DOI:
10.1039/D1QM01184G
(Research Article)
Mater. Chem. Front., 2022,
6, 33-39
Intramolecular-locked triphenylamine derivatives with adjustable room temperature phosphorescence properties by the substituent effect†
Received
23rd August 2021
, Accepted 31st October 2021
First published on 6th November 2021
Abstract
Organic room temperature phosphorescent materials have attracted a lot of research interest due to their wide applications. Herein, a series of intramolecular-locked triphenylamine derivatives have been synthesized with adjustable room temperature phosphorescence (RTP) properties, in which the RTP lifetime ranged from 30 to 288 ms and the emission color varied from sky blue to green. These results are mainly related to the substituent effect, which can tune the intramolecular charge transfer and intermolecular interactions, contributing to different intersystem crossing (ISC) processes, and the stability and energy levels of excited triplet states.
Room-temperature phosphorescence (RTP) of pure organic materials has attracted considerable attention for their potential applications in biological imaging,1–4 organic light-emitting diodes (OLEDs),5,6 sensors,7–9 optics and anti-counterfeiting technologies.10–20 However, it is still a challenge for organic luminogens to achieve persistent RTP with a long lifetime and a high efficiency, which is mainly related to two critical points: (a) the spin-forbidden intersystem crossing (ISC) process, which is hard to be overcome, and (b) the unstable excited triplet state, which can be easily relaxed by molecular motions as nonradiative transitions and quenched by oxygen and moisture.21–30
Recently, some efficient strategies have been proposed to achieve persistent RTP in pure organic materials.31–36 The introduction of heteroatoms with lone-pair electrons can promote the ISC process with increased spin–orbit coupling (SOC).37,38 Also, molecular rigidity is the key factor to the stability of excited triplet states, since the rigid conjugated system can suppress the possible nonradiative transitions by molecular motions.39,40 Alternatively, the substituents as the periphery groups play an assistant role in optimizing the molecular packing and intermolecular interactions.41–45 As one of the impressive examples, through the incorporation of substituents with different electronic properties into the 10-phenyl-10H-phenothiazine 5,5-dioxide core, the enhanced π–π electronic coupling of conjugated cores is obtained by the increased electron-withdrawing ability of substituents, contributing to the ultralong RTP effect.45
In this work, the commonly used triphenylamine (TPA) unit is selected as the conjugated skeleton,46 which exhibits nearly no RTP in the natural state. With the aim to enhance the molecular rigidity, an intramolecular locking strategy is employed to bind the two phenyl units together by carbon chains. The resultant compound C-TPA exhibits the observed afterglow with a RTP lifetime of 30 ms (Fig. 1), confirming the crucial role of molecular rigidity. Furthermore, different substituents are introduced to the unbinding phenyl in the TPA moiety to promote the intermolecular interactions. The RTP lifetimes of C-TPA-OMe and C-TPA-Me with methoxyl and methyl modification increase to 184 ms and 288 ms, respectively, while the emission color changes from sky blue to green. It is mainly related to the additional C–H⋯π interactions induced by alkyl chains and to the optimized molecular packing by the substituent effect. Herein, we would like to present the synthesis, characterization, and crystal structures to shed some light on the relationship among the molecular structure, molecular packing and RTP effect.
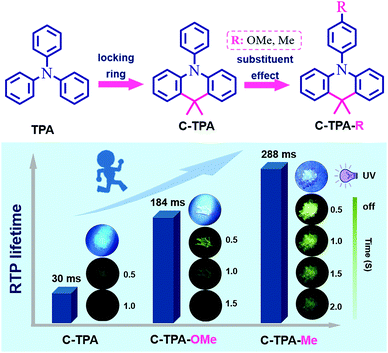 |
| Fig. 1 Molecular design strategy, molecular structures, and RTP lifetimes of C-TPA, C-TPA-OMe, and C-TPA-Me (crystals), and photographs taken before and after irradiation (365 nm) under ambient conditions. | |
Synthesis and emissive properties
The target compounds C-TPA, C-TPA-OMe and C-TPA-Me were facilely prepared through C–N coupling reactions (Scheme S1, ESI†). Their molecular structures were fully characterized by 1H NMR and 13C NMR spectroscopy, elemental analysis, HPLC and single-crystal X-ray diffraction (Fig. S1–S3 and S26–S31, ESI†). Their UV-visible absorption spectra in tetrahydrofuran (THF) solution (concentration: 10 μM) exhibit similar absorption peaks at about 270 nm (Fig. S4 and S5, ESI†), which become broader in the solid state. Compared to the photoluminescence (PL) spectrum of C-TPA without substituents in THF solution (Table 1), those of C-TPA-OMe and C-TPA-Me show blue-shifted emissions (Fig. S4, ESI†), mainly due to the electron-donating ability of methoxyl and methyl moieties. However, for their single crystals, after turning off the ultraviolet (UV) lamp (365 nm) (Fig. 1), sky-blue afterglow of C-TPA and green one of C-TPA-OMe could be visualized with a lifetime of up to 2.0 s. The persistent afterglow can be further confirmed by the time-dependent phosphorescence spectra (Fig. S6–S8, ESI†).
Table 1 Photophysical properties of the three C-TPA derivatives
Sample |
λ
abs(solution) (nm) |
λ
F(solution) (nm) |
λ
F(crystal) (nm) |
λ
P(crystal) (nm) |
τ
P(crystal) (ms) |
Φ
F(crystal) (%) |
Φ
p(crystal) (%) |
C-TPA |
275 |
369 |
351 |
475 |
30 |
13.47 |
2.26 |
C-TPA-OMe |
270 |
362 |
386 |
508 |
184 |
83.55 |
— |
C-TPA-Me |
270 |
363 |
361 |
529 |
288 |
20.83 |
2.0 |
Comparing the maximum PL wavelengths of these crystals carefully, there is a red-shifting tendency from C-TPA (351 nm) to C-TPA-Me (361 nm), then to C-TPA-OMe (386 nm). Similarly, the RTP emission peaks change from 475 nm for C-TPA with a lifetime of 30 ms (Fig. S9, ESI†), to 508 nm for C-TPA-OMe with a lifetime of 184 ms (Fig. 2a), then to 529 nm for C-TPA-Me with the increased RTP intensity and lifetime (288 ms), which is over 10 times of that of C-TPA (Fig. 2). When the temperature decreased to 77 K, the phosphorescence intensity increased largely, mainly due to the suppression of molecular motions as nonradiative transitions (Fig. S10–S14, ESI†). Once these crystals have been ground into powder, the XRD spectra exhibited the crystalline state to some extent (Fig. S15, ESI†), mainly related to the good crystallinity of these compounds. Accordingly, the RTP lifetimes of C-TPA, C-TPA-OMe and C-TPA-Me powders changed to 22 ms, 104 ms and 275 ms, respectively (Fig. S16 and S17, ESI†).
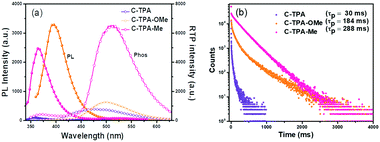 |
| Fig. 2 (a) The steady-state PL and phosphorescence and (b) temperature-dependent phosphorescence decays for C-TPA, C-TPA-OMe and C-TPA-Me crystals. | |
The persistent RTP effect of these C-TPA derivatives in the crystalline state or single crystal form can be explained by the theoretical calculations and crystal analysis.
Theoretical calculations
With the aim to explore the possible mechanisms of the emissive properties of three C-TPA derivatives, the optimized geometry, electrostatic potential (ESP) (Fig. S18, ESI†), frontier molecular orbitals and energy levels of TPA, C-TPA, C-TPA-OMe and C-TPA-Me were investigated by time-dependent density functional theory (TD-DFT) (Tables S2–S14, ESI†). H and L represent the highest occupied molecular orbital (HOMO) and the lowest unoccupied molecular orbital (LUMO), respectively. The blue arrows refer to the possible ISC channels. The major ISC channels were mainly determined by two elements: Tm gave a small energy gap (<0.3 eV) with S1 and contained the same transition configurations as the S1 state.47,48 When the energy of Tm was similar to or lower than that of S1, the latter element was considered to be more important. For TPA, as indicated in Fig. 3a, ΔE(S1–T1) is relatively large (0.75 eV), and the natural transition orbital (NTO) shows that S1 and T1 are locally excited (LE) states, and there are no channels for the ISC process (Tables S3 and S14, ESI†). Thus, TPA exhibits nearly no RTP properties. Once the two phenyl moieties are locked together by alkyl chains, the resultant C-TPA derivatives show twisted conformations with a torsion angle of about 90° between the free phenyl and the locked moiety. Furthermore, the DFT calculations of C-TPA derivatives exhibited a poor overlap between the HOMO and LUMO of intramolecular charge transfer (ICT) states, resulting in a reduced ΔE(S1–T1) and thus enhanced ISC processes.49–51 Accordingly, there are many ISC channels from S1 to Tm for these C-TPA derivatives (Tables S4–S13, ESI†), contributing to the RTP effect.52–54 Therefore, the NTOs of the excited states are shown in Table S14 (ESI†), and the S1 state of locked structures exhibits charge transfer (CT) character, while high-energy triplets with small ΔE(S1–Tm) exhibit more CT properties, and the T1 state shows locally excited (LE) states, which also exhibit 3(π,π*) transitions. Thus, intramolecular charge-transfer states (1CT and 3CT) are generated, and a stepwise energy transfer process of 1CT → 3CT → 3π–π* could be achieved to serve as intermediate states for minimizing ΔEST. In theory, this could accelerate kISC and promote the strong emission of phosphorescence.30,55–57
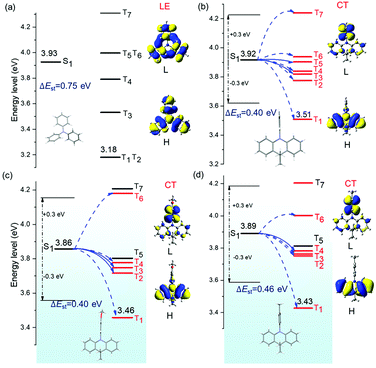 |
| Fig. 3 Diagrams of the TD-DFT calculated energy levels and possible ISC channels of single molecular TPA (a), C-TPA (b), C-TPA-OMe (c) and C-TPA-Me (d) at the excited singlet (S1) and triplet (Tm) states. H and L represent the highest occupied molecular orbital (HOMO) and the lowest unoccupied molecular orbital (LUMO), respectively. LE and CT mean locally excited and charge transfer states, respectively. The insets show the Kohn–Sham frontier orbitals obtained from the isolated states of these compounds. | |
Compared to the single molecule, substantial electronic interactions among the dimers of C-TPA derivatives result in the overlap of the excitonic orbitals, giving rise to energy splitting.30 Furthermore, the energy gaps between S1 and T1 states decrease (Fig. S19–S21 and Table S2, ESI†), which could lead to a reduced energy loss in the process of internal conversion at excited triplet states.58 The increased ISC channels and decreased ΔE(S1–T1) in the molecular dimer will jointly promote the generation of phosphorescence. The above calculation results theoretically explain the effective promotion of room temperature phosphorescence by molecular dimers and further prove the tremendous influence of molecular packing on their luminescence properties.
Crystal analysis
Fig. 4 presents the molecular conformations, dimers and packing of these three molecules in single crystals. All the C-TPA derivatives show twisted conformations, and the dihedral angles between the phenyl and the acridine moiety (as the locked structure) are in the range of 84–86°. The dihedral angle of the two phenyl groups in the acridine ring is about 30°, forming a butterfly-like spatial structure. Efficient intermolecular interactions, such as C–H⋯π and C–H⋯N, are observed, while no π–π interactions were found (Fig. S22–S24, ESI†). The intermolecular interactions would restrict the intermolecular motions effectively and lead to reduced non-radiative transitions, which could contribute greatly to the relatively high PL efficiencies of these materials.58–60 To simplify the packing analyses (Fig. S23, ESI†), for C-TPA, the molecules are intertwined, making the order of the crystal arrangement relatively poor. Furthermore, the herringbone arrangement of C-TPA-OMe is composed of mostly anti-parallel dimers. Then, the layered and orderly herringbone stacking was found in methyl-substituted C-TPA-Me, and thus the introduction of substituents can optimize molecular stacking.
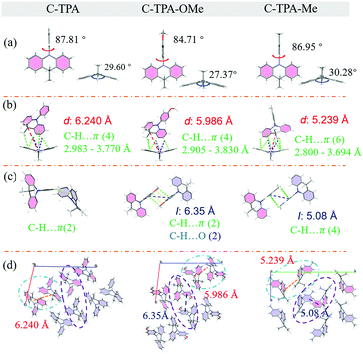 |
| Fig. 4 Single-crystal structures of the three target compounds: (a) The monomer, (b) dimer A of intra-unit-cell and (c) dimer B of inter-unit-cell; (d) packing of the molecules of C-TPA, C-TPA-OMe and C-TPA-Me. d represents the distances of carbon chains to the locked moiety. I represents the centroid–centroid distance of unbinding phenyl. | |
To analyse crystals in detail, we choose dimer A (Fig. 4b), which accounts for the majority in one unit cell, to represent the interaction within the unit cell. The distances d of C (lock) in one molecule to a locked moiety of another molecule in dimer A are regarded as a typical element to monitor the distance of adjacent molecules. As for the inter-unit-cell dimer, the anti-parallel dimer B (Fig. 4c) as a representative to study the interaction between the unit cells is chosen, except C-TPA, because the two unbinding phenyls of the two molecules are interlaced at an angle of 52.11°. For the substituents of C-TPA derivatives varying from H to OCH3 to CH3, the resultant compounds exhibit a decrease in distance d from 6.240 Å to 5.986 Å to 5.239 Å, which is supposed to be associated with luminous properties. For the intra-unit-cell dimer A of C-TPA, there are four types of C–H⋯π interaction (2.983–3.770 Å) and two types of C–H⋯N interaction (3.450 and 3.632 Å) (Table S16, ESI†). Furthermore, the inter-unit-cell dimer B contained two types of C–H⋯π interaction (3.328 Å and 3.743 Å). Moreover, in comparison with the H substituted compound, the additional group of the OCH3 substituted one provides more kinds of C–H⋯O and C–H⋯π interactions. For the intra-unit-cell dimer A of C-TPA-OMe, four types of C–H⋯π interaction (2.905–3.830 Å) and two types of C–H⋯N interaction (3.598 and 3.909 Å) are found. Additionally, there are two types of C–H⋯O interaction (3.875 Å) and two types of C–H⋯π interaction (3.554 Å) in the anti-parallel dimer B of inter-unit-cell (centroid–centroid distance of the unbinding phenyl is 6.35 Å). It can be found from the data that the intermolecular interaction of C-TPA-OMe is obviously stronger than that of C-TPA, and with more compact packing, which also contributed to the persistent RTP effect of C-TPA-OMe. As for C-TPA-Me, multiple hydrogen bonds of C–H⋯π (distances ranging from 2.800 to 3.694 Å) and C–H⋯N (distances of 3.556 and 3.898 Å) are found in dimer A. In the anti-parallel dimer B of inter-unit-cell (centroid–centroid distance of the unbinding phenyl is decreased to 5.08 Å), there are four kinds of C–H⋯π, whose distances range from 3.749 Å to 3.915 Å. It can be derived from the comparation that compact and orderly molecular packing leads to a low nonradiative transition of the conjugated system, resulting in the persistent RTP effect with a long RTP lifetime. These intense intermolecular interactions could largely reduce the possible energy loss through non-radiative relaxation, which is harmful for its phosphorescence. In summary, tight stacking with multiple C–H⋯π intermolecular interactions (listed in Table S16, ESI†) can also be further proved as a crucial factor to RTP lifetime.
The above experimental results and theoretical calculations illustrate that molecular stacking plays an important role in the luminescence process of the C-TPA compound derivatives. As shown in Fig. 5, from TPA to C-TPA-R, for the achievement of persistent RTP, two factors should be noted: two phenyl moieties locked by alkyl chains produce a CT orbital, generating a stepwise energy transfer process of 1CT → 3CT → 3π–π* for enhancing the intersystem crossing, which promoted phosphorescence.55–57 Furthermore, the results proved that the intramolecular locking strategy was highly necessary. In addition, the introduction of substituents not only promoted intramolecular interactions but also optimized molecular packing, which can facilitate the ISC process and suppress the molecular motions to achieve persistent RTP.41,42,58–62
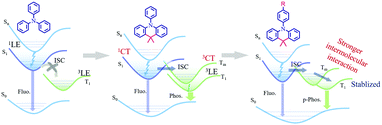 |
| Fig. 5 The proposed mechanism for PL processes in isolated molecules of TPA and C-TPA derivatives’ transition from the S0 to the S1 state. The ISC transition is not favoured in TPA. Formation of a CT transition bridge within molecular C-TPA could facilitate the communication between the excited singlet and triplet states to produce the RTP effect. C-TPA derivatives with strong intermolecular interactions can stabilize triplet states to extend the phosphorescence lifetime. | |
Lastly, considering their application, due to their different photophysical properties the three compounds may have potential use as anti-counterfeiting materials. As shown in Fig. S25 (ESI†), a simple encryption pattern was fabricated and combined with the prominent RTP feature under UV excitation. Under 365 nm UV light irradiation, the shape of two birds emit deep blue. After ceasing the UV irradiation, the visible green RTP of bird (up) can be readily visualized and lasts for seconds because of the long RTP lifetime; at the same time, the bird (bottom) emits sky-blue light but disappears quickly. Thus, the feasible application of the three compounds in information encryption and decryption is successfully demonstrated.
Conclusions
In summary, a series of intramolecular-locked triphenylamine derivatives have been synthesized with the incorporation of different substituents to the unlocked phenyl moiety. Compared to C-TPA without a substituent, C-TPA-OMe and C-TPA-Me with methoxyl and methyl modification exhibited largely increased RTP lifetimes of 184 ms and 288 ms, respectively, and the emission color varied from sky blue to green. This is mainly related to the additional C–H⋯π interactions induced by alkyl chains and to the optimized molecular packing by the substituent effect, which can extend the RTP lifetime from 30 ms to 288 ms. Thus, the idea of how molecular structure design regulates molecular packing and interactions can give a better understanding of the molecular structure–packing–performance relationship. This phenomenon could be termed as a Molecular Uniting Set Identified Characteristic (MUSIC).21 It is envisaged that the results of the present study could promote further studies based on rational structure design for the development of efficient organic p-RTP materials.
Author contributions
Li Zhen and Li Qianqian conceived the project. Han Mengmeng synthesized all the materials and performed all photophysical measurements. Xu Zhichen and Lu Jie performed a part of the synthetic experiments. Xie Yujun discussed the data. Han Mengmeng performed the theoretical calculations and wrote the paper. Li Qianqian and Li Zhen discussed and revised the manuscript. All the authors approved the manuscript.
Conflicts of interest
There are no conflicts to declare.
Acknowledgements
We are grateful to the National Natural Science Foundation of China (no. 51973162 and 21734007), Excellent Youth Foundation of Hubei Scientific Committee (2020CFA084), and the Fundamental Research Funds for the Central Universities (2042020kf0200) and Wuhan City (2019010701011412) for financial support. We also thank the national supercomputing center in Shenzhen.
Notes and references
- M. Palner, K. Pu, S. Shao and J. Rao, Semiconducting polymer nanoparticles with persistent near-infrared luminescence for in vivo optical imaging, Angew. Chem., Int. Ed., 2015, 54, 11477–11480 CrossRef CAS PubMed.
- S. M. A. Fateminia, Z. Mao, S. Xu, Z. Yang, Z. Chi and B. Liu, Organic nanocrystals with bright red persistent room-temperature phosphorescence for biological applications, Angew. Chem., Int. Ed., 2017, 56, 12160–12164 CrossRef CAS PubMed.
- S. Liu, H. Zhang, Y. Li, J. Liu, L. Du, M. Chen, R. T. K. Kwok, J. W. Y. Lam, D. L. Phillips and B. Z. Tang, Strategies to enhance the photosensitization: polymerization and the donor-acceptor even-odd effect, Angew. Chem., Int. Ed., 2018, 57, 15189–15193 CrossRef CAS.
- S. Xu, R. Chen, C. Zheng and W. Huang, Excited state modulation for organic afterglow: materials and applications, Adv. Mater., 2016, 28, 9920–9940 CrossRef CAS PubMed.
- R. Kabe, N. Notsuka, K. Yoshida and C. Adachi, Afterglow organic light-emitting diode, Adv. Mater., 2016, 28, 655–660 CrossRef CAS.
- Z. Yang, Z. Mao, Z. Xie, Y. Zhang, S. Liu, J. Zhao, J. Xu, Z. Chi and M. P. Aldred, Recent advances in organic thermally activated delayed fluorescence materials, Chem. Soc. Rev., 2017, 46, 116–915 Search PubMed.
- J. Xu, H. Feng, H. Teng, G. Chen, S. Pan, J. Chen and Z. Qian, Reversible switching between phosphorescence and fluorescence in a unimolecular system controlled by external stimuli, Chem. – Eur. J., 2018, 24, 12773–12778 CrossRef CAS.
- W. Sun, Z. Wang, T. Wang, L. Yang, J. Jiang, X. Zhang, Y. Luo and G. Zhang, Protonation-induced room-temperature phosphorescence in fluorescent polyurethane, J. Phys. Chem. A, 2017, 121, 4225–4232 CrossRef CAS.
- Z. Chai, C. Wang, J. Wang, F. Liu, Y. Xie, Y. Zhang, J. Li, Q. Li and Z. Li, Abnormal room temperature phosphorescence of purely organic boron-containing compounds: the relationship between the emissive behavior and the molecular packing, and the potential related applications, Chem. Sci., 2017, 8, 8336–8344 RSC.
- Z. He, H. Gao, S. Zhang, S. Zheng, Y. Wang, Z. Zhao, D. Ding, B. Yang, Y. Zhang and W. Z. Yuan, Achieving persistent, efficient, and robust room-temperature phosphorescence from pure organics for versatile applications, Adv. Mater., 2019, 31, 1807222 CrossRef.
- R. Iqbal, A. Ahmad, L. Mao, Z. A. Ghazi, A. Imani, C. Lu, L. Xie, S. Melhi, F. Su, C. Chen, L. Zhi and Z. Wei, A high energy density self-supported and bendable organic electrode for redox supercapacitors with a wide voltage window, Chin. J. Polym. Sci., 2020, 38, 522–530 CrossRef CAS.
- L. Bian, H. Ma, W. Ye, A. Lv, H. Wang, W. Jia, L. Gu, H. Shi, Z. An and W. Huang, Color-tunable ultralong organic phosphorescence materials for visual UV-light detection, Sci. China: Chem., 2020, 63, 1443–1448 CrossRef CAS.
- Y. Yang, T. Shan, J. Cao, H. Wang, J. Wang, H. Zhong and Y. Xu, Unsymmetric side chains of indacenodithiophene copolymers lead to improved packing and device performance, Chin. J. Polym. Sci., 2020, 38, 342–348 CrossRef CAS.
- J. Wang, W. Zhu, C. Li, P. Zhang, G. Jiang, G. Niu and B. Z. Tang, Mitochondria-targeting NIR fluorescent probe for rapid, highly sensitive and selective visualization of nitroxyl in live cells, tissues and mice, Sci. China: Chem., 2020, 63, 282–289 CrossRef CAS.
- L. Gu, X. Wang, M. Singh, H. Shi, H. Ma, Z. An and W. Huang, Organic room-temperature phosphorescent materials: from static to dynamic, J. Phys. Chem. Lett., 2020, 11, 6191–6200 CrossRef CAS.
- L. Bian, H. Shi, X. Wang, K. Ling, H. Ma, M. Li, Z. Cheng, C. Ma, S. Cai, Q. Wu, N. Gan, X. Xu, Z. An and W. Huang, Simultaneously enhancing efficiency and lifetime of ultralong organic phosphorescence materials by molecular self-assembly, J. Am. Chem. Soc., 2018, 140, 10734–10739 CrossRef CAS.
- H. Gao, Z. Gao, D. Jiao, J. Zhang, X. Li, Q. Tang, Y. Shi and D. Ding, Boosting room temperature phosphorescence performance by alkyl modification for intravital orthotopic lung tumor imaging, Small, 2021, 2005449 CrossRef CAS.
- M. Fang, J. Yang, X. Xiang, Y. Xie, Y. Dong, Q. Peng, Q. Li and Z. Li, Unexpected room-temperature phosphorescence from a non-aromatic, low molecular weight, pure organic molecule through the intermolecular hydrogen bond, Mater. Chem. Front., 2018, 2, 2124–2129 RSC.
- J. Yang, H. Gao, Y. Wang, Y. Yu, Y. Gong, M. Fang, D. Ding, W. Hu, B. Z. Tang and Z. Li, The odd-even effect of alkyl chain in organic room temperature phosphorescence luminogens and the corresponding in vivo imaging, Mater. Chem. Front., 2019, 3, 1391–1397 RSC.
- Y. Tao, R. Chen, H. Li, J. Yuan, Y. Wan, H. Jiang, C. Chen, Y. Si, C. Zheng, B. Yang, G. Xing and W. Huang, Resonance-activated spin-flipping for efficient organic ultralong room-temperature phosphorescence, Adv. Mater., 2018, 30, 1803856 CrossRef PubMed.
- Q. Li and Z. Li, Molecular packing: another key point for the performance of organic and polymeric optoelectronic materials, Acc. Chem. Res., 2020, 53, 962–973 CrossRef CAS.
- Y. Fan, Q. Li and Z. Li, Organic luminogens bearing alkyl substituents: design flexibility, adjustable molecular packing, and the optimized performance, Mater. Chem. Front., 2021, 5, 1525–1540 RSC.
- C. Wang, Y. Yu, Z. Chai, F. He, C. Wu, Y. Gong, M. Han, Q. Li and Z. Li, Recyclable mechanoluminescent luminogen: different polymorphs, different self-assembly effects of the thiophene moiety and recovered molecular packing via simple thermal-treatment, Mater. Chem. Front., 2019, 3, 32–38 RSC.
- F. Liu, S. Bi, X. Wang, X. Leng, M. Han, B. Xue, Q. Li, H. Zhou and Z. Li, Similar or different: the same Spiro-core but different alkyl chains with apparently improved device performance of perovskite solar cells, Sci. China: Chem., 2019, 62, 739–745 CrossRef CAS.
- M. Fang, J. Yang and Z. Li, Recent advances in purely organic room temperature phosphorescence polymer, Chin. J. Polym. Sci., 2019, 37, 383–393 CrossRef CAS.
- A. Forni, E. Lucenti, C. Botta and E. Cariati, Metal free room temperature phosphorescence from molecular self-interactions in the solid state, J. Mater. Chem. C, 2018, 6, 4603–4626 RSC.
- Kenry, C. Chen and B. Liu, Enhancing the performance of pure organic room-temperature phosphorescent luminophores, Nat. Commun., 2019, 10, 2111 CrossRef CAS.
- S. Hirata, Recent advances in materials with room-temperature phosphorescence: photophysics for triplet exciton stabilization, Adv. Opt. Mater., 2017, 5, 1700116 CrossRef.
- Q. Li and Z. Li, The strong light-emission materials in the aggregated state: what happens from a single molecule to the collective group, Adv. Sci., 2017, 4, 1600484 CrossRef PubMed.
- W. Zhao, Z. He and B. Z. Tang, Room-temperature phosphorescence from organic aggregates, Nat. Rev. Mater., 2020, 5, 869–885 CrossRef CAS.
- W. Z. Yuan, X. Y. Shen, H. Zhao, J. W. Y. Lam, L. Tang, P. Lu, C. Wang, Y. Liu, Z. Wang, Q. Zheng, J. Z. Sun, Y. Ma and B. Z. Tang, Crystallization-induced phosphorescence of pure organic luminogens at room temperature, J. Phys. Chem. C, 2010, 114, 6090–6099 CrossRef CAS.
- Y. Wang, J. Yang, Y. Tian, M. Fang, Q. Liao, L. Wang, W. Hu, B. Z. Tang and Z. Li, Persistent organic room temperature phosphorescence: what is the role of molecular dimers?, Chem. Sci., 2020, 11, 833–838 RSC.
- Y. Wang, J. Yang, M. Fang, Y. Yu, B. Zou, L. Wang, Y. Tian, J. Cheng, B. Z. Tang and Z. Li, Förster resonance energy transfer: an efficient way to develop stimulus-responsive room-temperature phosphorescence materials and their applications, Matter, 2020, 3, 449–463 CrossRef.
- R. Kabe and C. Adachi, Organic long persistent luminescence, Nature, 2017, 550, 384–387 CrossRef CAS.
- K. Jinnai, R. Kabe and C. Adachi, Wide-range tuning and enhancement of organic long-persistent luminescence using emitter dopants, Adv. Mater., 2018, 30, 1800365 CrossRef PubMed.
- W. Zhao, Z. He, J. W. Y. Lam, Q. Peng, H. Ma, Z. Shuai, G. Bai, J. Hao and B. Z. Tang, Rational molecular design for achieving persistent and efficient pure organic room-temperature Phosphorescence, Chem, 2016, 1, 592–602 CAS.
- C. Zhou, S. Zhang, Y. Gao, H. Liu, T. Shan, X. Liang, B. Yang and Y. Ma, Ternary emission of fluorescence and dual phosphorescence at room temperature: a single-molecule white light emitter based on pure organic Aza-aromatic material, Adv. Funct. Mater., 2018, 28, 1802407 CrossRef.
- J. Yuan, S. Wang, Y. Ji, R. Chen, Q. Zhu, Y. Wang, C. Zheng, Y. Tao, Q. Fan and W. Huang, Invoking ultralong room temperature phosphorescence of purely organic compounds through H-aggregation engineering, Mater. Horiz., 2019, 6, 1259–1264 RSC.
- Y. Yang, Y. Liu, Y. Liu and K. Jiang, Structure-lock induced phosphorescence lifetime enhancing of (9H-carbazol-9-yl) (phenyl)methanone: an organic phosphorescent materials, J. Lumin., 2020, 227, 117587 CrossRef CAS.
- M. Baroncini, G. Bergamini and P. Ceroni, Rigidification or interaction-induced phosphorescence of organic molecules, Chem. Commun., 2017, 53, 281–293 RSC.
- Y. Xie, Y. Ge, Q. Peng, C. Li, Q. Li and Z. Li, How the molecular packing affects the room temperature phosphorescence in pure organic compounds: ingenious molecular design, detailed crystal analysis, and rational theoretical calculations, Adv. Mater., 2017, 29, 1606829 CrossRef PubMed.
- Q. Liao, Q. Gao, J. Wang, Y. Gong, Q. Peng, Y. Tian, Y. Fan, H. Guo, D. Ding, Q. Li and Z. Li, 9,9-Dimethylxanthene derivatives with room-temperature phosphorescence: substituent effects and emissive properties, Angew. Chem., Int. Ed., 2020, 59, 9946–9951 CrossRef CAS.
- J. A. Li, J. Zhou, Z. Mao, Z. Xie, Z. Yang, B. Xu, C. Liu, X. Chen, D. Ren, H. Pan, G. Shi, Y. Zhang and Z. Chi, Transient and persistent room-temperature mechanoluminescence from a white-light-emitting AIEgen with tricolor emission switching triggered by light, Angew. Chem., Int. Ed., 2018, 57, 6449–6453 CrossRef CAS PubMed.
- Z. Yang, E. Ubba, Q. Huang, Z. Mao, W. Li, J. Chen, J. Zhao, Y. Zhang and Z. Chi, Enabling dynamic ultralong organic phosphorescence in molecular crystals through the synergy between intramolecular and intermolecular interactions, J. Mater. Chem. C, 2020, 8, 7384–7392 RSC.
- J. Yang, X. Zhen, B. Wang, X. Gao, Z. Ren, J. Wang, Y. Xie, J. Li, Q. Peng, K. Pu and Z. Li, The influence of the molecular packing on the room temperature phosphorescence of purely organic luminogens, Nat. Commun., 2018, 9, 840 CrossRef PubMed.
- Y. Lei, W. Dai, Y. Tian, J. Yang, P. Li, J. Shi, B. Tong, Z. Cai and Y. Dong, Revealing insight into long-lived room-temperature phosphorescence of host-guest systems, J. Phys. Chem. Lett., 2019, 10, 6019–6025 CrossRef CAS.
- J. Yang, X. Gao, Z. Xie, Y. Gong, M. Fang, Q. Peng, Z. Chi and Z. Li, Elucidating the excited state of mechanoluminescence in organic luminogens with room-temperature phosphorescence, Angew. Chem., Int. Ed., 2017, 56, 15299–15303 CrossRef CAS.
- J. Wang, C. Wang, Y. Gong, Q. Liao, M. Han, T. Jiang, Q. Dang, Y. Li, Q. Li and Z. Li, Bromine-substituted fluorene: molecular structure, Br-Br interactions, room-temperature phosphorescence, and tricolor triboluminescence, Angew. Chem., Int. Ed., 2018, 57, 16821–16826 CrossRef CAS.
- Q. Zhang, B. Li, S. Huang, H. Nomura, H. Tanaka and C. Adachi, Efficient blue organic light-emitting diodes employing thermally activated delayed fluorescence, Nat. Photonics, 2014, 8, 326–332 CrossRef CAS.
- M. Han, Y. Chen, Y. Xie, F. Zhang, X. Li, A. Huang, Y. Fan, Y. Fan, Y. Gong, Q. Peng, Q. Li, D. Ma and Z. Li, 1.42-fold enhancement of blue OLED device performance by simply changing alkyl groups on the acridine ring, Cell Rep. Phys. Sci., 2020, 1, 100252 CrossRef.
- Y. Liu, C. Li, Z. Ren, S. Yan and M. R. Bryce, All-organic thermally activated delayed fluorescence materials for organic light-emitting diodes, Nat. Rev. Mater., 2018, 3, 18020 CrossRef CAS.
- J. Wang, Z. Chai, J. Wang, C. Wang, M. Han, Q. Liao, A. Huang, P. Lin, C. Li, Q. Li and Z. Li, Mechanoluminescence or room-temperature phosphorescence: molecular packing-dependent emission response, Angew. Chem., Int. Ed., 2019, 58, 17297–17302 CrossRef CAS PubMed.
- L. Xiao and H. Fu, Enhanced room-temperature Phosphorescence through Intermolecular Halogen/Hydrogen Bonding, Chem. – Eur. J., 2018, 25, 714–723 CrossRef.
- Q. Huang, X. Mei, Z. Xie, D. Wu, S. Yang, W. Gong, Z. Chi, Z. Lin and Q. Ling, Photo-induced phosphorescence and mechanoluminescence switching in a simple purely organic molecule, J. Mater. Chem. C, 2019, 7, 2530–2534 RSC.
- L. Huang, B. Chen, X. Zhang, C. O. Trindle, F. Liao, Y. Wang, H. Miao, Y. Luo and G. Zhang, Proton-activated “Off-On” room-temperature phosphorescence from purely organic Thioethers, Angew. Chem., 2018, 130, 16278–16282 CrossRef.
- X. Chen, C. Xu, T. Wang, C. Zhou, J. Du, Z. Wang, H. Xu, T. Xie, G. Bi, J. Jiang, X. Zhang, J. N. Demas, C. O. Trindle, Y. Luo and G. Zhang, Versatile room-temperature-phosphorescent materials prepared from N-Substituted naphthalimides: emission enhancement and chemical conjugation, Angew. Chem., 2016, 128, 10026–10030 CrossRef.
- F. Liao, J. Du, X. Nie, Z. Wu, H. Su, W. Huang, T. Wang, B. Chen, J. Jiang, X. Zhang and G. Zhang, Modulation of red organic room-temperature phosphorescence in heavy atom-free phosphors, Dyes Pigm., 2021, 193, 109505 CrossRef CAS.
- J. Yang, Z. Ren, B. Chen, M. Fang, Z. Zhao, B. Z. Tang, Q. Peng and Z. Li, Three polymorphs of one luminogen: how the molecular packing affects the RTP and AIE properties?, J. Mater. Chem. C, 2017, 5, 9242–9246 RSC.
- J. Yang, Z. Chi, W. Zhu, B. Z. Tang and Z. Li, Aggregation-induced emission: a coming-of-age ceremony at the age of eighteen, Sci. China: Chem., 2019, 62, 1090–1098 CrossRef CAS.
- F. Liu, Q. Liao, J. Wang, Y. Gong, Q. Dang, W. Ling, M. Han, Q. Li and Z. Li, Intermolecular electronic coupling of 9-methyl-9H-dibenzo[a,c] carbazole for strong emission in aggregated state by substituent effect, Sci. China: Chem., 2020, 63, 1435–1442 CrossRef CAS.
- B. Zhou and D. Yan, Excitation-dependent organic persistent luminescence, Sci. China: Chem., 2020, 63, 423–425 CrossRef CAS.
- R. Liu, Z. Zhou, Y. Liu, Z. Liang, Y. Ming, T. Hao and Y. Nie, Differences in crystallization behaviors between cyclic and linear polymer nanocomposites, Chin. J. Polym. Sci., 2020, 38, 1034–1044 CrossRef CAS.
Footnote |
† Electronic supplementary information (ESI) available. CCDC 2081904–2081906. For ESI and crystallographic data in CIF or other electronic format see DOI: 10.1039/d1qm01184g |
|
This journal is © the Partner Organisations 2022 |