracted compound can reduce noble metal ions (e.g., AuIII and AgI) to corresponding metallic nanoparticles (NPs).19 At this point, TA plays a role as a large molecular stabilizer to provide stabilization to the NPs formed from the chelated metal species because of the steric hindrance provided by aromatic rings, and the electron donation/acceptance interactions among adjacent phenolic groups.20 (v) Supermolecular conjugation between TA and polymers: researchers have proved that TA has the ability to interact with biomacromolecules such as chitosan, collagen, gelatin, silk fiber, and albumin via supramolecular interactions.21 For instance, TA has high hemostatic efficiency since it could conjugate with various proteins in blood, leading to instant coagulation.22 Characteristically, abundant hydrophobic phenyl groups enable TA to adsorb firmly to a graphene nanosheet via π–π stacking, endowing graphene with water dispersion stability since a large number of hydrophilic phenolic hydroxyl groups decorate the graphene surface.23 The benzene rings of TA can also trigger π–π conjugation with the benzene ring of dye molecules.24 (vi) Clinical uses of TA: TA is also recognized as an anti-bacterial, anti-oxidant, anti-viral and anti-inflammation agent,25 and has been applied as a precise therapy for burn wounds and other therapeutic applications.26,27 (vii) UV-adsorption property of TA: As a widely distributed polyphenol in plant cells and tissues, TA possess broad absorption across UV regions, which is promising in skin protection.28
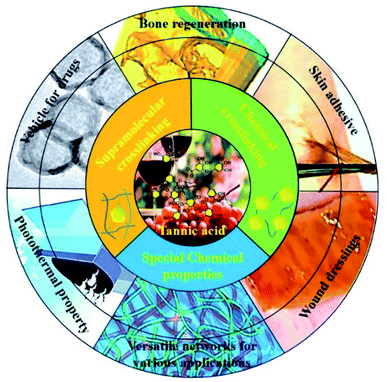 |
| Fig. 1 Versatile networks derived from tannic acid and their wide scope of applications. | |
Because of its specific polyhydric phenol structure, TA was found to be able to function as a crosslinker either physically or chemically, resulting in the emergence of versatile polymeric networks. When it comes to physical or supramolecular crosslinking, TA is able to complex or crosslink macromolecules at multiple binding sites through multiple interactions, including hydrogen bonding, ionic bonding and π–π/cation–π interactions.29 Three illustrative examples on these interactions in daily life are given here: (1) researchers found that TA present in red wine or tea accounts for the deposition of a coating on the inner surface of the containers in which those liquids are stored.30 In daily life, pyrogallol-rich liquids such as red wine, red tea, and coffee were also capable of successfully coating TA on flexible surfaces via intermolecular hydrogen bonds.31 (2) With reference to textile dyeing and coloration processes, TA can induce mordant combinations on a textile surface via the formation of a metal–phenolic network, manifesting that TA has desirable metal ion chelating/reducing capability.32 (3) Specifically, TA-based decoration of graphene or nanocarbon tubes has been proved to be a cost-effective and environmentally-friendly strategy leading to hybrid functional composites.33 Not confined to physical crosslinking to construct supramolecular materials, TA can also react with other functional molecules to form a covalent polymeric network via chemical crosslinking. Therefore, versatile functional polymers can be derived from TA-based polyphenol chemistry, which are promising in fields such as adhesive hydrogels, drug loading nano-carriers, thermosets, tissue engineering and so on (Table 1).
Table 1 Versatile routes leading to a TA-based polymeric network, through either physical crosslinking or chemical crosslinking
Entries for physical crosslinking |
Reagent or substrate |
TA based network |
1 |
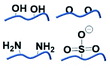 |
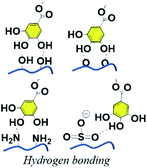 |
2 |
Mn+ |
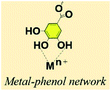 |
3 |
 |
 |
Entries for chemical crosslinking |
Reagent or substrate |
TA based network |
1 |
 |
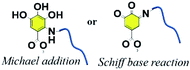 |
2 |
 |
 |
3 |
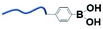 |
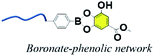 |
4 |
 |
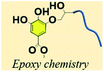 |
5 |
 |
 |
6 |
 |
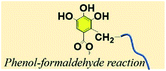 |
7 |
 |
 |
8 |
 |
 |
9 |
 |
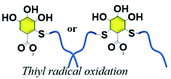 |
2. Physical/supramolecular crosslinking
Programmed crosslinking of functional molecules into 3D networks in non-covalent ways is a general route to obtain supramolecular materials, requiring the involvement of polymers or monomers to provide functional groups allowing the formation of dynamic supramolecular interactions through physical crosslinking. Nonetheless, some desirable functional groups in general do not exist in most water-soluble polymers.34 A tedious post-functionalization method through covalent bonding of the useful moieties onto polymer chains is normally used to fulfill the requirement of physical crosslinking. Therefore, researchers turned to discovering natural building blocks, leading to a bunch of supramolecular materials with advantages such as facile preparation, robustness and low cost.35
2.1. Hydrogen bonding
H-bonding is one of the most fundamental interactions facilitating the adhesion ability of polyphenol towards multiple surfaces. A large number of phenyl groups existing in TA enable it to couple to a vast majority of molecules containing a carboxyl group, hydroxyl group, amino group, ether linkage, fluorine atom, N-containing heterocyclic residues, sulfonate or phosphate through hydrogen bonding.36 As TA derivatives bind to a given surface through hydrogen bonding, bidentate or tridentate hydrogen bonds could be formed owing to the catechol or pyrogallol groups. Such interactions are stronger than single-ligand hydrogen bonding, which means that a TA crosslinked material could be used as an underwater adhesive to adapt to an ever-changing operational environment.37 Natural polymers, such as collagen, elastin, gelatin, and chitosan, or synthetic polymers such as poly(vinyl alcohol) (PVA), poly(ethylene glycol) (PEG), poly(vinylpyrrolidone) (PVP) and so on, could be crosslinked by TA through hydrogen bonding for biomedical applications. One of the advantages of such combinations is that strong interactions between TA and polymers could resist enzymatic degradation caused by collagenase or hyaluronidase.38 It should be noted that supramolecular assemblies of TA–polymers via hydrogen bonding are highly pH-dependent, which further influences the mechanical properties and stability of the resulting networks. Under acidic conditions, the catechol and pyrogallol groups of TA are mainly protonated, becoming desirable hydrogen donors readily interacting with hydrogen-accepting building blocks. Under neutral conditions, the catechol and pyrogallol groups of TA prefer to be ionized, causing the dissociation of H-bonds between hydrogen-accepting building blocks and TA. A further increase in pH value gives rise to an unstable TA–polymer complex since the polyphenol groups are oxidized to the quinone form under ambient conditions.39
As to a TA–PVA complex, a partially crosslinked network could be obtained at pH values ranging from 3 to 4, which could form a semitranslucent hydrogel after a few freeze–thaw cycles. The resulting TA–PVA hydrogel presented better elasticity and a more yellow color accompanied by an increase in the TA mass ratio during hydrogel preparation, as can be seen in the work provided by Hong.40 A fully crosslinked TA–PVA network could be obtained when the pH value was further decreased to 2, which was unstable in water and precipitated as a bulk plastic-like dry polymeric material, as evidenced by Niu et al. (Fig. 2).41 Owing to the high density of hydrogen bonds, this polymer composite had a toughness of 354 MJ m−3 along with a tensile strength of ca. 104.2 MPa and a Young's modulus of ca. 3.53 GPa. The intramolecular interaction between TA and PEG has also been involved to improve the mechanical properties of polymeric composites. Recently, Lee et al. successfully fabricated hyaluronic acid (HA)–TA hydrogels, in which TA was incorporated into the hydrogel network through hydrogen bonding with ether groups of polyethylene glycol diglycidyl ether (PEGDE).42 They also proved that the TA-based hydrogel network could fulfill demands over a prolonged clinical period as well as physiological functions with antioxidant, antibacterial and anti-inflammatory properties. Moreover, as reported in the work of Du et al., a hybrid hydrogel containing PEG, quaternized chitosan (QCS) and TA (PEGDA/QCS/TA) was developed based on mussel-inspired chemistry.43 The incorporation of TA via multiple hydrogen bonding with PEG enabled properties such as anti-swelling, high mechanical strength, antimicrobial and excellent adhesive properties to versatile surfaces.
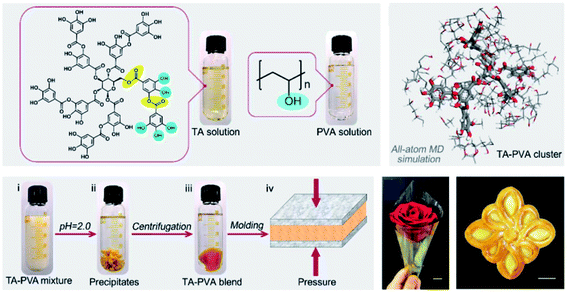 |
| Fig. 2 Schematic illustration of the preparation process of TA–PVA composites. Reproduced from ref. 41 with permission from the American Chemical Society, copyright 2020. | |
2.2. π–π stacking
A combination between graphene and TA was desirable for numerous researchers in fields such as dye adsorption, material reinforcement and three-dimensional (3D) graphene macrostructures.44,45 Over the past few years, graphene has gained substantial attention from researchers owing to its high tensile strength, huge specific area, distinguished electrical conductivity and gas tightness.46,47 Nonetheless, graphene nanosheets generally tend to aggregate and restack with each other because of strong π–π stacking, hydrophobic adsorption and van der Waals forces, leading to hindrance of the large accessible interfaces of graphene sheets.48 Pointing to this issue, Liu and Wang et al. reported that natural phenolic acids such as TA could promote the self-assembly of graphene nanosheets into 3D structures.49,50 In these cases, TA not only adsorbed firmly onto the graphene surface via π–π stacking, endowing graphene with water dispersion stability, but also reduced graphene oxide (GO) into reduced graphene oxide (RGO) for enhanced chemical stability.
Moreover, catechol and pyrogallol moieties of TA have features such as tissue adhesion, ready chemical reactivity and metal ion reduction, making TA a good interface stabilizer for polymer/graphene hybrid materials.51 For instance, Liu et al. developed a new chitin-based composite hydrogel reinforced by TA-modified reduced GO (TRGO) prepared via a facile freezing–thawing approach (Fig. 3). This TRGO reinforced hydrogel exhibited excellent adsorption capacity towards Congo red (CR).44 Zhao et al. synthesized a TA-modified graphene/SiO2 hybrid filler, which was proved to be a facile route leading to high-performance rubber nanocomposites.52 TA–GO composites can also incorporate noble metal nanoparticles due to the reducing property of polyphenols, thus providing TA–GO-based material with catalytic or antibacterial performance. In the work provided by Luo et al., a rapid, low-cost, and environmentally-friendly method was developed to obtain gold nanoparticle (Au NP) modified graphene hydrogel by taking advantage of TA-based reducibility.53 This Au NP modified graphene hydrogel (Au@TA-GH) manifested greatly advanced catalytic activities over unsupported and other polymer-supported Au NPs in the process of methylene blue (MB) reduction. Similarly, Wang et al. presented an ultralight 3D graphene hybrid aerogel containing TA, GO and modified polyaspartamide [PolyAspAm(EDA/EA)].45 The corresponding hydrogel is considered to be formed via various interactions such as hydrogen bonding, covalent bonding, and weak π–π interactions. These 3D polymer–graphene composite materials containing TA-mediated noble metal nanoparticles are promising for use as new interfacially active materials for a wide range of long-term catalytic applications.
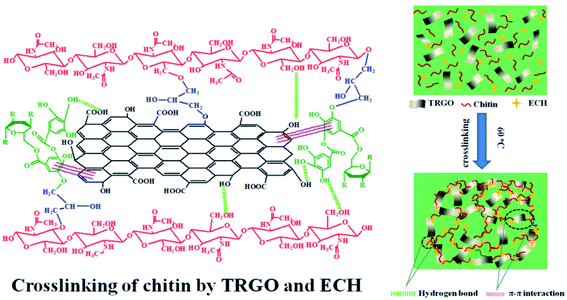 |
| Fig. 3 Preparation and the crosslinked network of chitin–TRGO composite hydrogels. Reproduced from ref. 44 with permission from Springer, copyright 2020. | |
Apart from TA-functionalized graphene, non-covalently functionalized carbon nanotubes (CNTs) via π–π interactions can also be obtained using TA as the stabilizer, which enhances the water dispersion property of CNTs in the polymeric network. He et al. developed a conductive hydrogel by incorporating TA-coated CNTs into a polyvinyl alcohol (PVA) hydrogel matrix, in which water and glycerol were used as a dispersion medium (Fig. 4).54 The resulting TA–CNT–glycerol–PVA (TCGP) hydrogel exhibited excellent freezing-resistance properties (−30 °C), long-term moisture retention performance (10 days), and outstanding strain or pressure sensitivity. Additionally, the TCGP hydrogel could be used not only as a wearable skin sensor for the tracking of multiple human motions, but also as a probe for detecting human electrophysiological information even in a harsh environment. In the work provided by Tan et al., a photoactive TA (pTA) was first synthesized via the ring-opening reaction between TA and glycidyl methacrylate (GMA), and then CNTs were coated with pTA via π–π interactions to obtain UV-curable carbon nanotubes (pTA/MWCNTs).55 Applied as a filler, this material was effective for the reinforcement of acrylated epoxidized soybean oil (AESO).
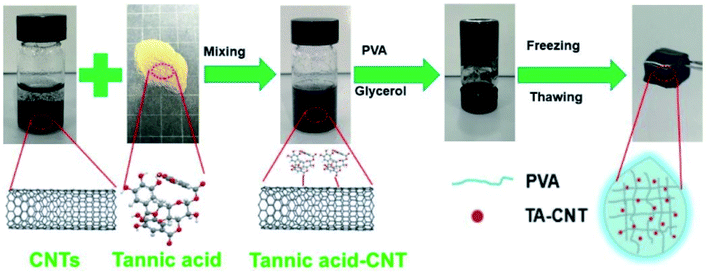 |
| Fig. 4 The preparation of TA–CNT–glycerol–PVA hydrogel for moist-electric generation. Reproduced from ref. 54 with permission from the Royal Society of Chemistry, copyright 2020. | |
2.3. Metal–phenol network (MPN)
TA-based metal–organic networks have aroused widespread attention because of their diverse properties, including (i) pH and temperature responsiveness derived from the supramolecular nature of dynamic coordination bonds, (ii) the combined biomedical properties of both metals and polyphenols, (iii) controlled crosslinking density achieved by variation of the metal/polyphenol mass ratio.56 These supramolecular materials have great potential in drug vehicles,57 optical materials,58 chemical sensors,59 gas adsorption,60 power storage,61 and catalysts.62 Specifically, TA and FeIII were usually chosen as the organic ligand and the inorganic crosslinker, respectively. Caruso et al. proposed that the MPN network formed between FeIII and TA is pH-dependent. At pH 2.0–3.0, most of the pyrogallol moieties were protonated, which gave rise to rapid destabilization of coordination bonds and disassembly of the network. The stability of the TA–FeIII complex increased at pH > 7 since TA could chelate with each FeIII ion to form a stable octahedral complex.63,64 Additionally, other metals, including Al, V, Cr, Mn, Co, Ni, Cu, Zn, Zr, Rh, were coordinated with TA to form functional MPN networks on particulate substrates. For instance, the RhIII–TA complex possessed a catalytic function for the hydrogenation of quinolone.65 The MnII–TA complex exhibited the highest relaxivity r2, which is of the order of 60 s−1 mm−1 and generally sufficient for in vivo magnetic resonance imaging.66
Straightforward crosslinking of a TA–polymer complex into bulk material is still a challenge since the strong tendency for supramolecular interactions often leads to partial coacervation rather than a homogeneous bulk material.67 Balanced control over the hydrogen bonding or ionic interactions between polymers and TA molecules should be considered, which can be solved by adding metal ions to TA–polymer composites. Based on the above concept, Fan et al. functionalized polymers such as polyvinylpyrrolidone (PVP), poly(ethylene glycol) (PEG), poly(sodium 4-styrenesulfonate) (PSS), and poly(dimethyldiallylammonium chloride) (PDDA) with catechol/pyrogallol groups by TA and FeIII sequentially (Fig. 5).68 The special performance of such hydrogels includes pH-stimulated assembly, rapid self-healing, and radical scavenging abilities. Since TA can affect the biological availability or activity of metal ions through chelation, the pH-sensitive property of MPN can also be utilized to construct smart biomaterials. Ninan et al. integrated the advantageous properties of TA, ZnCl2 and carboxylated agarose to fabricate a series of pH-sensitive hydrogels for wound healing (Fig. 6).69 At acidic pH, the polyphenol groups of TA were highly protonated and relatively few phenolate binding sites were allowed to complex with zinc ions. As the pH rose, deprotonation of TA occurred, triggering the formation of an MPN network. The hydrogels could release TA in a pH-dependent manner, which showed great potential as wound dressing. Li et al. also used FeIII to stabilize the interaction between TA and polyaniline, thus forming a stable hydrogel for a carbon aerogel precursor.70 Li et al. synthesized a new dual-network self-healing hydrogel, in which AlIII was employed to stabilize the TA–poly(acrylic acid)–chitosan complex.71
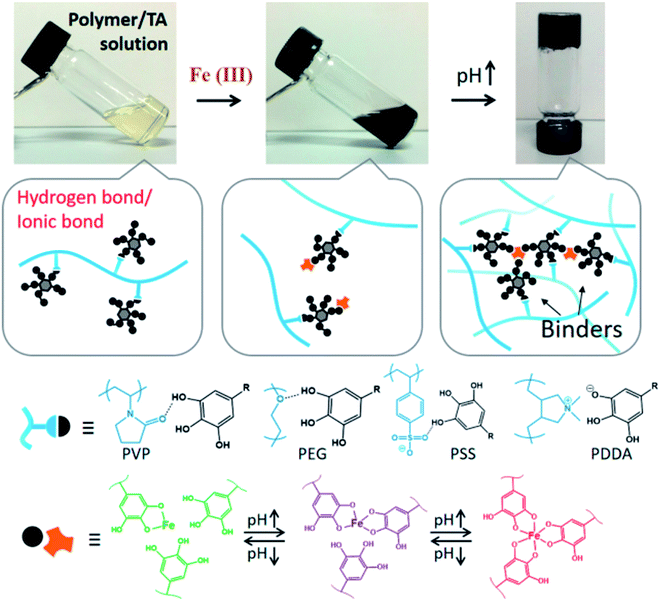 |
| Fig. 5 Formation mechanism of TA–polymer hydrogels based on polymer/TA/FeIII. Reproduced from ref. 68 with permission from the American Chemical Society, copyright 2017. | |
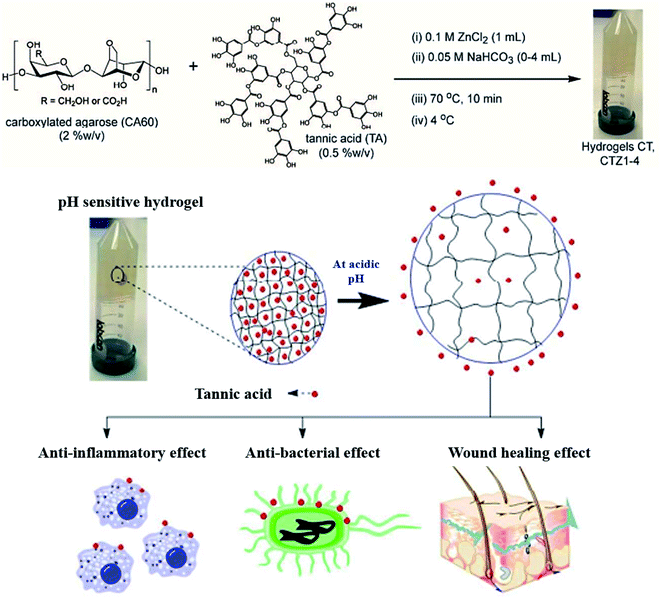 |
| Fig. 6 Anti-microbial and anti-inflammatory pH-sensitive TA-based composite hydrogels for wound healing. Reproduced from ref. 69 with permission from the American Chemical Society, copyright 2016. | |
3. Chemical crosslinking
The increasing interest in phenolic molecules is ascribed to their broad scope of chemical properties and multivalent reactive sites.6,7,72 In reference to the adhesive property of mussels, polydopamine (PDA) has been applied in surface modification to tailor nanoparticles, membranes or hydrogels at multiple scales because of their excellent adhesion ability, mild modification conditions without pollution, and versatile secondary reaction types.17,73 TA, with 2–12 gallic units per molecule, also has similar characteristics of chemical reactivity to PDA. Numerous chemical crosslinking methods including phenol–amine Michael/Schiff base reaction, phenol–thiol Michael addition, phenol–boronic acid reaction, phenol–epoxy ring-opening reaction, phenol–alcohol Mitsunobu reaction, phenol–aldehyde reaction, phenolic acylation and radical oxidation have been utilized by various researchers for extensive uses.
3.1. Phenol–amine Michael and Schiff base reaction
A weakly basic condition (at pH values of ∼8–8.5) can trigger successive crosslinking between phenols and amines to enhance the cohesion of catechol or pyrogallol moiety containing adhesive.74 Although the catechol–amine reaction type is recognized as either a Michael addition or a Schiff base process, it is still uncertain which mechanism is prevalent in a given case.75 The work reported by Qiu et al. suggested that Michael addition occurred under aerobic and mild alkaline conditions with both primary and secondary amines preferring to link with catechol to form oligomers. According to the results of molecular simulations, Michael addition products are prevalent for both aromatic and aliphatic amines with catechol, supporting the fact that the corresponding co-deposited coatings are durable under various operating environments.76 Whereas in the presence of strong oxidants (e.g. NaIO4), TA can be oxidized to yield a quinone-rich structure that is able to react with amines following the Schiff base mechanism.77
The products of the phenol/amine Michael/Schiff base reaction have been recognized as valuable in versatile applications because of their uniform coating or surface modification property.78 In contrast to dopamine (DA), TA and polyamines are much more stable in an ambient atmosphere, possessing the features of low cost and convenient storage. Furthermore, this reaction strategy is prevalent in biomimicking membranes since it has non-toxic or low-cost raw materials, controllable crosslinking density and rapid deposition.79 In an appropriate case, Zhang et al. demonstrated that TA are capable of forming coatings similar to polydopamine (PDA) on various surfaces via co-deposition with amino-containing polymers or organic molecules (Fig. 7a).80 In detail, polyphenol coatings were obtained by the phenol/amine Michael reaction between TA and diethylenetriamine (DETA) on polysulfone ultrafiltration membranes for improved water wettability and enhanced nanofiltration performance. With the aim of improving the adsorption capacity of heavy metal ions, Huang et al. modified MgAl-layered double hydroxide (LDH) via co-deposition of polyethylene polyamine (PP) and tannic acid (TA) (Fig. 7b).81 With its combination of polyphenol chemistry and nitrogen-rich binding sites, the as-prepared LDH-PP@TA could be used to adsorb copper(II) ions from wastewater.
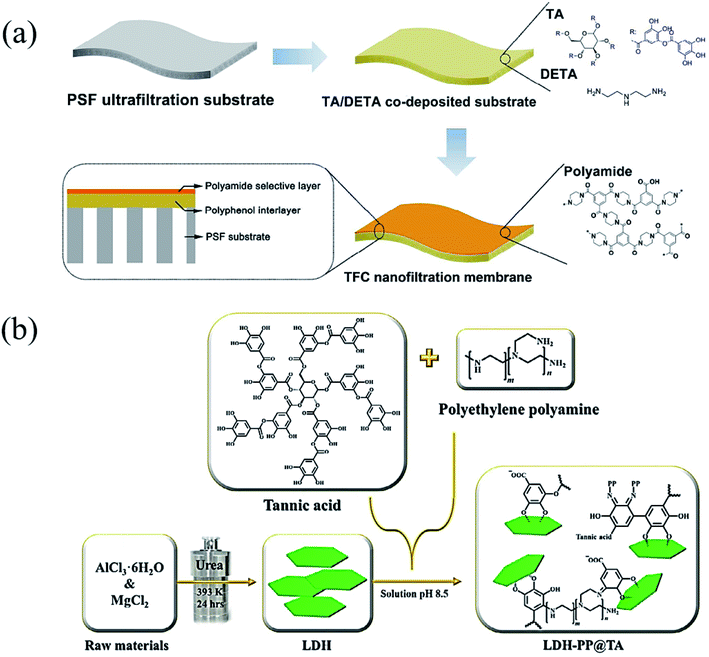 |
| Fig. 7 (a) A polyphenol interlayer coated onto a polysulfone (PSF) ultrafiltration membrane. Reproduced from ref. 80 with permission from the American Chemical Society, copyright 2016. (b) TA and polyethylene polyamine were co-deposited onto MgAl-layered double hydroxide (LDH). Reproduced from ref. 81 with permission from the Elsevier, copyright 2018. | |
Natural biopolymers such as silk fiber (SF), gelatin, lysine and chitosan are polyamides that could also be modified by tannic acid via the phenol–amine Michael/Schiff base reaction. The incorporation of TA as a commercially available chemical crosslinker, molecular glue, and antibacterial agent granted polymer–TA conjugates with a bioadhesive property.82 In the work reported by Pang et al., low-cost dopamine analogues (alkali lignin as well as TA) were used to develop a reinforced silkworm SF via a Schiff base reaction between polyphenol moieties and amine groups, which formed a network mimicking “reinforced concrete” (Fig. 8a).83 As a result, the lignin-coated and TA-coated SFs are preferable for strengthening SF due to the more condensed crosslinking sites as well as lower cost in contrast to PDA. TA-modified gelatin bioadhesives had advantages such as ready scalability, relatively low cost and the elimination of concerns about neurological side-effects caused by DA. Recently, Ge fabricated dual cross-linked gelatin-oxidized tannic acid–FeCl3·6H2O (DC-GT/OTA/FeIII) hydrogels in which phenol–amine and MPN crosslinking strategies were used.84 Moreover, Guo et al. designed a facile, straightforward Michael addition reaction method to produce TA-based bioadhesives, in which tannic acid and gelatin interacted under oxidizing conditions.85 Similarly, Zhao et al. created TA-modified gelatin hydrogels via both supramolecular interactions and covalent bonding under a strong oxidant (Fig. 8b).86 The outstanding viscoelasticity, thermal sensitivity, and macroporosity granted the present hydrogel promising application prospects in wound healing, tissue adhesion and drug loading.
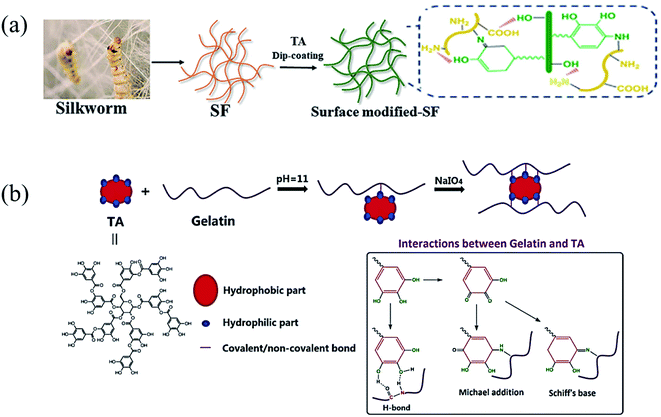 |
| Fig. 8 (a) Silk fiber was modified by TA. Reproduced from ref. 83 with permission from Wiley-VCH, copyright 2020. (b) Crosslinking mechanism of oxidated TA and gelatin under alkaline conditions. Reproduced from ref. 86 with permission from Wiley-VCH, copyright 2019. | |
3.2. Phenol–thiol Michael addition
Polyphenol chemistry accounts for the improved adhesion, macromolecular network formation, and mechanical strengthen in reference to mussel adhesive proteins.87 Catechol or pyrogallol groups could be easily transferred to o-quinone in the presence of an oxidase or in alkaline seawater.88 Nucleophilic reactions such as quinone–phenol dismutation, Schiff base reaction or Michael addition occur between o-quinone and various substrates containing catechol, amine or thiol groups.89,90 The phenol–thiol Michael addition between TA and thiol-bearing polymers has generally been utilized in the construction of antifouling membranes or surfaces with desired properties.91
In an effort to reduce environmental pollution, polymer brushes, whether hydrophilic or amphiphilic, have been designed to construct nondepleting coatings for antifouling membranes.92 Therefore, the development of new surface functionalization techniques with green chemistry and easy processing is in line with current interests and concerns. In comparison to dopamine or dopa-derivatives, TA is generally widely acknowledged as safe (GRAS) by the U.S. Food and Drug Administration (FDA), which ensures a “greener” surface-modification technique.93 In the work proposed by Pranantyo et al., multilayer polymeric layer anchors on stainless steel substrates were achieved by deposition of TA and then tethered hyperbranched polyglycerols bearing terminal thiol moieties (HPG-SH).94 In terms of qualitative and quantitative assays of settlement of the microalgae Amphora coffeaeformis, this polymeric anchor was proved to have low fouling characteristics. A similar method could be seen in the work provided by Xu et al.95
3.3. Phenol–epoxy ring opening reaction
In an age of rapid petroleum consumption, the world is currently encountering issues such as climbing oil prices, the greenhouse effect and stacks of nondegradable plastic. People urgently need to explore “greener” industry for sustainable uses.96 However, until now petrochemically-derived acrylate polymers have been used predominantly in many applications, which have been predicted to be exhausted in the foreseeable future.97 Reproducible resources such as degradable chemicals and oils originating from plants have already been used in the tannery industry both as resins by self-polycondensation and as raw material crosslinkers for coating resins. TA, a low-cost compound, possesses multiple chemical binding sites as well as multiple aromatic rings, ensuring a high Tg green epoxy resin.98–100
Previous studies have proved that under certain heating conditions, the pyrogallol groups on TA are capable of triggering ring-opening of oxirane rings, making TA a chemical hardener for epoxy material.101,102 Recently, Feng et al. proposed a facile method to prepare a TA-hardened epoxy resin, in which TA was applied as a multifunctional crosslinking agent for diglycidyl ether of bisphenol A (DGEBA) (Fig. 9).103 Previous researchers revealed that the reactivity of the outer-layer hydroxyl groups in TA have a higher chance of reacting with oxirane rings than inner-layer hydroxyl groups because of the steric hindrance of the dendritic structures of TA.98 Thanks to the multiple binding sites and special topological structure of TA, the resulting epoxy thermosets demonstrated a hierarchical network structure with desirable mechanical strength and tunable functionalities, such as shock absorption, shape memory, and sustainable uses. Similar work has also been proposed by Handique et al.104 and Baruah et al.,105 which revealed that TA could potentially replace novolac resins in a green way and alleviate the health and environmental burdens of undegradable epoxy material synthesis.
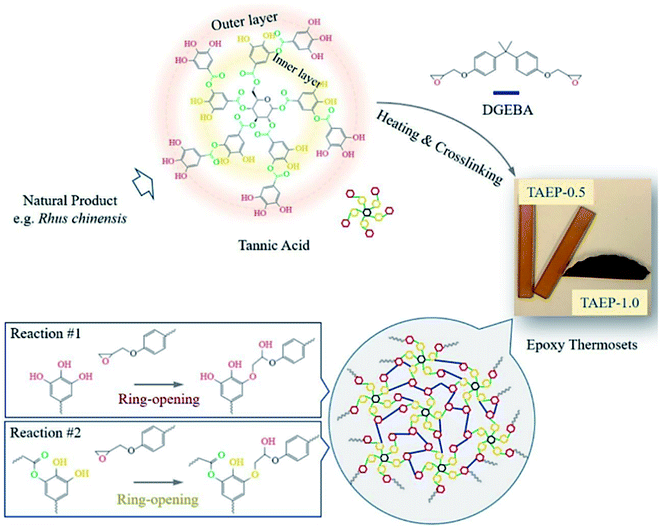 |
| Fig. 9 The polymerization between TA and epoxy monomer (DGEBA) to produce tunable thermosets. Reproduced from ref. 103 with permission from the American Chemical Society, copyright 2020. | |
3.4. Silanol–phenol condensation
Organic–inorganic hybrid polymers could be obtained via silanol–phenol condensation. This process normally consists of two steps: (i) silane/siloxane precursors are first hydrolyzed to form silanol in water. (ii) Silanol reacts with the hydroxyl groups of polyphenols. In the earliest studies, silanol–phenol polymeric networks were used for fabricating superhydrophilic organic–inorganic hybrid membranes via the co-deposition of polyphenols and silanes.106 Furthermore, Sa et al. proved that silanol would tend to react with the hydroxyl groups of dopamine, which are much more active than the hydroxyl groups belonging to PDA.107 Analogous to dopamine, the hydroxyl groups of TA are able to react with the Si–OH groups of silanol under mild conditions.
In the work of He et al., TA was applied as a stabilizer to disperse graphene in aqueous solution via π–π stacking to form graphene–TA hybrids. Subsequently, γ-(2,3-epoxypropoxy) propyltrimethoxysilane (KH560) was added to modify the surface of graphene–TA via silanol–phenol condensation to obtain an organic–inorganic hybrid anti-corrosion coating.108 Inspired by the phenomenon that polyphenols in red wine or tea tend to deposit onto flexible surfaces or given containers, an alcohol precursor γ-(2,3-epoxypropoxy) propyltriethoxysilane (KH561) was involved in constructing the polyphenol–silanol network. Chen et al. developed TA-KH561 copolymer (TA561) under mild conditions for a strong adhesion material and wound healing (Fig. 10).109 Under continuous heating, a sol–gel transition occurred to form solid TA561 because of the formation of a TA crosslinked polysiloxane network. TA561 copolymer could not only be applied as an adhesive for materials in daily use, but also manifested antibacterial activity in the wound healing process.
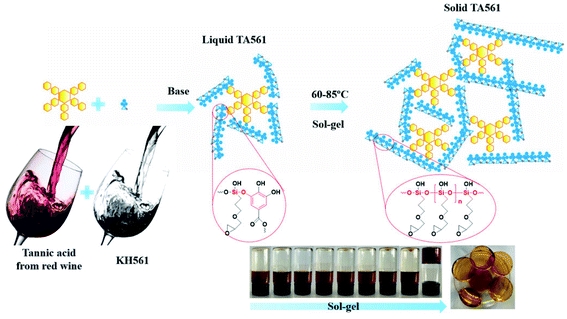 |
| Fig. 10 Synthetic production of TA-KH561 copolymer under mild conditions. Reproduced from ref. 109 with permission from the Royal Society of Chemistry, copyright 2021. | |
3.5. Boronate–phenolic network (BPN)
Currently, dynamic covalent interactions have been extended to prepare hydrogels with programed degradation and cargo release behaviour by exploiting the stimuli-responsive nature of dynamic covalent bonds.110–112 For instance, boronic esters are normally synthesized via the esterification reaction between boronic acids and cis-1,2 or cis-1,3 diols, which could reversibly dissociate under acidic conditions.113 It has been proved that boronic esters are stable under physiological conditions with no or extremely little side effects.114 Because of the favorable syn-peri-planar configuration of the aromatic hydroxyl groups as well as their electron-donating features, phenolic compounds are excellent building blocks to construct boronate ester networks.115 Coincidentally, TA usually consists of a central glucose surrounded by 10 galloyl units end-capped with aromatic triols, and the tip of the branch units feature either a catechol (1,2-diol) or a 1,3-diol. It is reasonable to construct copolymers via the reaction between TA and boronic acid derivatives.
Several research groups have reported a series of pH-responsive capsules prepared by the multistep layer-by-layer (LbL) assembly of boronate-functionalized macromolecules with polyelectrolytes.116,117 However, none of these capsules are stable and responsive to cis-diols under alkaline pH environments (e.g., pH 9–11), which is an obstacle in practical medical applications. Facing this issue, Guo et al. reported a dual-responsive capsule system toward pH and cis-diol through the boronate–phenolic network formed between phenylboronic acid (BDBA) and a polyphenol (TA) on particulate templates.118 At alkaline pH, the BDBA–TA composites turned out to be stable boronate ester networks. Exploring the pH responsiveness and dynamic nature of complexes between boronic acid-terminated PEG and polyphenols, Huang et al. created injectable BPN hydrogels (Fig. 11).119 In this procedure, the polyphenol acted both as therapeutic agent and crosslinker, and a sol–gel transition occurred when a precursor solution containing boronic-acid-terminated polymer and polyphenol was injected in vivo. Additionally, Montanari et al. developed a pH-responsive, hyaluronic acid (HA)-based nanoparticle system for the encapsulation of TA.120 Above all, these BPN-based materials are promising for application as smart, long-acting, and targetable therapeutic agents for cancer.
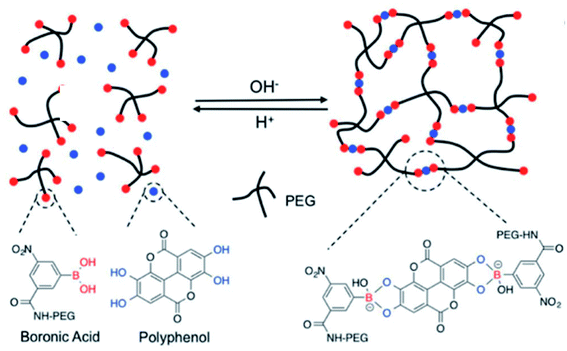 |
| Fig. 11 Dynamic nature and pH-responsive network formed between boronic acid-terminated PEG and polyphenols. Reproduced from ref. 119 with permission from the Royal Society of Chemistry, copyright 2018. | |
3.6. Phenol–alcohol Mitsunobu reaction
The Mitsunobu reaction, essentially a significant dehydration reaction, generally involves the coupling between a primary or secondary alcohol and an acidic pronucleophile (NuH) catalyzed by a redox combination of a trialkyl or triarylphosphine and a dialkyl azodicarboxylate. This reaction has gained extensive use in various synthetic procedures because of its wide scope, stereospecificity and mild reaction conditions.121 TA, with a pKα of 10, could be exploited as an acidic nucleophile as well as a crosslinker. Chen et al. proposed a covalent-crosslinking method in which TA was subjected to direct PEGylation via Mitsunobu polymerization (Fig. 12).122 This phenol–alcohol reaction was conducted under mild conditions (0–25 °C) in the presence of PPh3/diisopropyl azodicarboxylate (DIAD). TA–PEG hydrogel could not only chelate FeIII to form a composite hydrogel, but also had the ability to reduce AuIII and AgI ions rapidly within 5–10 min to produce the corresponding nanoparticles. Antimicrobial measurements suggested that TA–PEG hydrogel as well as TA–PEG–AgNPs hydrogel showed excellent antibacterial activity against S. aureus and E. coli.
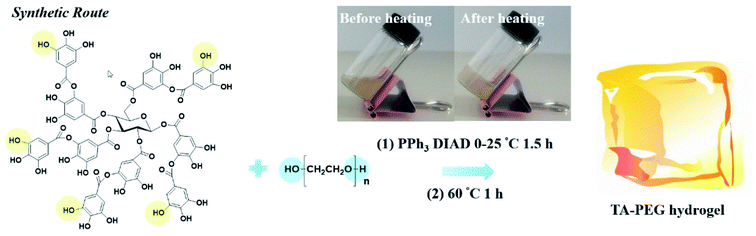 |
| Fig. 12 Direct PEGylation of TA under mild conditions to construct a TA–PEG hydrogel. Reproduced from ref. 122 with permission from the Royal Society of Chemistry, copyright 2021. | |
3.7. Phenol–aldehyde reaction
The pyrogallol groups of TA can also be crosslinked by formaldehyde just like phenolic resin. Wei et al. reported a method leading to TA-formaldehyde microspheres with uniform diameter as electrode materials (Fig. 13).123 Similarly, with the aim of removing pollutants from industrial wastewater, Zhang et al. synthesized a FeIII–TA-formaldehyde polymer via a one-pot hydrothermal method, in which FeIII and formaldehyde were utilized as dual crosslinkers.124 Owing to the polyphenol moieties, FeIII–TA-formaldehyde particles could efficiently trap methylene blue, PbII, and HgII from aqueous solution with rapid adsorption kinetics. Apart from the above TA-formaldehyde crosslinking method, Chen et al. employed TA-glutaraldehyde crosslinking for surface modification of melamine foam.125 The nucleophilic centers of TA allow it to react with glutaraldehyde, providing the possibility of achieving a stable network as well as enhancing surface roughness.
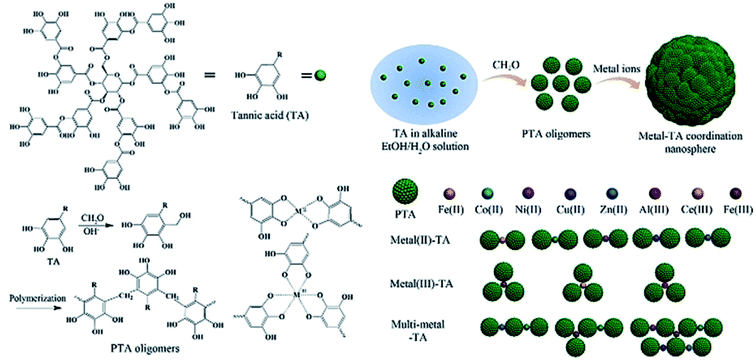 |
| Fig. 13 The synthetic procedure and the proposed precipitation mechanism of TA-formaldehyde microspheres. Reproduced from ref. 123 with permission from Wiley-VCH, copyright 2018. | |
3.8. Acylation of polyphenols
The acylation process incorporates the activation of acid groups belonging to polymer or monomer by a catalyst or continuous heating, followed by dehydration between the activated acid groups and hydroxyl groups.126 In the work of Sahiner et al., N,N′-carbonyldiimidazol was found to be an efficient catalyst for acylation of TA, offering some plus points including mild reaction conditions, very few byproducts, and low cost.127 The acylation of polyphenols can also proceed without a catalyst. Recently, Guo et al. proposed that, under specific crosslinking conditions (80–120 °C, 1–3 days), the hydroxyl groups of TA can bridge citrate to obtain stable tannin-bridged hydroxyapatite (HA) bone composites for lumbar fusion.128 Combining the merits of TA and citrate components, the obtained citrate-based tannin-bridged bone composites demonstrated increased compression strength, prolonged degradation behaviour, enhanced biomineralization performance, excellent biocompatibility, and low cellular toxicity as well as considerable antibacterial properties.
3.9. Polyphenol–thiyl radical oxidation reaction
It should be noted that the reducing activity of TA is based on consecutive radical reactions, such as radical scavenging of harmful radicals originating from biomacromolecules under high temperature or ultraviolet irradiation. New stable compounds come into being via radical-scavenging reactions between TA and radical species derived from food or metabolic products.129 Generally, TA was found to be applicable as a cooking ingredient for protein, in which a new polymeric network was formed by the breakage of the disulfide bonds and a polyphenol–thiyl radical oxidation reaction. Typically, improved dough properties could be achieved when the amount of TA added reached 0.03 g kg−1 in bread making.130 Above all, it can be concluded that the integration of TA and sulfur-rich molecules is a potential route to obtain crosslinked polymers for biomedical applications. Integrating the advantages of TA and polysulfur, Chen et al. synthesized a tannic acid–thioctic acid (TATA) hydrogel through a polyphenol–thiyl radical oxidation reaction (Fig. 14).131 Owing to the chemical crosslinking as well as supramolecular assembly of the TATA matrix, the TATA hydrogel exhibited strong tissue adhesion, desirable biocompatibility, and biodegradability with minimal inflammatory response. Thus, the TATA hydrogel is promising for use as an injective tissue adhesive.
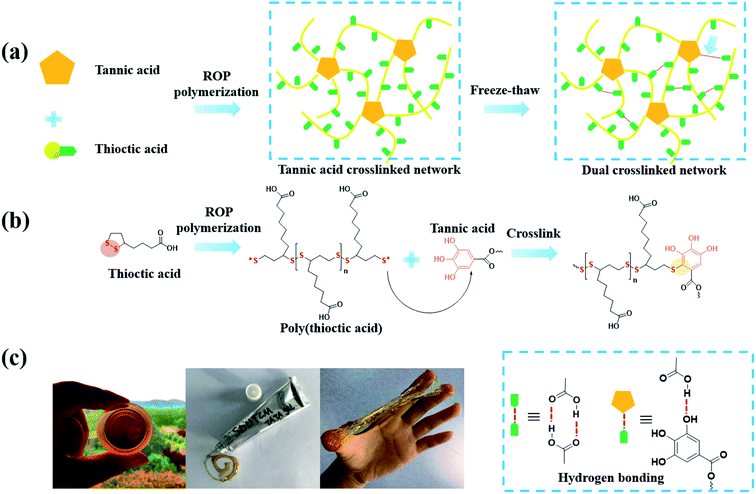 |
| Fig. 14 (a) A schematic illustration of the synthetic route of TATA hydrogel; (b) Mechanism of the polyphenol–thiyl radical oxidation reaction; (c) photos of the TATA hydrogel. Reproduced from ref. 131 with permission from the Royal Society of Chemistry, copyright 2021. | |
4. Applications of tannic-acid-crosslinked networks
The utilization of TA-functionalized networks can be found in various studies and the corresponding material categories cover hydrogels, nanoparticles, injectable adhesives, coatings, microspheres and so on. Thanks to the flexible crosslinking method, tunable binding substrates and multifunctional properties of polyphenols, TA-based materials have recently been extensively applied in research areas such as bone regeneration, skin adhesives, wound dressings, drug loading and photothermal materials (Table 2). In this section, TA-crosslinked networks with multiple crosslinking strategies are introduced, which play a critical role in material performance.
Table 2 Applications of TA-crosslinked networks
Application |
Composition |
Crosslinking method |
Ref. |
Bone regeneration |
TA–hydroxyapatite-collagen-peptide |
Hydrogen bonding, MPN network and hydrophobic interaction |
138 |
TA–hydroxyapatite–silk fiber |
Hydrogen bonding and MPN network |
139 |
TA–calcium alginate-peptide |
Hydrogen bonding and MPN network |
140 |
Skin adhesive for coagulation |
TA–PVA–polyacrylamide |
Hydrogen bonding |
144 |
TA-PAAm-kaolin |
Hydrogen bonding, MPN network and radical oxidation |
146 |
Skin adhesive for UV resistance |
TA–poly(glutamic acid) |
Hydrogen bonding |
147 |
Wound healing |
TA–poly(thioctic acid) |
Hydrogen bonding and radical oxidation |
131 |
TA–gelatin–gellan |
Hydrogen bonding |
152 |
TA–PEG |
Hydrogen bonding |
153 |
TA–hydroxypropyl chitin–FeIII |
Hydrogen bonding and MPN network |
154 |
TA–silk fiber–peptide |
Hydrogen bonding and hydrophobic interaction |
155 |
TA–chitosan–silk fiber–FeIII |
Hydrogen bonding and MPN network |
156 |
Drug loading |
TA–indocyanine green–DOX |
π–π interaction and electronic interaction |
166 |
TA–MSN–tetraethylenepentamine–DOX |
Michael addition and Schiff base reaction |
167 |
TA–sericin–DOX–FeIII |
MPN network |
169 |
Photothermal material |
TA–FeIII/VIII/RuIII |
MPN network |
171 |
TA–wood–FeIII |
MPN network |
174 |
In situ generation of noble metal nanoparticles |
TA–graphene–AuIII |
π–π interaction and MPN network |
53 |
TA–graphene–AgI–polyaspartamide |
π–π interaction and MPN network |
45 |
TA–PEG–AuIII/AgI |
Mitsunobu reaction and MPN network |
122 |
Antifouling coating |
TA–diethlyenetriamine |
Michael addition |
80 |
TA–thiol end-capped hyperbranched polyglycerols |
Michael addition |
94 |
TA–glutaraldehyde–β-FeOOH-1-dodecanethiol |
Michael addition and phenol–aldehyde reaction |
125 |
4.1. Bone regeneration
Nowadays, scaffolds aimed at the restoration of injured tissues have been reckoned to be the main topic in the field of tissue engineering applications.132 Most musculoskeletal defects are caused by tumors, bone marrow diseases, external force, traffic accidents, battlefield injuries, and even bone birth defects, giving rise to degeneration of hyaline cartilage and subchondral bone.133 To overcome these problems, conventional devices such as metallic plates and screws usually suffer from acute rejection, aseptic loosening, and a second operation for removal.134 Though synthetic polymer-based 3D porous scaffolds including poly(methyl methacrylate) (PMMA) and calcium phosphate (CPC) bone cements have aroused more and more interest as alternative treatments, several drawbacks have emerged, such as low levels of osteogenesis, poor biocompatibility, and toxic degradation products as well as poor adhesion.135 TA can be used as a bioactive component in fabricating a tissue engineering material with desirable antioxidant and anti-inflammatory properties. Thanks to its glue-like properties, including metal chelation, hydrogen bonds and π–π stacking, TA can form networks with proteins, hydroxyapatite, and naturally derived reinforced polymers to form hierarchical microstructures with appropriate adhesion performance in a physiological environment.136,137 Recently, multiple findings have suggested that TA is an effective surface modification intermediate and crosslinking aid in bone regeneration.
Based on the viewpoint that TA plays a role as a biocompatible multisite crosslinker for collagen as well as a growth-factor-delaying ingredient, Lee et al. designed new bone regeneration scaffolds composed of TA, calcium-deficient hydroxyapatite (CDHA), collagen, and plate-rich plasma (PRP) fabricated via a low-temperature printing process.138 Controlled release of the growth factors from this biocomposite was observed up to 35 days due to gradual degradation of the TA-based network. The cell proliferation and bone mineralization of this bone regeneration scaffold were substantially better than those without TA modification. Similar work on TA–hydroxyapatite composites was also exhibited by Bai et al., in which phenolic chemistry was used as a robust synthetic protocol for fabricating a bone adhesive with low toxicity and high mechanical performance containing silk fibroin (SF), TA and hydroxyapatite (SF@TA@HA) (Fig. 15).139 Typically, TA assembled with SF to form a nano-fibrillar structure with β-sheet-rich architecture, further strengthened by Ca2+–phenolic coordination bridges. This composite accelerated the regeneration of bone defects at the initial stage in vivo with strong water-resistant fixation. Inspired by the phenomenon that TA could form additional coordinate bridges with calcium ions belonging to Ca-alginate (CA) nanoparticles,140 Zhang et al. created a composite scaffold fabricated by prime-coating Ca-alginate hydrogel with TA followed by loading peptides that were beneficial for osteogenesis.141 A TA prime-coating process significantly increased the stability and mechanical properties of the scaffold, facilitating the immobilization of peptides for continuous delivery with an ignorable effect on tissue bioactivity.
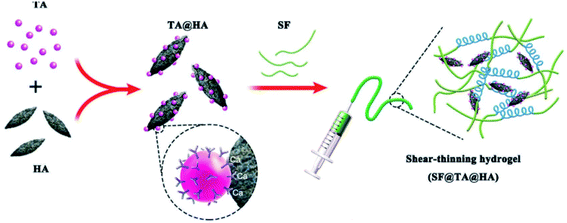 |
| Fig. 15 TA-based adhesive hydrogel for bone regeneration. Reproduced from ref. 139 with permission from Wiley-VCH, copyright 2019. | |
4.2. Skin adhesive
A protein-rich surface such as skin contains abundant functional groups, such as amide bonds, thiols and amines, which facilitates polyphenol-based coating through Michael addition and hydrogen bonding. TA has been employed as a pivotal component for the design of multifunctional coatings and adhesive hydrogels for skin adhesion. Though the TA-based adhesives had a relatively low adhesive strength compared with PDA, they exhibited outstanding advantages in terms of low cost, biocompatibility, cell adherence and desirable drug loading capacity.142,143 Moreover, owing to the polyphenol adhesion mechanism, TA-based adhesives exhibited water resistance and wet skin adhesion during practical uses, adapting to dynamic physiological conditions such as bleeding or leaching.144
TA-based adhesive hydrogels normally match the skin surface excellently with no skin sensitization or irritation. A combination between TA and a mechanical reinforcing agent gives rise to a hybrid adhesive with excellent and repeatable adhesion to versatile substrates.145 Based on this concept, Fan et al. developed a PAAm-TA-kaolin (KA) hydrogel for quick hemostatic application on bleeding skin.146 In detail, TA served not only as a chemical crosslinker but also as a polyphenol donor for wet adhesion. Meanwhile, KA played roles as a physical crosslinker as well as a hemostatic accelerator. The dual-crosslinked PAAm-TA-KA hydrogel possessed high toughness and universal adhesiveness. The hemostatic time of this hydrogel on a rat femoral artery model was as short as 24 s in contrast to 148 s of the control group. Similar work on PAAm-TA complexes was provided by Fan et al.144 Recently, a new dual-network hydrogel sunscreen (DNHS) based on poly-γ-glutamic acid (γ-PGA) and TA was developed by Wang et al. (Fig. 16).147 The driving force of gelation was induced by the intermolecular hydrogen bond between γ-PGA and TA, which endowed the hydrogel with remarkable self-recovery properties (within 60 s), excellent skin-integration, water resistance capacities and low peel strength. Considering the UV absorption characteristics of TA, this hydrogel demonstrated prominent UV skin protection performance across the broad UV region (360–275 nm).
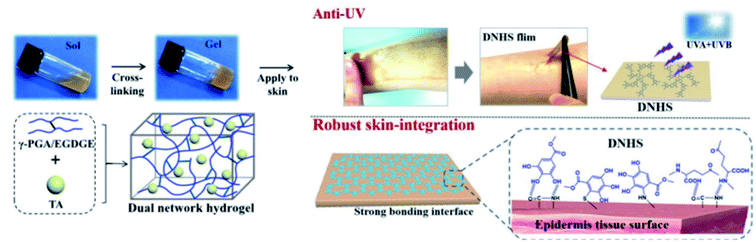 |
| Fig. 16 Chemical crosslinking structure of a dual-network hydrogel sunscreen (DNHS) and its skin adhesion mechanism. Reproduced from ref. 147 with permission from the American Chemical Society, copyright 2019. | |
4.3. Antibacterial biomaterial for wound healing
The application of TA in wound healing can be traced back to the 1920s since TA–protection of lesion location from microbe or virus intrusion.148 Since TA could interact with various biomacromolecules through supramolecular interactions, the antibacterial mechanism of TA is potentially associated with the cell wall complexation or membrane disruption initiated by oxidized TA.75 Accompanied by the discovery of mussel-inspired coatings, there has come about a renewed viewpoint on the efficacy of TA as an adjuvant therapy for antibacterial wound dressings. Currently, other wound dressings including silk fibroin (SF), gellan, chitosan, hyaluronic acid (HA), collagen and alginate are also being developed for therapeutic treatment.149 However, the use of toxic crosslinkers or organic solvents during the modification process poses an issue for long-term safety.150 To meet diverse demands, such as adhesion ability, flexibility, physical or chemical tunability of wound dressings, it is reasonable to employ TA as a green crosslinker for biomacromolecules or polymers, achieving multifunctionality and multitargeted uses.151
As for TA-based injectable hydrogels for wound healing, Zheng et al. verified that TA-loaded gellan hydrogel could accelerate wound healing in contrast to control groups.152 Moreover, Sun et al. fabricated a self-healing injectable adhesive via a supramolecular interaction between TA and eight-armed PEG end-capped with succinimide glutarate active ester (PEG-SG) (Fig. 17).153 Taking advantage of the hydrogen bonding induced by PEG segments and phenolic hydroxyl groups, as well as the ester exchange induced by succinimide and the tissue amino groups, this adhesive was capable of adhering to porcine tissues and sealing the rigid vascular artery. Ma et al. recently developed a thermal/pH responsive chitin/TA/ferric ion (HPCH/TA/Fe) composite hydrogel.154 TA played roles not only as a crosslinker to improve mechanical properties, but also as an antibacterial agent sustainably released under acidic circumstances. The relevant examinations revealed that this hydrogel could efficiently resist wound infection while accelerating the healing process with appendage-filled and scarless skin.
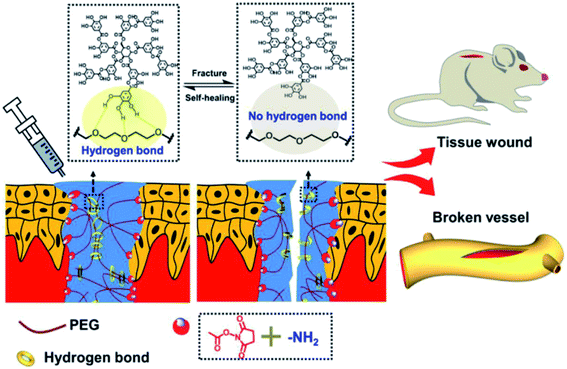 |
| Fig. 17 Schematic exhibition of the PEG-SG/TA injectable hydrogel and its application in wound healing. Reproduced from ref. 153 with permission from the American Chemical Society, copyright 2020. | |
Hydrogel patches or self-supporting 3D hydrogels are also recognized as an effective choice in clinical wound healing, especially those prepared from natural building blocks. For instance, the combination between TA and SF was constantly applied in superficial wound dressings. Jing et al. explored a TA-induced gelation method for SF via multiple interactions such as hydrogen bond association, hydrophobic interactions and π–π interactions.155 Acceleration of the wound healing process was observed by a full-thickness skin defect model on mice, revealing the fact that the incorporation of TA endowed the hydrogel with desirable bioactivity. Similar work was reported by Yu et al.156 They designed a hybrid hydrogel composed of chitosan, silk fibroin, TA and ferric ions for photothermal therapy in wound healing. The spongy structure of the concerned hydrogel enable it to absorb large capacities of blood and tissue exudate, which was effective for bleeding wounds.
In particular, micro-biomedicine for wound healing is also attractive because of its extensive specific surface area, deep penetration and accurate quantification.157 Instead of using toxic epichlorohydrin or polyphosphate as a crosslinker, TA can be used to stabilize HA microparticles for wound healing, as reported by Li et al.158 This kind of hemostatic microparticle was promising in clinical applications because of its wide spectrum antibacterial activity against both Escherichia coli and Staphylococcus aureus. Notably, the wound can be repaired rapidly by TA-functionalized HA microspheres in 14 days.
4.4. Drug loading vehicle
So far, cancer has always been one of the main threats to human health.159 Chemotherapy combined with nanotechnology is the most frequently used solution with prolonged blood circulation time, enhanced safety and intracellular drug release.160–162 TA was also involved in constructing smart, biodegradable, highly chemically tunable nanovehicles via multiple supramolecular interactions with payloads or other building units. As seen in Sections 2.1 and 2.3, TA-based polymer networks are generally pH sensitive and have the ability to reconstruct under neutral or alkaline conditions, facilitating on-demand or controlled drug release in vivo.68 Meanwhile, TA can promote the loading capacity of DOX via π–π interactions and TA also provides reactive sites for binding a targeting ligand, which enhances therapeutic efficiency and lowers toxic side effects.163
Nowadays, considering the fact that the tumor site has a weakly acid microenvironment, pH-responsive release has become the most widely used application.164,165 Deng et al. constructed a hybrid nano-transformer containing DOX, TA and indocyanine green, which was named DTIG. In this nanomaterial, π–π interactions and electronic interactions were the driving force for self-assembly (Fig. 18a).166 In this DTIG system, TA significantly reduced the nonspecific binding of nanovehicles, realized controlled release in blood circulation and avoided phagocytosis of the reticuloendothelial system (RES). In the lysosomal environment (pH 4.5), DTIG reassembled into a giant nanoreassembly (1.5 μm) in association with a hydrophobic–hydrophilic conversion, which gave rise to rapid lysosome escape and accurate intracellular payload release. Chen et al. also created a pH-responsive drug delivery system by applying TA as a “gatekeeper”.167 In brief, TA and tetraethylenepentamine (TEPA) were polymerized through a Schiff-base/Michael addition reaction to form a pH-sensitive shell, which was coated on the surface of mesoporous silica nanoparticles (MSNs) (Fig. 18b). The TA–TEPA binary coating shell could dissociate into a loose state under acidic conditions for drug release. When the surroundings returned to alkaline or neutral, the TA–TEPA shell reassembled again to stop drug discharge. Additionally, TA/ferric ion (Fe3+) MPN capsules were also applied in smart, pH-dependent drug delivery for cancer cells, as reported by Zhu et al.168 and Liu et al.,169 which effectively prevent premature drug leakage in healthy tissues while unloading payloads in feedback to the acidic tumor and intracellular-microenvironments.
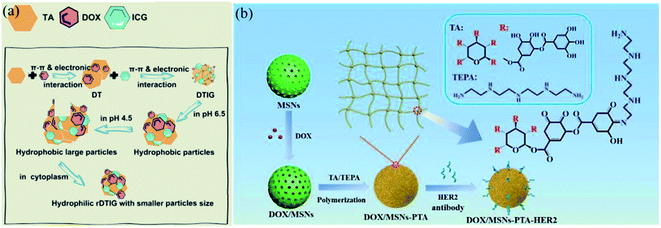 |
| Fig. 18 (a) The construction of a TA-based nanotransformer (DTIG) and its controlled release under acidic environments. Reproduced from ref. 166 with permission from the American Chemical Society, copyright 2020. (b) Drug delivery process of TA-coated MSNs for accurate cancer treatment. Reproduced from ref. 167 with permission from the Royal Society of Chemistry, copyright 2020. | |
4.5. Photothermal material
TA-based MPN networks for seawater desalination, cancer therapy, solar harvesting and near infra-red (NIR) light-induced actuation have aroused wide interest due to their high stability, facile synthetic procedure, biosafety, biocompatibility and high photothermal conversion rate.170,171 Moreover, MPNs can effectively adsorb various aromatic molecules, ready for versatile chemical modifications.172 Researchers have proved that the photothermal conversion mechanism lay in the ligand-to-metal charge transfer between multivalent metal ions and TA.173 Wang et al. confirmed that FeIII–TA complexes achieved the highest NIR absorbance in contrast to other nitrophenols, including gallic acid, pyrogallol and epigallocatechin gallate (EGCG), probably because TA possessed more vicinal diol groups and had a better chance of coming into contact with FeIII.174
Numerous studies stated that the TA/FeIII complex was promising in the desalination of seawater. Recently, He et al. proposed a simple, mild and versatile method for preparing photothermal wood toward solar steam generation (Fig. 19).175 They found that TA and Fe3+ can effectively transform different kind of wood into photothermal material (wood–TA–FeIII), which could be realized in aqueous phase at room temperature without any high-cost metals. Such wood exhibits outstanding thermal performance, such as corrosion-resistance, long-term anti-rinsing and durable service. Similar work could be seen in the work undertaken by Wang et al. using a TA/PVP/FeIII complex coated onto a polyurethane sponge.176 Recent progress on photothermal therapy for tumors was shown by Shi et al.,177 in which a TA/FeIII complex coated mesoporous silica nanoparticle (MSN-pTA) was designed. Under 808 nm laser irradiation, dissociation of the TA/FeIII complex occurred and the loaded DOX inside MSN was released, achieving combined chemo-photothermal therapy. Chen et al. constructed TA-hybridized mixed valence vanadium oxide nanosheets (TA@VOx NSs), which possessed excellent NIR-light mediated conversion ability to inhibit tumors.178 In the case of solar harvesting, Huang et al. combined TA with liquid metals to realize photothermal materials with high efficiency, which manifested strong broad-band light absorption (96.9–99.3%) and excellent photothermal conversion ability (ηPT = 77.3%).179 In particular, TA-based MPN networks could also be utilized in (NIR) light-triggered actuation. As proposed by Wang et al.,180 a TA/WS2/polyurethane latex was coated onto a cellulose nanofiber film to form a bi-layer flexible device. Since the photothermal conversion performance of TA/WS2/polyurethane latex was more excellent than that of cellulose nanofiber film, the NIR-induced actuation could be realized based on the mismatch of thermal expansion between the two layers.
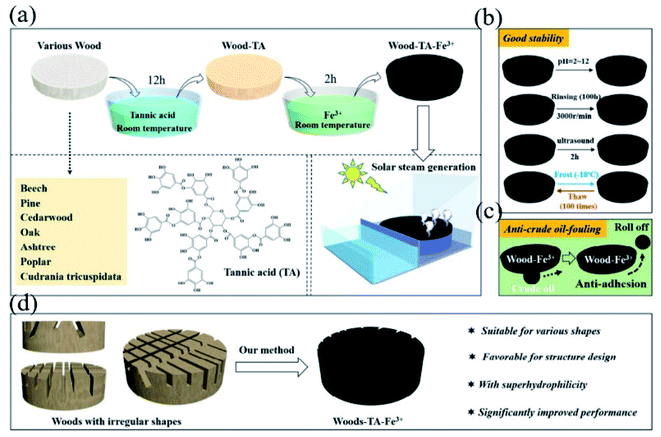 |
| Fig. 19 (a) Wood modified by an MPN network for solar steam generation; (b) wood with excellent corrosion-resistance; (c) anti-crude oil-fouling character of the resultant MPN-based photothermal materials; (d) exclusive advantages of the methods for enlarging the specific surface area of wood. Reproduced from ref. 175 with permission from Elsevier, copyright 2020. | |
5. Conclusions and future prospects
This review has systematically summarized various protocols leading to tannic-acid-based materials with a wide scope of functions and applications. The underlying reason why tannic acid is prevalent for building versatile polymeric networks lies in the multi-pyrogallol moieties as reactive binding sites. According to various natural inspirations as well as research findings, tannic acid has previously been used as a crosslinking agent with proteins, polysaccharides, hydrogen bonding acceptors, polycations, metal ions and other functional molecules. In view of physical crosslinking, tannic acid could achieve supramolecular materials such as hydrogels, reductive graphene complexes and nanocapsules through hydrogen bonding, π–π stacking and metal–polyphenol interactions. Covalent bonding through chemical crosslinking enhanced the stability of the resulting poly(tannic acid). Molecules bearing amine, thiol, epoxy, boronate, alcoholic hydroxyl, anhydride, carboxyl moieties and thiyl radicals are capable of reacting with a polyphenol group whether in a Michael addition or in nucleophilic ways. Moreover, tannic acid is recognized as a low-cost dopamine analogue owing to its abundant natural resources, rapid deposition property and nontoxicity, which is promising in developing new functional materials through scale-up productions. Though other forms polyphenols, such as epigallocatechin-3-gallate, proanthocyanidins, caffeic acid, resveratrol and so on have received a lot of attention, they could not be used instead of tannic acid because of their high cost, low chemical stability and limited binding sites. Possessing biocompatibility, antimicrobial properties, smart self-assembly capability and reductive ability, tannic acid has aroused more and more attention in the fields of macromolecular biomaterials. Incorporating the special properties of tannic acid and other building blocks, a wide range applications of tannic-acid-crosslinked networks, including bone regeneration, skin adhesives, wound dressings, drug loading vehicles and photothermal materials, have been studied by numerous researchers recently. In future prospects, tannic acid also shows potential in combination with other new materials at the frontier of science, such as black phosphorus, MXene, and graphyne, to achieve wider fields of application.
The initial tannic-acid-crosslinked materials were mainly metal–phenol networks with various nanostructures. Over time, macroscopic three-dimensional materials containing tannic acid, especially adhesive hydrogels, have been developed in numerous studies, in which researchers found that hydrophilic polymeric substrates are necessary for constructing a long-range, highly hydrated network. The latest trends for a tannic-acid-based network could be summarized as follows: (i) new crosslinking methods have emerged, including phenol–alcohol reactions, acylation of polyphenols, polyphenol–thiyl radical oxidation reaction, silanol–phenol and phenol–epoxy ring-opening reaction. (ii) In practical applications, tannic-acid-crosslinked biomacromolecules, such as silk fibers, gelation, gellan, peptides and polysaccharides, have received widespread interest owing to their biocompatibility, biodegradability, adhesiveness, and mechanical reinforcement, as well as facile preparation. (iii) A combination of multiple crosslinking methods has become more and more common, which is beneficial for integrating the advantages of various raw materials. (iv) A macroscopic combination between tannic-acid-based materials and other flexible materials has been studied, in which a tannic-acid-based complex was used as a coating or a functional layer to form smart devices. (v) Besides the antimicrobial, antioxidant and anti-inflammatory properties of tannic acid, researchers have exploited other properties, such as UV adsorption, photothermal conversion and chemodynamic responsiveness. Above all the latest trends, a hybrid material that incorporates new substrates is the main theme of progress on tannic-acid-crosslinked networks.
However, challenges still exist and advances in this field are demanded. Firstly, the coacervation between tannic acid and polymers owing to rapid multisite binding has become an obstacle for developing new complexes with even better performance, which requires an accurate feeding ratio and pH regulation. Though basic conditions could resist the formation of coacervation, the oxidation progress of tannic acid could not be ignored. Secondly, tannic acid tends to bind randomly to hydrogen bonding acceptors, leading to an amorphous rather than long-range-ordered material with weak mechanical strength. In other words, a conjugated multidentate ligand with high molecular weight for tannic acid could further expand this research area in organic–organic frameworks, organic–inorganic hybrid frameworks and directionally solidified materials. Thirdly, other than synthetic polymer–tannic acid conjugates, the exact binding mechanism of polysaccharide–tannic acid conjugates has yet to be more thoroughly studied, considering the fact that there are numerous forms of polysaccharides in nature, which limits the utilization of polysaccharide–tannic acid in the preparation of hydrogels, 3D printing inks and organic films. Last but not least, the reaction between polyphenol and radicals features rapid kinetics and robust preparation, which provides a horizon for new functional networks. Therefore, further efforts on synthetic procedures, post-functional methods and the accurate design of building blocks leading to a TA-based network will continue to be of great interest.
Overall, in the past few decades, mussel-inspired catechol chemistry has added new life and vigor to tannic acid in building various polymeric complexes. In view of the rising severe environmental problems derived from the unsustainable petrochemical industry, tannic-acid-crosslinked functional polymeric networks provide a green platform with a facile, sustainable, wide source and abundant content manner. Although many mechanisms concerning the association and disassembly of tannic-acid-based materials still remain unclear or controversial, abundant research on tannic-acid-crosslinked functional polymeric networks has emerged recently, showing that this field is gradually deepening and expanding. It could be inferred that tannic-acid-based functional materials will continue to be of great interest for researchers, especially in biomedicine, nano-technology, catalysis and energy storage.
Conflicts of interest
There are no conflicts to declare.
Acknowledgements
The study was supported by the Health Commission of Shandong Province (Grant No. 202004010938).
References
- J. L. Guo, Y. Ping, H. Ejima, K. Alt, M. Meissner, J. J. Richardson, Y. Yan, K. Peter, D. v. Elverfeldt, C. E. Hagemeyer and F. Caruso, Angew. Chem., Int. Ed., 2014, 53, 1–7 CrossRef.
- Y. L. Liu, K. L. Ai and L. H. Lu, Chem. Rev., 2014, 114, 5057–5115 CrossRef CAS.
- Z. Nie, A. Petukhova and E. Kumacheva, Nat. Nanotechnol., 2010, 5, 15–25 CrossRef CAS.
- L. Xu, W. Ma, L. Wang, C. Xu, H. Kuang and N. A. Kotov, Chem. Soc. Rev., 2013, 42, 3114–3126 RSC.
- E. Munch, M. E. Launey, D. H. Alsem, E. Saiz, A. P. Tomsia and R. O. Ritchie, Science, 2008, 322, 1516–1520 CrossRef CAS.
- T. S. Sileika, D. G. Barrett, R. Zhang, K. H. A. Lau and P. B. Messersmith, Angew. Chem., Int. Ed., 2013, 52, 10766–10770 CrossRef CAS.
- S. Quideau, D. Deffieux, C. Douat-Casassus and L. Pouysegu, Angew. Chem., Int. Ed., 2011, 50, 586–621 CrossRef CAS.
- B. N. Singh, S. Shankar and R. K. Srivastava, Biochem. Pharmacol., 2011, 82, 1807–1821 CrossRef CAS.
- H. Ejima, J. J. Richardson and F. Caruso, Polym. J., 2014, 46, 452–459 CrossRef CAS.
- A. King and G. Young, J. Am. Diet. Assoc., 1999, 99, 213–218 CrossRef CAS.
- S. Zhao, S. C. Xie, X. L. Liu, X. M. Shao, Z. Zhao, Z. X. Xin and L. Li, J. Polym. Res., 2018, 25, 225 CrossRef.
- S. Z. Moghaddam, S. Sabury and F. Sharif, RSC Adv., 2014, 4, 8711–8719 RSC.
- C. Zhu, E. Chalmers, L. M. Chen, Y. Q. Wang, B. B. Xu, Y. Li and X. Q. Liu, Small, 2019, 15, 1902440 CrossRef.
- Z. Wang, S. Zhao, R. Song, W. Zhang, S. Zhang and J. Li, Sci. Rep., 2017, 7, 9664 CrossRef PubMed.
- A. E. Hagerman, K. M. Riedl, G. A. Jones, K. N. Sovik, N. T. Ritchard, P. W. Hartzfeld and T. L. Riechel, J. Agric. Food Chem., 1998, 46, 1887–1892 CrossRef CAS.
- Y. Du, W. Z. Qiu, Z. L. Wu, P. F. Ren, Q. Zheng and Z. K. Xu, Adv. Mater., 2016, 3, 1600167 Search PubMed.
- T. Yoshida, T. C. Lai, G. S. Kwon and K. Sako, Expert Opin. Drug Delivery, 2013, 10, 1497–1513 CrossRef CAS.
- F. Jehle, P. Fratzl and M. J. Harrington, ACS Nano, 2018, 12, 2160–2168 CrossRef CAS.
- U. T. Khatoon, G. N. Rao, M. K. Mohan, A. Ramanaviciene and A. Ramanavicius, J. Environ. Chem. Eng., 2018, 6, 5837–5844 CrossRef CAS.
- X. Huang, H. Wu, X. Liao and B. Shi, Green Chem., 2010, 12, 395–399 RSC.
- A. Sionkowska, B. Kaczmarek, M. Gnatowska and J. Kowalonek, J. Photochem. Photobiol., B, 2015, 148, 333–339 CrossRef CAS.
- V. Kozlovskaya, O. Zavgorodnya, Y. Chen, K. Ellis, H. M. Tse, W. Cui, J. A. Thompson and E. Kharlampieva, Adv. Funct. Mater., 2012, 22, 3389–3398 CrossRef CAS.
- S. Zhao, S. C. Xie, Z. Zhao, J. L. Zhang, L. Li and Z. X. Xin, ACS Sustainable Chem. Eng., 2018, 6, 7652–7661 CrossRef CAS.
- J. Fu, Z. Chen, M. Wang, S. Liu, J. Zhang, J. Zhang, R. Han and Q. Xu, Chem. Eng. J., 2015, 259, 53–61 CrossRef CAS.
- R. G. Andrade, L. T. Dalvi, J. M. C. Silva, G. K. B. Lopes, A. Alonso and M. Hermes-Lima, Arch. Biochem. Biophys., 2005, 437, 1–9 CrossRef CAS.
- D. E. Payne, N. R. Martin, K. R. Parzych, A. H. Rickard, A. Underwood and B. R. Boles, Infect. Immun., 2013, 81, 496–504 CrossRef CAS.
- J. Luo, J. Lai, N. Zhang, Y. Liu, R. Liu and X. Liu, ACS Sustainable Chem. Eng., 2016, 4, 1404–1413 CrossRef CAS.
- W. Chou, Y. Wang, K. Chen, J. Wu, C. Liang and S. H. Juo, Cell. Immunol., 2012, 273, 79–84 CrossRef CAS PubMed.
- I. Erel-Unal and S. A. Sukhishvili, Macromolecules, 2008, 41, 3962–3970 CrossRef CAS.
- F. Reitzer, M. Allais, V. Ball and F. Meyer, Adv. Colloid Interface Sci., 2018, 257, 31–41 CrossRef CAS.
- S. Hong, J. Yeom, I. T. Song, S. M. Kang, H. Lee and H. Lee, Adv. Mater. Interfaces, 2014, 1, 1400113 CrossRef.
- S. M. Burkinshaw and N. Kumar, Dyes Pigm., 2009, 80, 53–60 CrossRef CAS.
- Y. Lei, Z. Tang, R. Liao and B. Guo, Green Chem., 2011, 13, 1655–1658 RSC.
- M. Cencer, M. Murley, Y. Liu and B. P. Lee, Biomacromolecules, 2015, 16, 404–410 CrossRef CAS PubMed.
- V. Natarajan, N. Krithica, B. Madhan and P. K. Sehgal, J. Biomed. Mater. Res., Part B, 2013, 101, 560–567 CrossRef PubMed.
- C. Zhang, B. H. Wu, Y. S. Zhou, F. Zhou, W. M. Liu and Z. K. Wang, Chem. Soc. Rev., 2020, 49, 3605–3637 RSC.
- J. Saiz-Poseu, J. Mancebo-Aracil, F. Nador, F. Busqué and D. Ruiz-Molina, Angew. Chem., Int. Ed., 2019, 58, 696–714 CrossRef CAS PubMed.
- M. Rinaudo, B. Lardy, L. Grange and T. Conrozier, Polymers, 2014, 6, 1948–1957 CrossRef.
- T. Shutava, M. Prouty, D. Kommireddy and Y. Lvov, Macromolecules, 2005, 38, 2850–2858 CrossRef CAS.
- K. H. Hong, Polym. Bull., 2017, 74, 2861–2872 CrossRef CAS.
- W. W. Niu, Y. L. Zhu, R. Wang, Z. Y. Lu, X. K. Liu and J. Q. Sun, ACS Appl. Mater. Interfaces, 2020, 12, 30805–30814 CrossRef CAS.
- H. Y. Lee, C. H. Hwang, H. E. Kim and S. H. Jeong, Carbohydr. Polym., 2018, 186, 290–298 CrossRef CAS PubMed.
- X. C. Du, L. Wu, H. Y. Yan, L. J. Qu, L. N. Wang, X. Wang, S. Ren, D. L. Kong and L. Y. Wang, ACS Biomater. Sci. Eng., 2019, 5, 2610–2620 CrossRef CAS.
- C. Y. Liu, H. Y. Liu, K. Y. Tang, K. K. Zhang, Z. X. Zou and X. P. Gao, J. Polym. Environ., 2020, 28, 984–994 CrossRef CAS.
- B. Wang, J. R. Moon, S. Ryu, K. D. Park and J. H. Kim, Polym. Compos., 2020, 41, 2578–2587 CrossRef CAS.
- N. Srivastava, M. Srivastava, V. K. Gupta, P. Ramteke and P. Mishra, Bioresour. Technol., 2018, 270, 337–345 CrossRef CAS PubMed.
- R. K. Layek, K. R. Ramakrishnan, E. Sarlin, O. Orell, M. Kanerva, J. Vuorinen and M. Honkanen, J. Mater. Chem. A, 2018, 6, 13203–13214 RSC.
- J. Luo, N. Zhang, R. Liu and X. Liu, RSC Adv., 2014, 4, 64816–64824 RSC.
- K. Liu, H. Li, Y. Wang, X. Gou and Y. Duan, Colloids Surf., A, 2015, 477, 35–41 CrossRef CAS.
- J. Wang, Z. Shi, J. Fan, Y. Ge, J. Yin and G. Hu, J. Mater. Chem., 2012, 22, 22459–22466 RSC.
- Z. Zhao, L. Li, X. M. Shao, X. L. Liu, S. Zhao, S. C. Xie and Z. X. Xin, Polym. Test., 2018, 70, 396–402 CrossRef CAS.
- S. Zhao, S. C. Xie, P. P. Sun, Z. Zhao, L. Li, X. M. Shao, X. L. Liu and Z. X. Xin, RSC Adv., 2018, 8, 17813–17825 RSC.
- J. Luo, N. Zhang, J. P. Lai, R. Liu and X. Y. Liu, J. Hazard. Mater., 2015, 300, 615–623 CrossRef CAS PubMed.
- P. He, J. Y. Wu, X. F. Pan, L. H. Chen, K. Liu, H. L. Gao, H. Wu, S. L. Cao, L. L. Huang and Y. H. Ni, J. Mater. Chem. A, 2020, 8, 3109–3118 RSC.
- K. Tan, R. Liu, J. Luo, Y. Zhu, W. Wei and X. Y. Liu, Prog. Org. Coat., 2019, 130, 214–220 CrossRef CAS.
- A. Bétard and R. A. Fischer, Chem. Rev., 2012, 112, 1055–1083 CrossRef.
- L. Y. Chou, K. Zagorovsky and W. C. Chan, Nat. Nanotechnol., 2014, 9, 148–155 CrossRef CAS PubMed.
- R. Hayward, D. Saville and I. Aksay, Nature, 2000, 404, 56–59 CrossRef CAS PubMed.
- M. P. Cecchini, V. A. Turek, J. Paget, A. A. Kornyshev and J. B. Edel, Nat. Mater., 2013, 12, 165–171 CrossRef CAS.
- S. J. Yang, M. Antonietti and N. Fechler, J. Am. Chem. Soc., 2015, 137, 8269–8273 CrossRef CAS PubMed.
- A. Magasinski, P. Dixon, B. Hertzberg, A. Kvit, J. Ayala and G. Yushin, Nat. Mater., 2010, 9, 353–358 CrossRef CAS PubMed.
- S. Guo and S. Sun, J. Am. Chem. Soc., 2012, 134, 2492–2495 CrossRef CAS.
- T. K. Ross and R. A. Francis, Corros. Sci., 1978, 18, 351–361 CrossRef CAS.
- J. L. Guo, B. L. Tardy, A. J. Christofferson, Y. L. Dai, J. J. Richardson, W. Zhu, M. Hu, Y. Ju, J. W. Cui, R. R. Dagastine, I. Yarovsky and F. Caruso, Nat. Nanotechnol., 2016, 11, 1105–1111 CrossRef CAS.
- J. Lee, O. K. Farha, J. Roberts, K. A. Scheidt, S. T. Nguyen and J. T. Hupp, Chem. Soc. Rev., 2009, 38, 1450–1459 RSC.
- Y. Gossuin, P. Gillis, A. Hocq, Q. L. Vuong and A. Roch, Wiley Interdiscip. Rev.: Nanomed. Nanobiotechnol., 2009, 1, 299–310 CAS.
- Z. Peng and H. Zhong, J. Macromol. Sci., Part B: Phys., 2014, 53, 233–242 CrossRef CAS.
- H. L. Fan, L. Wang, X. D. Feng, Y. Z. Bu, D. C. Wu and Z. X. Jin, Macromolecules, 2017, 50, 666–676 CrossRef CAS.
- N. Ninan, A. Forget, V. P. Shastri, N. H. Voelcker and A. Blencowe, ACS Appl. Mater. Interfaces, 2016, 8, 28511–28521 CrossRef CAS.
- H. Li, X. X. Shu, P. R. Tong, J. H. Zhang, P. F. An, Z. X. Lv, H. Tian, J. T. Zhang and H. B. Xia, Small, 2021, 17, 2102002 CrossRef CAS.
- T. T. Li, X. M. Hu, Q. S. Zhang, Y. Y. Zhao, P. Wang, X. Wang, B. T. Qin and W. Lu, Polym. Adv. Technol., 2020, 31, 1648–1660 CrossRef CAS.
- H. Lee, S. M. Dellatore, W. M. Miller and P. B. Messersmith, Science, 2007, 318, 426–430 CrossRef CAS PubMed.
- X. Ao, Y. Wang, P. Jiang, S. Li and X. Huang, Compos. Sci. Technol., 2018, 154, 154–164 CrossRef.
- M. A. North, C. A. D. Grosso and J. J. Wilker, ACS Appl. Mater. Interfaces, 2017, 9, 7866–7872 CrossRef CAS.
- W. Zhang, R. X. Wang, Z. M. Sun, X. W. Zhu, Q. Zhao, T. F. Zhang, A. Cholewinski, F. K. Yang, B. X. Zhao, R. Pinnaratip, P. K. Forooshani and B. P. Lee, Chem. Soc. Rev., 2020, 49, 433–464 RSC.
- W. Z. Qiu, G. P. Wu and Z. K. Xu, ACS Appl. Mater. Interfaces, 2018, 10, 5902–5908 CrossRef CAS.
- B. Liu, B. Lyle and K. Thomas, J. Am. Chem. Soc., 2006, 128, 15228–15235 CrossRef CAS PubMed.
- J. Yang, M. A. Cohen Stuart and M. Kamperman, ChemInform, 2014, 43, 8271–8298 CAS.
- Y. Cao, N. Liu, W. Zhang, L. Feng and Y. Wei, ACS Appl. Mater. Interfaces, 2016, 8, 3333–3339 CrossRef CAS.
- X. Zhang, Y. Lv, H. C. Yang, Y. Du and Z. K. Xu, ACS Appl. Mater. Interfaces, 2016, 8, 32512–32519 CrossRef CAS.
- Q. Huang, J. Zhao, M. Y. Liu, J. Y. Chen, X. L. Zhu, T. Wu, J. W. Tian, Y. Q. Wen, X. Y. Zhang and Y. Wei, J. Taiwan Inst. Chem. Eng., 2018, 82, 92–101 CrossRef CAS.
- X. Zhang, M. D. Do, P. Casey, A. Sulistio, G. G. Qiao, L. Lundin, P. Lillford and S. Kosaraju, J. Agric. Food Chem., 2010, 58, 6809–6815 CrossRef CAS.
- H. W. Pang, S. J. Zhao, L. T. Mo, Z. Wang, W. Zhang, A. Huang, S. F. Zhang and J. Z. Li, J. Appl. Polym. Sci., 2020, 137, 48785 CrossRef CAS.
- S. J. Ge, N. Ji, S. N. Cui, W. Xie, M. Li, Y. Li, L. Xiong and Q. J. Sun, J. Agric. Food Chem., 2019, 67, 11489–11497 CrossRef CAS PubMed.
- J. S. Guo, W. Sun, J. P. Kim, X. L. Lu, Q. Y. Li, M. Lin, O. Mrowczynski, E. B. Rizk, J. G. Cheng, G. Y. Qian and J. Yang, Acta Biomater., 2018, 72, 35–44 CrossRef CAS PubMed.
- Q. X. Zhao, S. D. Mu, Y. R. Long, J. Zhou, W. Y. Chen, D. Astruc, C. Gaidau and H. B. Gu, Macromol. Mater. Eng., 2019, 304, 1800664 CrossRef.
- Q. Lin, D. Gourdon, C. J. Sun, N. Holten-Andersen, T. H. Anderson, J. H. Waite and J. N. Israelachvili, Proc. Natl. Acad. Sci. U. S. A., 2007, 104, 3782–3786 CrossRef CAS PubMed.
- J. J. Wu, L. Zhang, Y. X. Wang, Y. H. Long, H. Gao, X. L. Zhang, N. Zhao, Y. L. Cai and J. Xu, Langmuir, 2011, 27, 13684–13691 CrossRef CAS PubMed.
- H. Lee, N. F. Scherer and P. B. Messersmith, Proc. Natl. Acad. Sci. U. S. A., 2006, 103, 12999–13003 CrossRef CAS.
- L. Q. Xu, W. J. Yang, K. G. Neoh, E. T. Kang and G. D. Fu, Macromolecules, 2010, 43, 8336–8339 CrossRef CAS.
- H. B. Zeng, D. S. Hwang, J. N. Israelachvili and J. H. Waite, Proc. Natl. Acad. Sci. U. S. A., 2010, 107, 12850–12853 CrossRef CAS PubMed.
- J. Imbrogno, M. D. Williams and G. Belfort, ACS Appl. Mater. Interfaces, 2015, 7, 2385–2392 CrossRef CAS PubMed.
- X. F. Huang, J. W. Jia, Z. K. Wang and Q. L. Hu, Chin. J. Polym. Sci., 2015, 33, 284–290 CrossRef CAS.
- D. Pranantyo, L. Q. Xu, K. G. Neoh and E. T. Kang, Ind. Eng. Chem. Res., 2016, 55, 1890–1901 CrossRef CAS.
- L. Q. Xu, D. Pranantyo, K. G. Neoh, E. T. Kang and G. D. Fu, ACS Sustainable Chem. Eng., 2016, 4, 4264–4272 CrossRef CAS.
- R. Liu, J. J. Zhu, J. Luo and X. Y. Liu, Prog. Org. Coat., 2014, 77, 30–37 CrossRef CAS.
- J. F. Wu, S. Fernando, D. Weerasinghe, Z. G. Chen and D. C. Webster, ChemSusChem, 2011, 4, 1135–1142 CrossRef CAS.
- M. Korey, G. P. Mendis, J. P. Youngblood and J. A. Howarter, J. Polym. Sci., Part A: Polym. Chem., 2018, 56, 1468–1480 CrossRef CAS.
- M. Qi, Y. J. Xu, W. H. Rao, X. Luo, L. Chen and Y. Z. Wang, RSC Adv., 2018, 8, 26948–26958 RSC.
- X. M. Fei, W. Wei, F. Q. Zhao, Y. Zhu, J. Luo, M. Q. Chen and X. Y. Liu, ACS Sustainable Chem. Eng., 2017, 5, 596–603 CrossRef CAS.
- G. M. Roudsari, A. K. Mohanty and M. Misra, ACS Sustainable Chem. Eng., 2014, 2, 2111–2116 CrossRef.
- Y. O. Kim, J. Cho, H. Yeo, B. W. Lee, B. J. Moon, Y. M. Ha, Y. R. Jo and Y. C. Jung, ACS Sustainable Chem. Eng., 2019, 7, 3858–3865 CrossRef CAS.
- X. M. Feng, J. Z. Fan, A. Li and G. Q. Li, ACS Sustainable Chem. Eng., 2020, 8, 874–883 CrossRef CAS.
- J. Handique, J. Gogoi, J. Nath and S. K. Dolui, Polym. Eng. Sci., 2020, 60, 140–150 CrossRef CAS.
- P. Baruah, R. Duarah and N. Karak, Iran. Polym. J., 2016, 25, 849–861 CrossRef CAS.
- W. Z. Qiu, H. C. Yang and Z. K. Xu, Adv. Colloid Interface Sci., 2018, 256, 111–125 CrossRef CAS.
- R. Sa, Y. Yan, Z. H. Wei, L. Q. Zhang, W. C. Wang and M. Tian, ACS Appl. Mater. Interfaces, 2014, 6, 21730–21738 CrossRef CAS.
- Y. He, C. L. Chen, G. Q. Xiao, F. Zhong, Y. Q. Wu and Z. He, React. Funct. Polym., 2019, 137, 104–115 CrossRef CAS.
- C. Chen, X. Yang, S. J. Li, F. J. Ma, X. Yan, Y. N. Ma, Y. X. Ma, Q. H. Ma, S. Z. Gao and X. J. Huang, RSC Adv., 2021, 11, 5182–5191 RSC.
- M. C. Roberts, M. C. Hanson, A. P. Massey, E. A. Karren and P. F. Kiser, Adv. Mater., 2007, 19, 2503–2507 CrossRef CAS.
- L. He, D. E. Fullenkamp, J. G. Rivera and P. B. Messersmith, Chem. Commun., 2011, 47, 7497–7499 RSC.
- J. A. Burdick and W. L. Murphy, Nat. Commun., 2012, 3, 1269 CrossRef PubMed.
- S. H. Hong, S. Kim, J. P. Park, M. Shin, K. Kim, J. H. Ryu and H. Lee, Biomacromolecules, 2018, 19, 2053–2061 CrossRef CAS.
- J. N. Cambre and B. S. Sumerlin, Polymer, 2011, 52, 4631–4643 CrossRef CAS.
- E. Faure, C. Falentin-Daudré, C. Jérôme, J. Lyskawa, D. Fournier, P. Woisel and C. Detrembleur, Prog. Polym. Sci., 2013, 38, 236–270 CrossRef CAS.
- B. M. W. Wohl and J. F. J. Engbersen, J. Controlled Release, 2012, 158, 2–14 CrossRef CAS PubMed.
- U. Manna and S. Patil, ACS Appl. Mater. Interfaces, 2010, 2, 1521–1527 CrossRef CAS PubMed.
- J. L. Guo, H. L. Sun, K. Alt, B. L. Tardy, J. J. Richardson, T. Suma, H. Ejima, J. W. Cui, C. E. Hagemeyer and F. Caruso, Adv. Healthcare Mater., 2015, 4, 1796–1801 CrossRef CAS.
- Z. J. Huang, P. Delparastan, P. Burch, J. Cheng, Y. Cao and P. B. Messersmith, Biomater. Sci., 2018, 6, 2487–2495 RSC.
- E. Montanari, A. Gennari, M. Pelliccia, C. Gourmel, E. Lallana, P. Matricardi, A. J. McBain and N. Tirelli, Macromol. Biosci., 2016, 16, 1815–1823 CrossRef CAS PubMed.
- O. Mitsunobu, M. Yamada and T. Mukaiyama, Bull. Chem. Soc. Jpn., 1967, 40, 935–939 CrossRef CAS.
- C. Chen, X. W. Geng, Y. H. Pan, Y. N. Ma, Y. X. Ma, S. Z. Gao and X. J. Huang, RSC Adv., 2020, 10, 1724–1732 RSC.
- J. Wei, G. Wang, F. Chen, M. Bai, Y. Liang, H. T. Wang, D. Y. Zhao and Y. X. Zhao, Angew. Chem., Int. Ed., 2018, 57, 9838–9843 CrossRef CAS.
- M. M. Zhang, S. X. Zhang, X. X. Liu, H. Chen, Y. F. Ming, Q. Xu and Z. H. Wang, Environ. Sci. Pollut. Res., 2019, 26, 31834–31845 CrossRef CAS PubMed.
- G. Y. Chen, Y. R. Cao, L. Ke, X. X. Ye, X. Huang and B. Shi, Ind. Eng. Chem. Res., 2018, 57, 16442–16450 CrossRef CAS.
- D. L. Tao, L. Z. Feng, Y. Chao, C. Liang, X. J. Song, H. C. Wang, K. Yang and Z. Liu, Adv. Funct. Mater., 2018, 28, 1804901 CrossRef.
- N. Sahiner and S. B. Sengel, Colloids Surf., A, 2016, 508, 30–38 CrossRef CAS.
- J. S. Guo, X. G. Tian, D. H. Xie, K. Rahn, E. Gerhard, M. L. Kuzma, D. F. Zhou, C. Dong, X. C. Bai, Z. H. Lu and J. Yang, Adv. Funct. Mater., 2020, 30, 2002438 CrossRef CAS.
- A. Fujimoto and T. J. Masuda, J. Agric. Food Chem., 2012, 60, 5142–5151 CrossRef CAS PubMed.
- L. Zhang, L. B. Cheng, L. J. Jiang, Y. S. Wang, G. X. Yang and G. Y. He, J. Sci. Food Agric., 2010, 90, 2462–2468 CrossRef CAS.
- C. Chen, X. Yang, S. J. Li, C. Zhang, Y. N. Ma, Y. X. Ma, P. Gao, S. Z. Gao and X. J. Huang, Green Chem., 2021, 23, 1794–1804 RSC.
- J. A. Stella, A. D'Amore, W. R. Wagner and M. S. Sacks, Acta Biomater., 2010, 6, 2365–2381 CrossRef PubMed.
- A. R. Amini, C. T. Laurencin and S. P. Nukavarapu, CRC Crit. Rev. Bioeng., 2012, 40, 363–408 Search PubMed.
- A. M. Pobloth, S. Checa, H. Razi, A. Petersen, J. C. Weaver, K. Schmidt-Bleek, M. Windolf, A. Á. Tatai, C. P. Roth, K. D. Schaser, G. N. Duda and P. Schwabe, Sci. Transl. Med., 2018, 10, eaam8828 CrossRef.
- J. Gleeson, N. Plunkett and F. O'Brien, Eur. Cells Mater., 2010, 20, 218–230 CrossRef CAS PubMed.
- D. Gan, W. Xing, L. Jiang, J. Fang, C. Zhao, F. Ren, L. Fang, K. Wang and X. Lu, Nat. Commun., 2019, 10, 1487 CrossRef.
- S. M. Bai, X. L. Zhang, P. Q. Cai, X. W. Huang, Y. Q. Huang, R. Liu, M. Y. Zhang, J. B. Song, X. D. Chen and H. B. Yang, Nanoscale Horiz., 2019, 4, 1333–1341 RSC.
- J. U. Lee and G. H. Kim, ACS Biomater. Sci. Eng., 2018, 4, 278–289 CrossRef CAS.
- S. M. Bai, X. L. Zhang, X. L. Lv, M. Y. Zhang, X. W. Huang, Y. Shi, C. H. Lu, J. B. Song and H. B. Yang, Adv. Funct. Mater., 2019, 30, 1908381 CrossRef.
- S. R. Abulateefeh and M. O. Taha, J. Microencapsulation, 2015, 32, 96–105 CrossRef CAS PubMed.
- W. Zhang, C. Ling, H. Y. Liu, A. N. Zhang, L. Mao, J. Wang, J. Chao, L. J. Backman, Q. Q. Yao and J. L. Chen, Chem. Eng. J., 2020, 396, 125232 CrossRef CAS.
- L. Q. Xu, K. G. Neoh and E. T. Kang, Prog. Polym. Sci., 2018, 87, 165–196 CrossRef CAS.
- W. W. Shen, Q. W. Wang, Y. Shen, X. Gao, L. Li, Y. Yan, H. Wang and Y. Y. Cheng, ACS Cent. Sci., 2018, 4, 1326–1333 CrossRef CAS PubMed.
- H. L. Fan, J. H. Wang and Z. X. Jin, Macromolecules, 2018, 51, 1696–1705 CrossRef CAS.
- C. Y. Shao, M. Wang, L. Meng, H. L. Chang, B. Wang, F. Xu, J. Yang and P. B. Wan, Chem. Mater., 2018, 30, 3110–3121 CrossRef CAS.
- X. M. Fan, S. B. Wang, Y. Fang, P. Y. Li, W. K. Zhou, Z. C. Wang, M. F. Chen and H. Q. Liu, Mater. Sci. Eng., C, 2020, 109, 110649 CrossRef CAS PubMed.
- R. Wang, X. X. Wang, Y. J. Zhan, Z. Xu, Z. Q. Xu, X. H. Feng, S. Li and H. Xu, ACS Appl. Mater. Interfaces, 2019, 11, 37502–37512 CrossRef CAS PubMed.
- E. C. Davidson, Surg., Gynecol. Obstet., 1925, 41, 202–221 Search PubMed.
- M. Farokhi, F. Mottaghitalab, Y. Fatahi, A. Khademhosseini and D. L. Kaplan, Trends Biotechnol., 2018, 36, 907–922 CrossRef CAS PubMed.
- N. Kojic, M. J. Panzer, G. G. Leisk, W. K. Raja, M. Kojic and D. L. Kaplan, Soft Matter, 2012, 8, 6897–6905 RSC.
- M. Parani, G. Lokhande, A. Singh and A. K. Gaharwar, ACS Appl. Mater. Interfaces, 2016, 8, 10049–10069 CrossRef CAS PubMed.
- Y. Y. Zheng, Y. Q. Liang, D. P. Zhang, X. Y. Sun, L. Liang, J. Li and Y. N. Liu, ACS Omega, 2018, 3, 4766–4775 CrossRef CAS.
- F. F. Sun, Y. Z. Bu, Y. R. Chen, F. Yang, J. K. Yu and D. C. Wu, ACS Appl. Mater. Interfaces, 2020, 12, 9132–9140 CrossRef CAS PubMed.
- M. S. Ma, Y. L. Zhong and X. L. Jiang, Carbohydr. Polym., 2020, 236, 116096 CrossRef CAS PubMed.
- J. Jing, S. F. Liang, Y. F. Yan, X. Tian and X. M. Li, ACS Biomater. Sci. Eng., 2019, 5, 4601–4611 CrossRef CAS PubMed.
- Y. L. Yu, P. F. Li, C. L. Zhu, N. Ning, S.
Y. Zhang and G. J. Vancso, Adv. Funct. Mater., 2019, 29, 1904402 CrossRef.
- M. R. Humphreys, E. P. Castle, P. E. Andrews, M. T. Gettman and M. H. Ereth, Am. J. Surg., 2008, 195, 99–103 CrossRef CAS PubMed.
- N. Li, X. Yang, W. Liu, G. H. Xi, M. S. Wang, B. Liang, Z. P. Ma, Y. K. Feng, H. Chen and C. C. Shi, Macromol. Biosci., 2018, 18, 1800209 CrossRef PubMed.
- C. Chen, W. Sun, X. Wang, Y. Wang and P. Wang, Int. J. Biol. Macromol., 2018, 111, 1106–1115 CrossRef CAS PubMed.
- S. D. Li and L. Huang, Mol. Pharmaceutics, 2008, 5, 496–504 CrossRef CAS PubMed.
- H. Yang, Q. Wang, Z. F. Li, F. Y. Li, D. Wu, M. Fan, A. B. Zheng, B. Huang, L. Gan, Y. L. Zhao and X. L. Yang, Nano Lett., 2018, 18, 7909–7918 CrossRef CAS PubMed.
- H. Yang, Q. Wang, W. Chen, Y. B. Zhao, T. Y. Yong, L. Gan, H. B. Xu and X. L. Yang, Mol. Pharmaceutics, 2015, 12, 1636–1647 CrossRef CAS PubMed.
- Q. H. Sun, Z. X. Zhou, N. S. Qiu and Y. Q. Shen, Adv. Mater., 2017, 29, 1606628 CrossRef.
- C. Y. Sun, Y. Liu, J. Z. Du, Z. T. Cao, C. F. Xu and J. Wang, Angew. Chem., Int. Ed., 2016, 55, 1010–1014 CrossRef CAS PubMed.
- Q. Zhou, S. Q. Shao, J. Q. Wang, C. H. Xu, J. J. Xiang, Y. Piao, Z. X. Zhou, Q. S. Yu, J. B. Tang, X. R. Liu, Z. H. Gan, R. Mo, Z. Gu and Y. Q. Shen, Nat. Nanotechnol., 2019, 14, 799–809 CrossRef CAS.
- H. Xiong, Z. H. Wang, C. Wang and J. Yao, Nano Lett., 2020, 20, 1781–1790 CrossRef CAS PubMed.
- C. Chen, T. H. Ma, W. Tang, X. L. Wang, Y. B. Wang, J. F. Zhuang, Y. C. Zhu and P. Wang, Nanoscale Horiz., 2020, 5, 986–998 RSC.
- H. M. Zhu, G. D. Cao, Y. K. Fu, C. Fang, Q. Chu, X. Li, Y. L. Wu and G. R. Han, Nano Res., 2021, 14, 222–231 CrossRef CAS.
- J. Liu, Y. Deng, D. Fu, Y. Yuan, Q. L. Li, L. Shi, G. B. Wang, Z. Wang and L. Wang, Bioact. Mater., 2021, 6, 273–284 CrossRef CAS PubMed.
- Y. Wang, Z. Wang, C. Xu, H. Tian and X. Chen, Biomaterials, 2019, 197, 284–293 CrossRef CAS PubMed.
- T. Liu, M. K. Zhang, W. L. Liu, X. Zeng, X. L. Song, X. Q. Yang, X. Z. Zhang and J. Feng, ACS Nano, 2018, 12, 3917–3927 CrossRef CAS PubMed.
- A. Vargas Jentzsch, A. Hennig, J. Mareda and S. Matile, Acc. Chem. Res., 2013, 46, 2791–2800 CrossRef CAS PubMed.
- J. Li, X. Li, S. Gong, C. Zhang, C. Qian, H. Qiao and M. Sun, Nano Lett., 2020, 20, 4842–4849 CrossRef CAS.
- Y. B. Wang, F. Liu, N. Yan, S. Sheng, C. N. Xu, H. Y. Tian and X. S. Chen, ACS Biomater. Sci. Eng., 2019, 5, 4700–4707 CrossRef CAS.
- F. He, M. C. Han, J. Zhang, Z. X. Wang, X. C. Wu, Y. Y. Zhou, L. F. Jiang, S. Q. Peng and Y. X. Li, Nano Energy, 2020, 71, 104650 CrossRef CAS.
- Z. X. Wang, X. C. Wu, J. M. Dong, X. H. Yang, F. He, S. Q. Peng and Y. X. Li, Chem. Eng. J., 2022, 427, 130905 CrossRef CAS.
- Q. Shi, K. Wu, X. Y. Huang, R. Xu, W. Zhang, J. Bai, S. Y. Du and N. Han, Colloids Surf., A, 2021, 618, 126475 CrossRef CAS.
- T. Chen, R. T. Huang, J. W. Liang, B. Zhou, X. L. Guo, X. C. Shen and B. P. Jiang, Chem.–Eur. J., 2020, 26, 15159–15169 CrossRef CAS.
- X. Huang, J. Z. Liu, P. Zhou, G. H. Su, T. Zhou, X. X. Zhang and C. H. Zhan, Small, 2021, 2104048, DOI:10.1002/smll.202104048.
- Y. Y. Wang, X. Huang and X. X. Zhang, Nat. Commun., 2021, 12, 1291 CrossRef CAS PubMed.
|
This journal is © The Royal Society of Chemistry 2022 |
Click here to see how this site uses Cookies. View our privacy policy here.