DOI:
10.1039/D1QM01303C
(Research Article)
Mater. Chem. Front., 2022,
6, 40-51
Acceptor modulation for blue and yellow TADF materials and fabrication of all-TADF white OLED†
Received
24th September 2021
, Accepted 8th November 2021
First published on 8th November 2021
Abstract
4,4′-(4-Phenylpyridine-2,6-diyl)dibenzonitrile (PyDCN) was designed to reduce the electron-withdrawing strength and to decentralize the unsaturated N atoms relative to triazine. PyDCN was used as an acceptor to construct thermally activated delayed fluorescence (TADF) emitters PyDCN–PXZ and PyDCN–DMAC with phenoxazine (PXZ) and 9,9-dimethyl-9,10-dihydroacridine (DMAC) as electron donors. The relatively high radiative transition rate constants (kF) in the order of 107 s−1, high photoluminescence quantum yields in the range of 80–90%, and balanced charge transportation were detected for both TADF emitters. PyDCN–DMAC exhibited electroluminescence (EL) with a peak at 472 nm and CIE coordinates of (0.17, 0.26), more approaching to real blue in comparison with most of DMAC based sky-blue TADF analogues. A high external quantum yield (ηext) of 25.6% was obtained for a PyDCN–DMAC doped organic light-emitting diode (OLED). PyDCN–PXZ exhibited green EL with a ηext of 26.9% at 519 nm in the doped OLED. Its non-doped yellow OLED also revealed a moderate ηext of 18.2% with a peak at 557 nm. Furthermore, PyDCN–DMAC was used as the blue host emitter to fabricate a single-emitting-layer all-TADF white OLED in combination with a yellow home-made TADF emitter PP–PXZ, which exhibited a maximum ηext of 18.5% with CIE coordinates of (0.38, 0.44) and excellent color stability.
Introduction
White organic light-emitting diodes (WOLEDs) have been developed rapidly in recent years due to their applications in solid-state lighting source and full color flat-panel displays with favorable advantages, such as high emission efficiency, homogeneous large-area emission, superior white color balance and low energy consumption.1–5 To achieve a high color rendering index (CRI) and high efficiency simultaneously, two-color and three-color multilayer WOLEDs with complex device structures are fabricated.6–8 However, WOLEDs using a single-emitting layer (SEL) have been widely developed in recent years, which can reduce the structural complexity and processing cost as well as improve device stability.9,10 The all-phosphorescent and fluorescent/phosphorescent (F–P) hybrid WOLEDs are the typical approaches to achieve theoretical maximum internal quantum efficiency. However, all-phosphorescent devices based on noble metal (such as Ir, Pt, and Au) complexes still suffer from the intrinsic deficiencies including rare metal reliance, color impurity, and fragile operational stability for blue phosphors.11–13 The typical fluorescent host/phosphorescent dye (F/P) hybrid WOLEDs combine emission from a blue fluorophor and a green/red (orange) phosphor. This strategy is intrinsically superior to the fully fluorescent or phosphorescent approach because it combines the merits of long-term stability of fluorophors and high efficiency of phosphors, respectively.14–17 In hybrid WOLEDs, all the singlet excitons (25%) can be utilized by the fluorophor to give blue emission, while the triplet excitons (75%) generally diffuse into the adjacent phosphorescent layer to give out phosphorescence. In this way, all the electrically generated excitons can be harvested to reach high internal quantum efficiency. Nevertheless, the lack of blue fluorescent emitters with both high triplet state energy (T1) and high photoluminescence quantum yield (PLQY) greatly limits the performance of hybrid WOLEDs.18,19 Furthermore, in the hybrid WOLEDs, the triplet excitons formed on the host molecules diffuse into the host molecules near the dopants and then transfer the energy onto the dopant molecules through the Dexter energy transfer mechanism. During the diffusion process, parts of the triplet excitons are inevitably deactivated through other interactions, such as triplet–triplet annihilation (TTA) or triplet-polaron annihilation (TPA).20–22 Meanwhile, these hybrid WOLEDs usually suffer from unbalanced white spectra and poor color stability because the radiative decay rate of triplet excitons (T1) in phosphors is faster than that of singlet excitons (S1) in fluorophors.23
Recently, thermally activated delayed fluorescence (TADF) that was used as a new mechanism in OLEDs has achieved high efficiency, comparable to phosphorescence.24–26 With extremely small energy differences (ΔEST) between the lowest singlet and triplet excited states (S1 and T1), TADF emitters can efficiently harvest both singlet and triplet excitons via the reverse intersystem crossing (RISC) process for light emission.27–29 And the tiny ΔEST can be obtained by sufficiently separating the highest occupied molecular orbital (HOMO) and the lowest unoccupied molecular orbital (LUMO) through combining electron–donor (D) and electron–acceptor (A) moieties with highly twisted structures.30–33 This kind of D–A structures are also advantageous to achieve efficient bipolar carrier transport. Ever since Adachi's group first reported WOLEDs based on TADF emitters,34 TADF emitters have been widely used for WOLEDs to achieve a high CRI and high efficiencies.15–17 The all-fluorescence WOLEDs based on blue TADF host emitters have provided an alternative for the realization of highly efficient white emission, where more efficient energy transfer is achieved because a portion of the generated non-radiative triplet excitons can be up-converted to radiative singlet excitons via a RISC process induced by sufficiently small ΔEST, and then the energy of the host singlet can be transferred to the TADF dopant through the long-range-interaction Förster energy transfer at very low dopant concentrations of ≤1%. Compared with TADF/phosphorescence hybrid WOLEDs, the all-TADF WOLEDs consisting of a blue TADF host and an orange/red TADF/fluorescent dopant hold a great promise owing to the potential for full exciton utilization and broad spectral range with pure organic fluorescence emitters. Wang's group recently developed a blue TADF emitter o,o′-NPh2 and fabricated a bi-component all-TADF WOLED by doping a yellow TADF emitter TXO–PhCz4 in o,o′-NPh2 at a low concentration of 0.5 wt%, which exhibited a maximum EL efficiency of 25.4 cd A−1, 21.7 lm W−1 and 10.6% with CIE coordinates of (0.35, 0.36).35 Zhao's group developed a sky-blue TADF emitter DCB–BP–SFAC and also fabricated a bi-component emitting-layer WOLED with a red TADF emitter TPA–AQ, providing white light emission with CIE coordinates of (0.47, 0.45), a moderate CRI of 56, and a maximum EQE of 19.1%.36 Xu's group fabricated single-emitting-layer all-TADF WOLEDs by combining yellow 4CzTPNBu and blue ptBCzPO2TPTZ, providing white light emission with a maximum EQE of 23.6%, CIE coordinates of (0.34, 0.36) and a high CRI of 87.37 However, this all-TADF WOLED exhibited serious efficiency roll-off and poor color stability owing to unbalanced carrier transport. Therefore, there is still a long road to improve the performance of all-TADF WOLEDs, for example through developing new bipolar blue TADF materials to act as host emitters for simple-structure WOLEDs.
Herein, a novel acceptor was designed to construct novel TADF emitters, especially a blue TADF emitter for use in all-TADF WOLEDs. Triazine is a widely used electron acceptor in the development of various color TADF emitters. When combined with acridine or its derivatives, the triazine–acridine TADF materials emit sky-blue luminescence with fluorescence peaks in the typical range of 480–500 nm, rather than real blue.38,39 This is obviously because of the relatively strong electron-withdrawing ability of the triazine acceptor and the strong electron-donating ability of acridine donors and thus the strong intramolecular charge transfer (ICT) effect. As shown in Scheme 1, three sp2 N atoms are all centered on the triazine ring, resulting in a relatively strong and centralized electron deficient feature of triazine. In order to tune the emission color of TADF emitters more approaching to blue, two phenylnitrile were introduced onto the 2,6-sites of the pyridine ring to design 4,4′-(4-phenylpyridine-2,6-diyl)dibenzonitrile (PyDCN) as an electron acceptor. In comparison with triazine, both phenylnitrile and pyridine are less electron deficient. With the electron deficient functional groups (CN and pyridine) distributed and decentralized onto three different aromatic rings, the PyDCN group is less electron withdrawing than triazine, as verified by the more negative electrochemical reduction potential (−1.54 V) of PyDCN relative to that of triazine (−1.32 V). At the same time, the presence of the CN groups in PyDCN would enlarge the LUMO distribution, which should be favorable for radiative transition and high PL quantum yields of the generated D–A type molecules.40 PyDCN was used as an acceptor to design two novel TADF emitters in combination with 9,9-dimethyl-9,10-dihydroacridine (DMAC) and phenoxazine (PXZ) as the electron donors, namely 4,4′-(4-(4-(9,9-dimethylacridin-10(9H)-yl)phenyl)pyridine-2,6-diyl)dibenzonitrile (PyDCN–DMAC) and 4,4′-(4-(4-(10H-phenoxazin-10-yl)phenyl)pyridine-2,6-diyl)dibenzonitrile (PyDCN-PXZ) (Scheme 2). PyDCN–DMAC exhibited sky blue fluorescence with a photoluminescence (PL) peak at 480 nm in toluene solution, which is hypsochromic shifted by at least 10 nm in comparison with the analogue with triazine as the acceptor.38 PyDCN–PXZ exhibits green PL with an emission peak at 520 nm. It is interesting to observe that both PyDCN–DMAC and PyDCN–PXZ exhibit a relatively high radiative transition rate constant (kF) in the order of 107 s−1, which is mainly ascribed to the decentralized acceptor. PyDCN–PXZ and PyDCN–DMAC were used as the doped and non-doped emitters to fabricate monochromic OLEDs. PyDCN–PXZ exhibited green and yellow EL with maximum external quantum efficiencies (ηext) of 26.9% and 18.2% in doped and non-doped devices, respectively, while PyDCN–DMAC realized sky-blue emission with a maximum ηext of 25.6% in doped OLED. Furthermore, PyDCN–DMAC was used as a blue host emitter to fabricate a single-emitting-layer all-TADF WOLED together with a home-made orange TADF emitter 10,10′-(pyrido[2,3-b]pyrazine-2,3-diylbis(4,1-phenylene))bis(10H-phenoxazine) (PP–PXZ). The all-TADF WOLED exhibited a maximum ηext of 18.5% with CIE coordinates of (0.38, 0.44) and a CRI of 73, which are higher than the efficiencies of both the blue doped device of PyDCN–DMAC or the orange doped device of PP–PXZ.
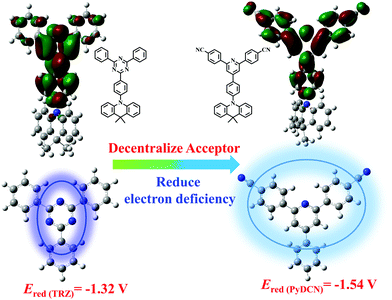 |
| Scheme 1 Comparison of the chemical structures and electron deficient atoms distribution between triazine and PyDCN. | |
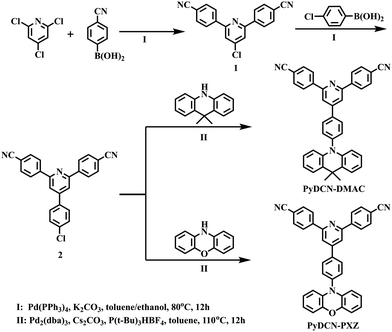 |
| Scheme 2 Chemical structures and the synthetic routes of PyDCN–DMAC and PyDCN–PXZ. | |
Results and discussion
Design, synthesis and thermal properties
As shown in Fig. S1 in the ESI,† the designed acceptor PyDCN can be reduced at a more negative potential (−1.54 eV) than triazine (−1.32 eV) during the cathodic scan, proving that PyDCN has a weaker electron withdrawing strength than triazine. This important character would be helpful to make the fluorescence of the TADF molecules containing PyDCN as the acceptor shift to the blue direction. PyDCN–DMAC and PyDCN–PXZ were designed by linking DMAC and PXZ donors with PyDCN acceptor. The synthetic procedures of PyDCN–DMAC and PyDCN–PXZ are presented in Scheme 2. The intermediates 4,4′-(4-chloropyridine-2,6-diyl)dibenzonitrile (1) and 4,4′-(4-(4-chlorophenyl)pyridine-2,6-diyl)dibenzonitrile (2) were synthesized by a tetrakis(triphenylphosphine)-palladium(0) mediated typical Suzuki cross-coupling reaction. PyDCN–DMAC and PyDCN–PXZ were prepared with yields in the range of 40–50% through a C–N coupling reaction in the presence of the Pd2(dba)3 catalyst. In addition, the two target compounds have good solubilities in most of common organic solvents; so they can be thoroughly purified by column chromatography and repeated recrystallization to achieve a high purity for OLED applications. 1H and 13C NMR spectroscopy, mass spectrometry and elemental analysis (provided in the supporting information) were used to characterize the molecular structures.
The thermal and morphological stabilities of these compounds were investigated using thermogravimetric analysis (TGA) and differential scanning calorimetry (DSC), respectively. The TGA thermograms are shown in Fig. S2 (ESI†); the DSC traces are shown in Fig. 1, and all the relevant data are summarized in Table 1. PyDCN–DMAC and PyDCN–PXZ showed thermal decomposition temperatures (Td) at 398 and 436 °C (corresponding to 5% weight loss), respectively, indicating good thermal stability. As shown in Fig. 1, the DSC traces of PyDCN–DMAC and PyDCN–PXZ revealed well-defined glass transition temperatures (Tg) of 140 and 138 °C, respectively, indicating that these materials could form stable amorphous films during thermal evaporation in the process of OLED fabrication, and stable OLEDs that can survive for long time under operation could also be expected.
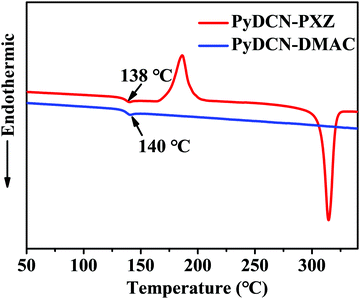 |
| Fig. 1 DSC traces (at the second heating cycle) of PyDCN–PXZ and PyDCN–DMAC recorded at a heating rate of 10 °C min−1. | |
Table 1 Summary of physical parameters of PyDCN–PXZ and PyDCN–DMAC
Compound |
λ
abs
[nm] |
λ
em
[nm] |
E
S
[eV] |
E
T
[eV] |
ΔESTc [eV] |
HOMO/LUMOd [eV] |
T
d/Tge [°C] |
Φ
PL
[%] |
Absorption and fluorescence peak wavelengths in dilute toluene solutions.
Determined from the LT fluorescence and phosphorescence spectra in 2-MeTHF at 77 K.
Energy split between S1 and T1 estimated as ΔEST = ES − ET.
HOMO and LUMO energies were determined from the onset potential of the first oxidation and reduction wave.
Decomposition temperature (Td) at 5 wt% weight loss obtained from TGA measurements, and glass transition temperature (Tg) obtained from DSC measurements.
Φ
PL, PLQY of the doped films of PyDCN–PXZ:CBP (15 wt%) and PyDCN–DMAC:PPF (10 wt%).
|
PyDCN–PXZ |
282/320 |
532 |
2.71 |
2.65 |
0.06 |
−5.06/−2.94 |
436/138 |
89.6 |
PyDCN–DMAC |
283/318 |
480 |
2.99 |
2.98 |
0.01 |
−5.21/−2.95 |
398/140 |
82.8 |
Computational simulation and electrochemical properties
The geometry optimization of PyDCN–DMAC and PyDCN–PXZ in the ground states (S0) was performed by using density functional theory (DFT) with the B3LYP/6-31G* basis set. The geometries and frontier molecular orbitals of the S0 state for both compounds are shown in Fig. 2a. In both molecules, the DMAC and PXZ rings have large dihedral angles of 87.8° and 88.6° to the adjacent phenylene ring, indicating the highly twisted molecular conformations of both compounds. The HOMOs of both compounds are mainly centered on the donor units of DMAC and PXZ, and slightly extended to the phenylene linkers, while the LUMOs are mainly located on the acceptor unit, spreading from the central pyridinyl ring to the surrounding phenylene and phenylnitrile rings. In comparison with the reported analogue containing triphenyltriazine as the acceptor and DMAC as the donor,38 the LUMO of PyDCN–DMAC is further extended onto the CN groups. The enlarged LUMO distribution should influence the transition dipole moment of these two molecules. The small spatial overlap between HOMO and LUMO is conducive to obtain small singlet and triplet energy gaps (ΔEST). The natural transition orbitals (NTOs) of their optimized S1 and T1 states were further investigated using time-dependent DFT (TD-DFT). As shown in Fig. S3 (ESI†), similar to the FMO location of their ground states, the hole and particle of both molecules are obviously distributed on their D and A segments, respectively, demonstrating their intramolecular charge-transfer (ICT) characteristics, which correspond experimentally to the solvatochromic properties of both compounds (Fig. S4, ESI†). It is also observed that the S1 and T1 NTOs share similar spatial distributions of their hole and particle, which should be conducive to the easy RISC processes and the relatively high RISC rate.39
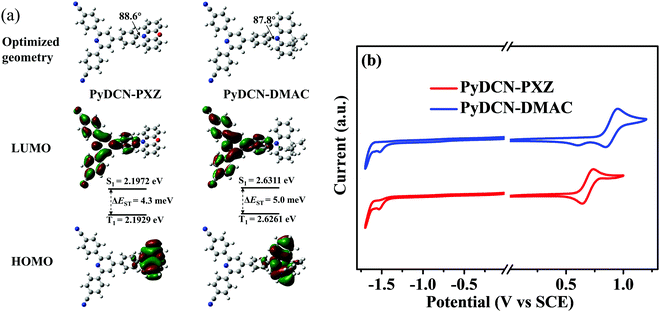 |
| Fig. 2 Spatial distributions of HOMO and LUMO, geometry optimized structures in the ground state, and the calculated energies for the S1 and T1 states (a), and the cyclic voltammograms (b) of PyDCN–DMAC and PyDCN–PXZ. | |
The electrochemical behaviors of PyDCN–DMAC and PyDCN–PXZ were studied by cyclic voltammetry (CV) measurements in deoxygenated CH2Cl2 (for anodic scan) and anhydrous DMF (for cathodic scan) solutions containing 0.1 M tetra-(n-butyl)ammonium hexafluorophosphate (n-Bu4NPF6) as the supporting electrolyte. The cyclic voltammograms are shown in Fig. 2b. These two compounds exhibit distinct oxidation and reduction behaviors, obviously indicating their bipolar electrochemical properties. The HOMO energies of PyDCN–DMAC and PyDCN–PXZ were determined from the onset potential of the first oxidation wave (Eonsetox) according to the equation EHOMO = −e(Eonsetox + 4.4) as −5.21 eV and −5.06 eV, respectively.17 In a similar way, the LUMO energies were determined from the onset potential of the first reduction wave (Eonsetred) according to the equation ELUMO = −e(Eonsetred + 4.4) to be −2.95 eV and −2.94 eV for PyDCN–DMAC and PyDCN–PXZ, respectively.17 Compared to PyDCN–DMAC, the stronger electron-donating feature of PXZ shifts the HOMO level of PyDCN–PXZ shallower by 0.15 eV, finally resulting in the smaller band gap and the possible red shift of the fluorescence for PyDCN–PXZ based on their same acceptor and almost identical LUMO levels.
Photophysical properties
The UV-vis absorption and photoluminescence (PL) spectra of PyDCN–DMAC and PyDCN–PXZ were measured in dilute toluene solutions and are shown in Fig. 3, and all the photophysical data are summarized in Table 1. Due to their similar D–A structures and acceptor units, the absorption bands of both the compounds are similar. The strong absorption bands of these two compounds at 270–320 nm could be assigned to the π–π* transition, while the very weak and broad absorption band at around 360–440 nm is attributed to the intramolecular charge-transfer (ICT) transition from the DMAC or PXZ moiety to the acceptor moiety. Upon photoexcitation, both PyDCN–DMAC and PyDCN–PXZ exhibit broad and featureless fluorescence spectra, emitting sky-blue and green fluorescence with the peaks at 480 and 532 nm, respectively. The emission peak of PyDCN–PXZ is redshifted by 52 nm as compared to that of PyDCN–DMAC, consistent with the smaller band gap observed in the electrochemical measurement due to the stronger electron-donating nature of the PXZ donor. As shown in Fig. S4 (ESI†), with gradually increasing solvent polarity, the fluorescence spectra of PyDCN–DMAC and PyDCN–PXZ exhibited a regular bathochromic shift, further confirming the ICT properties of both the compounds. The absorption and PL spectra of these two compounds in the doped films in bis-(diphenylphosphoryl)-dibenzo[b,d]-furan (PPF) and 4,4′-di(9H-carbazol-9-yl)-1,1′-biphenyl (CBP) hosts (Fig. S5, ESI†) are similar in both profiles and positions to those in dilute toluene solutions. The PL quantum yields (PLQYs, ΦPL) of the neat films of PyDCN–DMAC and PyDCN–PXZ were measured to be 39.8% and 58.1%, respectively. The ΦPLs of the co-deposited films of 10 wt% PyDCN–DMAC:PPF and 15 wt% PyDCN–PXZ:CBP were greatly increased to 82.8% and 89.6%, respectively.
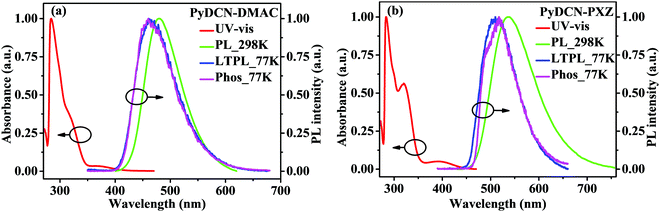 |
| Fig. 3 UV-vis absorption and PL spectra of (a) PyDCN–DMAC and (b) PyDCN–PXZ in dilute toluene solutions at room temperature, and their LTPL spectra and phosphorescence spectra in frozen 2-MeTHF at 77 K. | |
The low-temperature (LT) PL and phosphorescence of these two compounds were measured in a frozen 2-methyltetrahydrofuran (2-Me-THF) matrix at 77 K, and the spectra are shown in Fig. 3. It is obvious that both the LT fluorescence and phosphorescence of these two compounds are both blue shifted as compared with their room-temperature fluorescence. The energy levels of S1 and T1 were determined from the onset wavelength (shortest-wavelength) of the LT fluorescence and phosphorescence spectra to be 2.99 (2.98) and 2.71 (2.65) eV for PyDCN–DMAC and PyDCN–PXZ, respectively. Therefore, the ΔEST values of PyDCN–DMAC and PyDCN–PXZ were estimated to be 0.01 and 0.06 eV, respectively. Apparently these ΔEST values are small enough for an efficient RISC process from T1 to S1, revealing their high potential as TADF emitters.
In order to further demonstrate the TADF properties of these emitters, time-resolved transient PL decay curves were measured by the multichannel scanning (MCS) method using a 365 nm EPL laser as the excitation source for the doped films PyDCN–DMAC:PPF (10 wt%) and PyDCN–PXZ:CBP (15 wt%) (Fig. S6 a and b, ESI†). The decay curves follow the bi-exponential style, indicating that the PL of these films includes two emitting components, one is the nanosecond component that would be the prompt fluorescence (PF), and the other is the microsecond component. Using the time correlated single photon counting (TCSPC) technique with a 375 nm picosecond laser as the excitation source (Fig. S6c and d, ESI†), the lifetimes of PF (τPF) were detected to be 14.8 and 22.1 ns for PyDCN–DMAC and PyDCN–PXZ, respectively. At the same time, the emission spectra at a delayed time of 10 μs (Fig. S7, ESI†) show identical wavelength and spectral profiles to the PF spectra of each compound, suggesting that these microsecond components should originate from the same singlet excited states as the corresponding PF, i.e. they are the DF of PyDCN–DMAC and PyDCN–PXZ.17 From the transient PL decay curves of these compounds obtained by the MCS method (Fig. S6a and b, ESI†), the lifetimes of DF (τDF) were detected to be 5.6 and 6.4 μs for PyDCN–DMAC and PyDCN–PXZ, respectively. The DF lifetimes of both compounds are relatively short in the microsecond scale, indicating that an efficient up-conversion from T1 to S1 can be expected mainly due to smaller ΔEST.39,40 In order to further disclose the mechanism of DF for both the compounds, the temperature-dependent transient PL decay of PyDCN–DMAC and PyDCN–PXZ in doped films was studied (Fig. 4). With increasing temperature from 100 to 300 K, both the two doped films exhibit enhanced fluorescence intensity, especially in the first 50 μs range. This proves that the DF can be activated by thermal energy, confirming the observed DF of these two compounds are TADF.
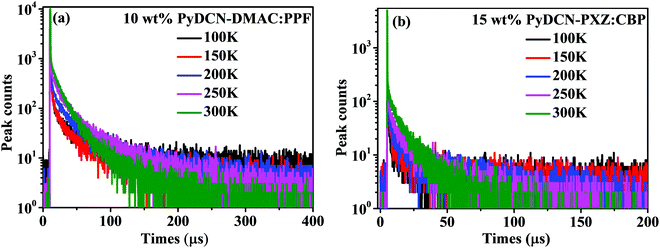 |
| Fig. 4 Temperature-dependent transient decay spectra of (a) 10 wt% PyDCN–DMAC:PPF film and (b) 15 wt% PyDCN–PXZ:CBP film. | |
The rate constants of RISC (kRISC), fluorescence decay (kF), intersystem crossing (kISC) and more processing related to the excited state kinetics for these two compounds were calculated from the PLQYs and lifetimes of the decay curves and are summarized in Table S1 in the ESI.† The kRISCs were calculated to be 4.6 × 105 and 5.1 × 105 s−1 for PyDCN–DMAC and PyDCN–PXZ, respectively, which are higher than those of many typical TADF molecules. The fast RISC process suggests an efficient T1–S1 exciton up-conversion, which is conducive to the efficient utilization of triplet excitons for light emission. At the same time, PyDCN–DMAC and PyDCN–PXZ exhibit relatively high kFs values of 2.13 × 107 and 1.48 × 107 s−1, indicating an efficient radiative decay process. In comparison with the analogue DMAC–TRZ that contains triazine as the electron acceptor and exhibits a kF of 1.20 × 107 s−1,39 the incorporation of PyDCN in PyDCN–DMAC almost doubled the kF to 2.13 × 107 s−1. Evidently the enhanced kF can be ascribed to the enlarged and decentralized LUMO distribution on the whole PyDCN moiety as compared with the triazine.
Carrier-transport properties
To investigate the charge-transporting character of PyDCN–DMAC and PyDCN–PXZ, single-charge devices were fabricated using PyDCN–DMAC and PyDCN–PXZ as the major functional layer. The configuration of hole-only devices was ITO/poly-(3,4-ethylenedioxythiophene):polystyrene sulfonate (PEDOT:PSS, 40 nm)/1,1-bis[4-[N,N-di(p-tolyl)-amino]phenyl]cyclohexane (TAPC, 5 nm)/TADF compounds (60 nm)/TAPC (5 nm)/Al (200 nm), and the structure of electron-only devices was ITO/1,3,5-tri-[(3-pyridyl)-phen-3-yl]benzene (TmPyPB, 5 nm)/TADF compounds (60 nm)/TmPyPB (5 nm)/LiF (1 nm)/Al (200 nm). Fig. S8 (ESI†) illustrates the chemical structures of the related materials and their energy levels. The TAPC/Al and ITO/TmPyPB interfaces were designed to avoid electron- and hole-injection from the cathode and anode owing to large electron and hole barriers of 2.3 eV and 1.88 eV, respectively.41 The thickness of the TADF layer was much greater than that of the adjacent layers to reflect the inherent charge transport capability of the PyDCN–DMAC and PyDCN–PXZ layer and reduce the possible influence from adjacent layers.42 The current density–voltage (J–V) curves of these single-carrier devices are shown in Fig. 5. Both the hole-only and electron-only devices of each compound exhibited significantly high hole current densities and electron current densities in the voltage range suitable for OLEDs, indicating the good bipolar charge-transporting abilities of both compounds. More importantly, the hole current and electron current of each compound are quite close to each other at any given voltage, indicating that each compound has balanced hole- and electron-transporting capabilities. This is definitely favorable to a good balance state of the hole- and electron-transports in OLEDs and then the desirable device performance, especially when they are used as the undoped active layer in devices.
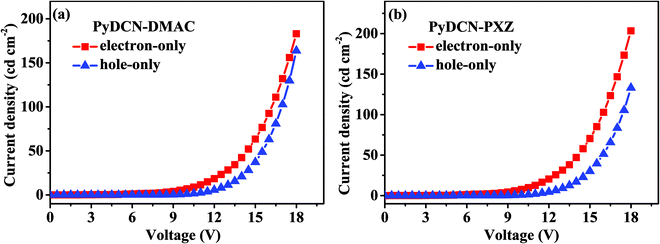 |
| Fig. 5 The current density versus voltage curves of the hole-only (a) and electron-only (b) devices for PyDCN–DMAC and PyDCN–PXZ. | |
Electroluminescent devices
Based on the excellent TADF properties of PyDCN–PXZ and PyDCN–DMAC with a relatively high kFs in the order of 107 s−1 and kRISCs in the order of 105 s−1, they were used as emitters to fabricate OLEDs. PyDCN–PXZ was first used either as a doped or non-doped emitter to fabricate green and yellow emitting devices with the configuration of ITO/PEDOT:PSS (40 nm)/TAPC (20 nm)/emission layer (EML) (20 nm)/TmPyPB (40 nm)/LiF (1 nm)/Al (200 nm). In the doped device G1, PyDCN–PXZ was doped in the CBP host at an optimized concentration of 15 wt% as the EML. At the same time, the neat film of PyDCN–PXZ was used as the EML to fabricate the non-doped device G2 based on the high and balanced charge transports of this compound (Fig. 5). TAPC was used as the hole-transporting layer (HTL) and TmPyPB as electron-transporting layer (ETL), PEDOT:PSS and LiF as hole- and electron-injecting materials. The devices structures are shown in Fig. 6. The molecular structures of the related materials and energy-level diagrams of these devices are shown in Fig. S8 (ESI†). The current density–voltage–brightness (J–V–B) characteristics and efficiency curves and the EL spectra of the green device G1 and the yellow non-doped device G2 are depicted in Fig. 7 and Fig. S9 (ESI†), and the EL data of these devices are summarized in Table 2. The doped device G1 of PyDCN–PXZ with a doping concentration of 15 wt% realized green EL at 519 nm with CIE coordinates of (0.30, 0.53) and high performance with a maximum luminance (Lmax) of 22 534 cd m−2, a maximum external quantum efficiency (EQE, ηext) of 26.9%, a maximum current efficiency (ηC) of 81.9 cd A−1 and a maximum power efficiency (ηP) of 79.6 lm W−1. At a high brightness of 1000 cd m−2, ηext, ηC and ηP still remained as high as 20.1%, 62.4 cd A−1 and 36.8 lm W−1, respectively. While the non-doped device G2 of PyDCN–PXZ exhibited yellow EL with CIE coordinates of (0.43, 0.53) and a peak wavelength at 557 nm, which is red shifted in comparison with the doped device G1 by 38 nm. It is also interesting that device G2 exhibited a much higher current density and higher brightness than the doped device G1 at a given voltage, as shown in Fig. 7a. This should be because PyDCN–PXZ is bipolar charge transporting due to the presence of both n- and p-type units in the molecule, which has much better charge conductivity than the CBP host that is predominantly hole-transporting due to the presence of p-type carbazole groups. The non-doped device G2 also exhibited excellent performance with a low turn on voltage (Von, the voltage to deliver a brightness of 1 cd m−2) of 2.6 V, a Lmax of 48 114 cd m−2, a maximum ηext of 18.2%, a maximum ηC of 51.6 cd A−1 and a maximum ηP of 53.3 lm W−1. Although the current density and brightness of the non-doped device G2 were tremendously improved in comparison with the doped device G1, the efficiencies of device G2 are slightly reduced. The reduced efficiencies in G2 can be ascribed to the concentration quenching in the neat films and the possible leak current that does not contribute to effective charge recombination.43 However, with increasing the current density, the efficiencies of the non-doped device G2 decayed more slowly than those of the doped device G1. For example, at an extremely high brightness of 8000 cd m−2, the ηext of G2 remained at 11.2%, which is already slightly higher than that of device G1 (10.7%). The extra efficient stability in the high current density region for the non-doped device G2 should benefit from the more balanced positive and negative charge transportations in the neat film of the bipolar PyDCN–PXZ than CBP in device G1.
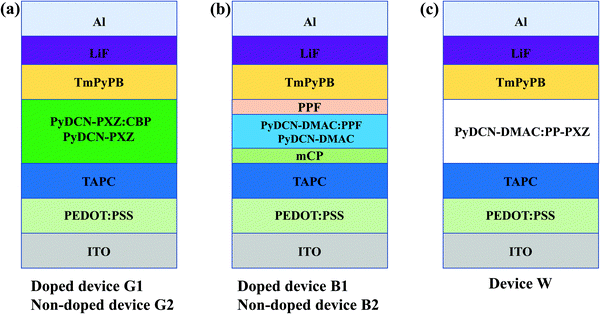 |
| Fig. 6 Structures of the single emitting color TADF-OLEDs and WOLED. | |
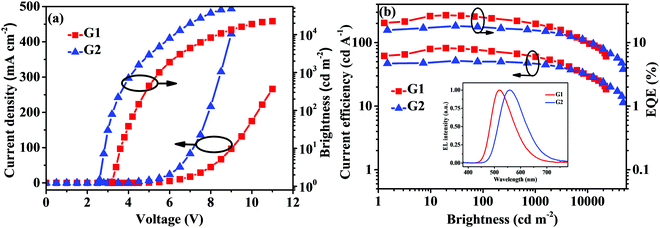 |
| Fig. 7 The J–V–B characteristics (a) and efficiency curves (b) for doped device G1 and non-doped device G2 based on PyDCN–PXZ. | |
Table 2 Electroluminescence characteristics of the single emitting color and white OLEDsa
Devices |
EML |
V
on (V) |
L
max (cd m−2) |
η
c
(cd A−1) |
η
p
(lm W−1) |
η
ext
(%) |
λ
EL (nm) |
CIEd (x, y) |
CRI |
Abbreviations: Von, turn-on voltage. Lmax, maximum luminance. ηext, external quantum efficiency. ηc, current efficiency. ηp, power efficiency. CIE (x, y), Commission International de I’Eclairage coordinates.
Order of measured values: maximum and at 1000 cd m−2.
Maximum values.
Measured at 7 V.
|
G1 |
15 wt% PyDCN–PXZ:CBP |
3.2 |
22534 |
81.9/62.4 |
79.6 |
26.9/20.1 |
519 |
0.30,0.53 |
— |
G2 |
PyDCN–PXZ |
2.6 |
48114 |
51.6/46.6 |
53.3 |
18.2/14.3 |
557 |
0.43,0.53 |
— |
B1 |
10 wt% PyDCN–DMAC:PPF |
2.9 |
8651 |
45.6/27.5 |
51.5 |
25.6/15.2 |
472 |
0.17,0.26 |
— |
B2 |
PyDCN–DMAC |
2.8 |
10066 |
16.8/10.5 |
13.2 |
6.8/4.2 |
494 |
0.22,0.41 |
— |
W |
0.3 wt% PP–PXZ:PyDCN–DMAC |
2.6 |
9000 |
44.8/30.4 |
49.0 |
18.5/12.6 |
472/581 |
0.38,0.44 |
73 |
In a similar way, PyDCN–DMAC was also used as a doped and non-doped emitter to fabricate OLEDs with the device structure of ITO/PEDOT:PSS (40 nm)/TAPC (20 nm)/mCP (10 nm)/emitting layer (EML) (20 nm)/PPF (10 nm)/TmPyPB (40 nm)/LiF (1 nm)/Al (200 nm), where the EML is a co-deposited layer of PyDCN–DMAC (10 wt%):PPF (device B1, Fig. 6b) or non-doped PyDCN–DMAC (device B2, Fig. 6b). The high-triplet-energy (3.1 eV) PPF was used as the host for PyDCN–DMAC in the doped device B1 to guarantee forward energy transfer from host to dopant. At the same time, 9,9′-(1,3-phenylene)bis-9H-carbazole (mCP) and PPF serve as the exciton-blocking layers to confine triplet excitons within the EML. The EL spectra, current density–voltage–brightness (J–V–B) characteristics and efficiency curves for these devices are shown in Fig. 8 and Fig. S9 (ESI†), and the EL data are summarized in Table 2. The doped device B1 with an optimized doping concentration of 10 wt% in the PPF host turned on at a low voltage (Von) of 2.9 V, exhibited sky-blue light emission with an EL peak at 472 nm and CIE coordinates of (0.17, 0.26). Apparently the emitting color of the PyDCN–DMAC based device B1 more approaches to real blue in comparison to that of the triazine based analogue DMAC–TRZ, which gave the sky-blue emitting color with CIE coordinates of (0.22, 0.46).39 The doped device B1 of PyDCN–DMAC achieved a good performance with a maximum ηext of 25.6%, ηC of 45.6 cd A−1 and ηP of 51.5 lm W−1. However, the performance of the non-doped device B2 of PyDCN–DMAC is rather inferior with a maximum ηext of 6.8%, ηC of 16.8 cd A−1 and ηP of 13.2 lm W−1 and a peak EL at 490 nm. Obviously, the possible concentration quenching and/or triplet–triplet annihilation in the non-doped device of PyDCN–DMAC are so strong that the EL efficiency was not as satisfactory as expected.
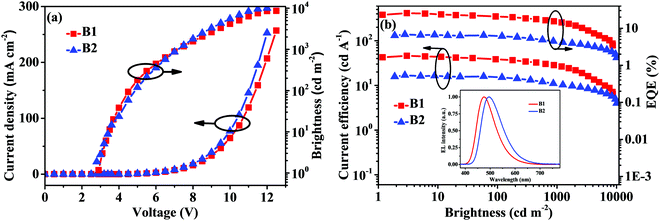 |
| Fig. 8 The J–V–B characteristics (a) and efficiency curves (b) for the doped (B1) and non-doped (B2) devices of PyDCN–DMAC. | |
Considering the sky-blue emission of PyDCN–DMAC and appropriate HOMO and LUMO energy levels and balanced charge transportations, we were motivated to fabricate a bi-component single-EML all-TADF WOLED utilizing PyDCN–DMAC as the blue host emitter. The TADF emitter (PP–PXZ) reported by our group was selected as the orange component (chemical structure shown in Fig. S8, ESI†).44 The simplified single-EML all-TADF WOLED (W) has the structure of ITO/PEDOT:PSS (45 nm)/TAPC (20 nm)/PyDCN–DMAC:PP–PXZ (0.3 wt%, 20 nm)/TmPyPB (40 nm)/LiF (1 nm)/Al (200 nm), as shown in Fig. 6c. The J–V–B characteristics, efficiency curves, and EL spectra of the white device are shown in Fig. 9, and the key performance parameters of device W are summarized in Table 2. When the doping concentration of PP–PXZ was tuned at 0.3 wt% in PyDCN–DMAC, a warm white light emission was obtained, as shown by the EL spectra in Fig. 9c, which includes a blue component centered at 472 nm from PyDCN–DMAC and an orange emission at 581 nm from PP–PXZ. The PL spectrum of the PyDCN–DMAC:PP–PXZ film with same doping concentration as that in the emitting layer of white OLED (Fig. S10 in ESI†) has the same profile with the EL spectra, with a blue component at 472 nm and an orange one centered at 581 nm, confirming the roles of PyDCN–DMAC as the blue host emitter and PP–PXZ as the orange emitting dopant. Apparently, the coexistence of blue and orange emission and the generation of white light were realized by in-complete forward energy transfer from the singlet/triplet excitons of sky-blue host to orange dopant due to the low doping concentrations of 0.3 wt%. It is exciting that the EL spectra of device W are rather stable within the investigated voltage range. For example, device W exhibited CIE coordinates of (0.39, 0.44) with a color rendering index (CRI) of 69 at 6 V, while only a small change was detected at 10 V with CIE coordinates of (0.38, 0.44) and a CRI of 73, as illustrated in Fig. S11 (ESI†). In comparison with the poor color stability that was frequently observed in hybrid WOLEDs and mainly caused by blue emission intensity enhancement under high current density, the extraordinarily stable white light color of the present all-TADF WOLED is really a precious merit. The bipolar and balanced charge transporting feature of PyDCN–DMAC (Fig. 5b) and thus the stable and wide recombination zone in the emitting layer of device W should be responsible, at least partially, for the color stability.
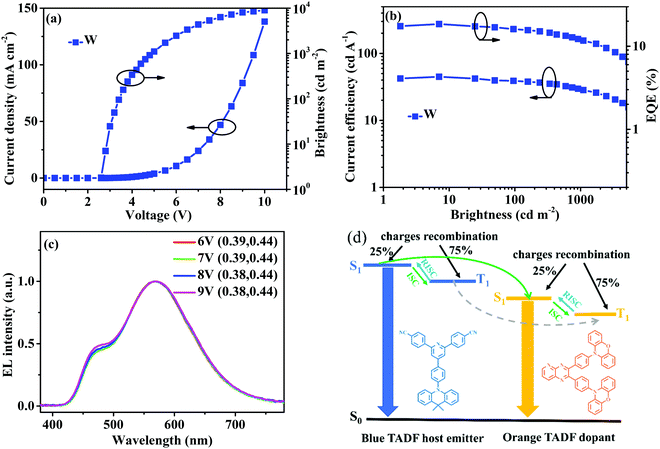 |
| Fig. 9 The J–V–B characteristics (a), efficiency curves (b), EL spectra (c), and supposed energy transfer diagram (d) of the PyDCN–DMAC:PP–PXZ based full-TADF WOLED. | |
The excited state conversion and light emission mechanism in this all-TADF W is shown in Fig. 9d. Obviously, both singlet and triplet excitons are mainly generated on the host molecules through charge recombination in this all-TADF WOLED. In the emitting layer, with very low doping levels, the triplet excitons of PyDCN–DMAC will mainly up-convert into its singlet excitons via reverse intersystem crossing owing to the small ΔEST. And then a part of the singlet excitons (including those directly formed by charge recombination and those up-converted from triplets) will emit blue prompt fluorescence and TADF, and most of the rest will transfer energy through long-range Förster mechanism onto the PP–PXZ molecules to form their singlet excitons, which then emit orange fluorescence to compose white light. The Dexter energy transfer from PyDCN–DMAC triplets to PP–PXZ can be neglected in device W because the rapid and efficient RISC must reduce the triplet exciton density on the PyDCN–DMAC host and Dexter energy transfer is a short-range process and usually requires high dopant concentrations. Furthermore, the doped orange TADF PP–PXZ can be excited by charge recombination as well to form both singlet and triplet excitons. The triplet excitons also easily up-convert into its singlets through RISC owing to the small ΔEST. Finally, both PyDCN–DMAC and PP–PXZ molecules emit fluorescence or delayed fluorescence to form white light.
Device W exhibited good performance with a maximum brightness (Lmax) of 9000 cd m−2, a maximum ηext of 18.5%, a peak ηc of 44.8 cd A−1 and a maximum ηp of 49.0 lm W−1. The quantum efficiency of the present W (18.5%) is comparable to the efficiencies of many reported all-TADF WOLEDs, as shown in Table S2 (ESI†),45–49 although there is still space to optimize and improve the efficiency. The efficiencies of device W are relatively stable with slow efficiency roll-off. At a practical brightness of 100 cd m−2 and a high brightness of 1000 cd m−2, the ηext of device W remained at 16.0% and 12.6%, respectively. It is obvious that the good performance of device W should benefit from efficient energy transfer from PyDCN–DMAC to PP–PXZ. In addition, the excellent bipolar charge transport property of PyDCN–DMAC can reduce the interface charge accumulation and facilitate charge injection and transport.
Conclusions
In conclusion, 4,4′-(4-phenylpyridine-2,6-diyl)dibenzonitrile (PyDCN) was designed and developed as an acceptor for TADF emitters with emphasis on decentralizing the electron-withdrawing functional groups and reducing the electron-withdrawing strength in comparison with triazine. Two novel TADF emitters PyDCN–DMAC and PyDCN–PXZ were developed with DMAC and PXZ as the donors, which exhibited a relatively high radiative transition rate constant (kFs) in the order of 107 s−1 and a reverse intersystem crossing rate constant (kRISCs) in the order of 105 s−1 based on the appropriate structure design strategy. The PL and EL of PyDCN–DMAC have peak wavelengths of 480 and 472 nm, which are blue shifted by at least 10 nm relative to most of the DMAC based sky-blue materials. PyDCN–PXZ exhibited green and yellow emission in doped and non-doped TADF-OLEDs, with maximum EQEs of 26.9% and 18.2% and slow efficiency roll-off. PyDCN–DMAC realized an excellent EQE of 25.6% in its doped OLED. Furthermore, single-EML all-TADF warm white OLED was fabricated using PyDCN–DMAC as the blue host emitter in combination with an orange TADF dopant PP–PXZ, giving an impressive peak EQE of 18.5% and a CRI of 73. It has been demonstrated that PyDCN is an excellent acceptor for constructing high-performance TADF emitters, especially for blue TADF emitters, and the structure design strategy in this study should be valid for the development of large amount of acceptor and TADF materials.
Conflicts of interest
There are no conflicts of interest to declare.
Acknowledgements
We thank the National Natural Science Foundation of China (22078051 and U1801258), the Fundamental Research Funds for the Central Universities (DUT19TD28), and the Open Fund of the State Key Laboratory of Luminescent Materials and Devices (South China University of Technology, 2021-skllmd-03) for financial support.
References
- S. Reineke, F. Lindner, G. Schwartz, N. Seidler, K. Walzer, B. Lussem and K. Leo, White organic light-emitting diodes with fluorescent tube efficiency, Nature, 2009, 459, 234–238 CrossRef CAS PubMed.
- G. Schwartz, M. Pfeiffer, S. Reineke, K. Walzer and K. Leo, Harvesting Triplet Excitons from Fluorescent Blue Emitters in White Organic Light-Emitting Diodes, Adv. Mater., 2007, 19, 3672–3676 CrossRef CAS.
- C. J. Zheng, J. Wang, J. Ye, M. F. Lo, X. K. Liu, M. K. Fung, X. H. Zhang and C. S. Lee, Novel efficient blue fluorophors with small singlet-triplet splitting: hosts for highly efficient fluorescence and phosphorescence hybrid WOLEDs with simplified structure, Adv. Mater., 2013, 25, 2205–2211 CrossRef CAS.
- N. Sun, Q. Wang, Y. Zhao, Y. Chen, D. Yang, F. Zhao, J. Chen and D. Ma, High-performance hybrid white organic light-emitting devices without interlayer between fluorescent and phosphorescent emissive regions, Adv. Mater., 2014, 26, 1617–1621 CrossRef CAS.
- X. L. Li, G. Xie, M. Liu, D. Chen, X. Cai, J. Peng, Y. Cao and S. J. Su, High-Efficiency WOLEDs with High Color-Rendering Index based on a Chromaticity-Adjustable Yellow Thermally Activated Delayed Fluorescence Emitter, Adv. Mater., 2016, 28, 4614–4619 CrossRef CAS.
- B. Liu, H. Nie, X. Zhou, S. Hu, D. Luo, D. Gao, J. Zou, M. Xu, L. Wang, Z. Zhao, A. Qin, J. Peng, H. Ning, Y. Cao and B. Z. Tang, Manipulation of Charge and Exciton Distribution Based on Blue Aggregation-Induced Emission Fluorophors: A Novel Concept to Achieve High-Performance Hybrid White Organic Light-Emitting Diodes, Adv. Funct. Mater., 2016, 26, 776–783 CrossRef CAS.
- X.-K. Liu, C.-J. Zheng, M.-F. Lo, J. Xiao, Z. Chen, C.-L. Liu, C.-S. Lee, M.-K. Fung and X.-H. Zhang, Novel Blue Fluorophor with High Triplet Energy Level for High Performance Single-Emitting-Layer Fluorescence and Phosphorescence Hybrid White Organic Light-Emitting Diodes, Chem. Mater., 2013, 25, 4454–4459 CrossRef CAS.
- C. Cao, W. C. Chen, J. X. Chen, L. Yang, X. Z. Wang, H. Yang, B. Huang, Z. L. Zhu, Q. X. Tong and C. S. Lee, Bipolar Blue Host Emitter with Unity Quantum Yield Allows Full Exciton Radiation in Single-Emissive-Layer Hybrid White Organic Light-Emitting Diodes, ACS Appl. Mater. Interfaces, 2019, 11, 11691–11698 CrossRef CAS.
- P. Wei, D. Zhang and L. Duan, Modulation of Förster and Dexter Interactions in Single-Emissive-Layer All-Fluorescent WOLEDs for Improved Efficiency and Extended Lifetime, Adv. Funct. Mater., 2020, 30, 1907083 CrossRef CAS.
- P. Heimel, A. Mondal, F. May, W. Kowalsky, C. Lennartz, D. Andrienko and R. Lovrincic, Unicolored phosphor-sensitized fluorescence for efficient and stable blue OLEDs, Nat. Commun., 2018, 9, 4990 CrossRef.
- S. Ying, P. Pang, S. Zhang, Q. Sun, Y. Dai, X. Qiao, D. Yang, J. Chen and D. Ma, Superior Efficiency and Low-Efficiency Roll-Off White Organic Light-Emitting Diodes Based on Multiple Exciplexes as Hosts Matched to Phosphor Emitters, ACS Appl. Mater. Interfaces, 2019, 11, 31078–31086 CrossRef CAS.
- K. H. Kim, S. Lee, C. K. Moon, S. Y. Kim, Y. S. Park, J. H. Lee, J. Woo Lee, J. Huh, Y. You and J. J. Kim, Phosphorescent dye-based supramolecules for high-efficiency organic light-emitting diodes, Nat. Commun., 2014, 5, 4769 CrossRef CAS.
- K. Li, G. Cheng, C. Ma, X. Guan, W.-M. Kwok, Y. Chen, W. Lu and C.-M. Che, Light-emitting platinum(ii) complexes supported by tetradentate dianionic bis(N-heterocyclic carbene) ligands: towards robust blue electrophosphors, Chem. Sci., 2013, 4, 2630 RSC.
- G. Schwartz, S. Reineke, T. C. Rosenow, K. Walzer and K. Leo, Triplet Harvesting in Hybrid White Organic Light-Emitting Diodes, Adv. Funct. Mater., 2009, 19, 1319–1333 CrossRef CAS.
- Q. Wang, Y. X. Zhang, Y. Yuan, Y. Hu, Q. S. Tian, Z. Q. Jiang and L. S. Liao, Alleviating Efficiency Roll-Off of Hybrid Single-Emitting Layer WOLED Utilizing Bipolar TADF Material as Host and Emitter, ACS Appl. Mater. Interfaces, 2019, 11, 2197–2204 CrossRef CAS.
- D. Zhang, M. Cai, Y. Zhang, D. Zhang and L. Duan, Highly Efficient Simplified Single-Emitting-Layer Hybrid WOLEDs with Low Roll-off and Good Color Stability through Enhanced Förster Energy Transfer, ACS Appl. Mater. Interfaces, 2015, 7, 28693–28700 CrossRef CAS.
- D. Liu, D. Li, H. Meng, Y. Wang and L. Wu, Multifunctional applications of triazine/carbazole hybrid thermally activated delayed fluorescence emitters in organic light emitting diodes, J. Mater. Chem. C, 2019, 7, 12470–12481 RSC.
- J. Sun, J. Zhang, Q. Liang, Y. Wei, C. Duan, C. Han and H. Xu, Charge-Transfer Exciton Manipulation Based on Hydrogen Bond for Efficient White Thermally Activated Delayed Fluorescence, Adv. Funct. Mater., 2019, 30, 1908568 CrossRef.
- N. Sun, Q. Wang, Y. Zhao, D. Yang, F. Zhao, J. Chen and D. Ma, A hybrid white organic light-emitting diode with above 20% external quantum efficiency and extremely low efficiency roll-off, J. Mater. Chem. C, 2014, 2, 7494–7504 RSC.
- Y. Chi and P. T. Chou, Transition-metal phosphors with cyclometalating ligands: fundamentals and applications, Chem. Soc. Rev., 2010, 39, 638–655 RSC.
- X. Tang, Y. Li, Y. K. Qu, C. C. Peng, A. Khan, Z. Q. Jiang and L. S. Liao, All-Fluorescence White Organic Light-Emitting Diodes Exceeding 20% EQEs by Rational Manipulation of Singlet and Triplet Excitons, Adv. Funct. Mater., 2020, 30, 1910633 CrossRef CAS.
- B. Liu, X.-L. Li, H. Tao, J. Zou, M. Xu, L. Wang, J. Peng and Y. Cao, Manipulation of exciton distribution for high-performance fluorescent/phosphorescent hybrid white organic light-emitting diodes, J. Mater. Chem. C, 2017, 5, 7668–7683 RSC.
- F. M. Xie, S. J. Zou, Y. Li, L. Y. Lu, R. Yang, X. Y. Zeng, G. H. Zhang, J. Chen and J. X. Tang, Management of Delayed Fluorophor-Sensitized Exciton Harvesting for Stable and Efficient All-Fluorescent White Organic Light-Emitting Diodes, ACS Appl. Mater. Interfaces, 2020, 12, 16736–16742 CrossRef CAS.
- H. Uoyama, K. Goushi, K. Shizu, H. Nomura and C. Adachi, Highly efficient organic light-emitting diodes from delayed fluorescence, Nature, 2012, 492, 234–238 CrossRef CAS.
- Q. Zhang, J. Li, K. Shizu, S. Huang, S. Hirata, H. Miyazaki and C. Adachi, Design of efficient thermally activated delayed fluorescence materials for pure blue organic light emitting diodes, J. Am. Chem. Soc., 2012, 134, 14706–14709 CrossRef CAS.
- Q. Zhang, H. Kuwabara, W. J. Potscavage, Jr., S. Huang, Y. Hatae, T. Shibata and C. Adachi, Anthraquinone-based intramolecular charge-transfer compounds: computational molecular design, thermally activated delayed fluorescence, and highly efficient red electroluminescence, J. Am. Chem. Soc., 2014, 136, 18070–18081 CrossRef CAS.
- K. Goushi, K. Yoshida, K. Sato and C. Adachi, Organic light-emitting diodes employing efficient reverse intersystem crossing for triplet-to-singlet state conversion, Nat. Photonics, 2012, 6, 253–258 CrossRef CAS.
- S. Hirata, Y. Sakai, K. Masui, H. Tanaka, S. Y. Lee, H. Nomura, N. Nakamura, M. Yasumatsu, H. Nakanotani, Q. Zhang, K. Shizu, H. Miyazaki and C. Adachi, Highly efficient blue electroluminescence based on thermally activated delayed fluorescence, Nat. Mater., 2015, 14, 330–336 CrossRef CAS PubMed.
- T. Nishimoto, T. Yasuda, S. Y. Lee, R. Kondo and C. Adachi, A six-carbazole-decorated cyclophosphazene as a host with high triplet energy to realize efficient delayed-fluorescence OLEDs, Mater. Horiz., 2014, 1, 264–269 RSC.
- J. Guo, Z. Zhao and B. Z. Tang, Purely Organic Materials with Aggregation-Induced Delayed Fluorescence for Efficient Nondoped OLEDs, Adv. Opt. Mater., 2018, 6, 1800264 CrossRef.
- D. H. Ahn, H. Lee, S. W. Kim, D. Karthik, J. Lee, H. Jeong, J. Y. Lee and J. H. Kwon, Highly Twisted Donor-Acceptor Boron Emitter and High Triplet Host Material for Highly Efficient Blue Thermally Activated Delayed Fluorescent Device, ACS Appl. Mater. Interfaces, 2019, 11, 14909–14916 CrossRef CAS PubMed.
- Y. Yuan, X. Tang, X. Y. Du, Y. Hu, Y. J. Yu, Z. Q. Jiang, L. S. Liao and S. T. Lee, The Design of Fused Amine/Carbonyl System for Efficient Thermally Activated Delayed Fluorescence: Novel Multiple Resonance Core and Electron Acceptor, Adv. Opt. Mater., 2019, 7, 1801536 CrossRef.
- I. S. Park, H. Komiyama and T. Yasuda, Pyrimidine-based twisted donor-acceptor delayed fluorescence molecules: a new universal platform for highly efficient blue electroluminescence, Chem. Sci., 2017, 8, 953–960 RSC.
- J.-i. Nishide, H. Nakanotani, Y. Hiraga and C. Adachi, High-efficiency white organic light-emitting diodes using thermally activated delayed fluorescence, Appl. Phys. Lett., 2014, 104, 233304 CrossRef.
- Y. Chen, S. Li, T. Hu, X. Wei, Z. Li, Y. Liu, J. Liu, R. Wang, Y. Yi, C. Zhao, Y. Wang and P. Wang, Highly efficient white light-emitting diodes with a bi-component emitting layer based on blue and yellow thermally activated delayed fluorescence emitters, J. Mater. Chem. C, 2018, 6, 2951–2956 RSC.
- H. Chen, H. Liu, P. Shen, J. Zeng, R. Jiang, Y. Fu, Z. Zhao and B. Z. Tang, Efficient Sky-Blue Bipolar Delayed Fluorescence Luminogen for High-Performance Single Emissive Layer WOLEDs, Adv. Opt. Mater., 2021, 9, 2002019 CrossRef CAS.
- D. Ding, Z. Wang, C. Li, J. Zhang, C. Duan, Y. Wei and H. Xu, Highly Efficient and Color-Stable Thermally Activated Delayed Fluorescence White Light-Emitting Diodes Featured with Single-Doped Single Emissive Layers, Adv. Mater., 2020, 32, 1906950 CrossRef CAS.
- W. L. Tsai, M. H. Huang, W. K. Lee, Y. J. Hsu, K. C. Pan, Y. H. Huang, H. C. Ting, M. Sarma, Y. Y. Ho, H. C. Hu, C. C. Chen, M. T. Lee, K. T. Wong and C. C. Wu, A versatile thermally activated delayed fluorescence emitter for both highly efficient doped and non-doped organic light emitting devices, Chem. Commun., 2015, 51, 13662–13665 RSC.
- W. Li, M. Li, W. Li, Z. Xu, L. Gan, K. Liu, N. Zheng, C. Ning, D. Chen, Y. C. Wu and S. J. Su, Spiral Donor Design Strategy for Blue Thermally Activated Delayed Fluorescence Emitters, ACS Appl. Mater. Interfaces, 2021, 13, 5302–5311 CrossRef CAS PubMed.
- L. Wang, X. Cai, B. Li, M. Li, Z. Wang, L. Gan, Z. Qiao, W. Xie, Q. Liang, N. Zheng, K. Liu and S. J. Su, Achieving Enhanced Thermally Activated Delayed Fluorescence Rates and Shortened Exciton Lifetimes by Constructing Intramolecular Hydrogen Bonding Channels, ACS Appl. Mater. Interfaces, 2019, 11, 45999–46007 CrossRef CAS.
- W. Li, J. Li, D. Liu and Q. Jin, Simple Bipolar Host Materials for High-Efficiency Blue, Green, and White Phosphorescence OLEDs, ACS Appl. Mater. Interfaces, 2016, 8, 22382–22391 CrossRef CAS.
- F. Wang, D. Liu, J. Li and M. Ma, Modulation of n-Type Units in Bipolar Host Materials toward High-Performance Phosphorescent OLEDs, ACS Appl. Mater. Interfaces, 2017, 9, 37888–37897 CrossRef CAS.
- D. Li, D. Liu, J. Li, R. Dong, B. Liu and Q. Wei, Low-driving-voltage sky-blue phosphorescent organic light-emitting diodes with bicarbazole-bipyridine bipolar host materials, Mater. Chem. Front., 2021, 5, 2867–2876 RSC.
- T. Huang, D. Liu, J. Jiang and W. Jiang, Quinoxaline and Pyrido[x,y-b]pyrazine-Based Emitters: Tuning Normal Fluorescence to Thermally Activated Delayed Fluorescence and Emitting Color over the Entire Visible-Light Range, Chem. – Eur. J., 2019, 25, 10926–10937 CrossRef CAS.
- T. Huang, D. Liu, D. Li, W. Jiang and J. Jiang, Novel yellow thermally activated delayed fluorescence emitters for highly efficient full-TADF WOLEDs with low driving voltages and remarkable color stability, New J. Chem., 2019, 43, 13339–13348 RSC.
- W. Li, B. Li, X. Cai, L. Gan, Z. Xu, W. Li, K. Liu, D. Chen and S. J. Su, Tri-Spiral Donor for High Efficiency and Versatile Blue Thermally Activated Delayed Fluorescence Materials, Angew. Chem., Int. Ed., 2019, 58, 11301–11305 CrossRef CAS.
- W. Luo, T.-T. Wang, X. Chen, K.-N. Tong, W. He, S.-Q. Sun, Y.-J. Zhang, L.-S. Liao and M.-K. Fung, High-performance organic light-emitting diodes with natural white emission based on thermally activated delayed fluorescence emitters, J. Mater. Chem. C, 2020, 8, 10431–10437 RSC.
- C. Zhang, Y. Lu, Z. Liu, Y. Zhang, X. Wang, D. Zhang and L. Duan, A pi-D and pi-A Exciplex-Forming Host for High-Efficiency and Long-Lifetime Single-Emissive-Layer Fluorescent White Organic Light-Emitting Diodes, Adv. Mater., 2020, 32, 2004040 CrossRef CAS.
- C. Zhang, D. Zhang, Z. Bin, Z. Liu, Y. Zhang, H. Lee, J. H. Kwon and L. Duan, Color-Tunable All-Fluorescent White Organic Light-Emitting Diodes with a High External Quantum Efficiency Over 30% and Extended Device Lifetime, Adv. Mater., 2021, 2103102 CrossRef PubMed.
Footnote |
† Electronic supplementary information (ESI) available: Appendix A. See DOI: 10.1039/d1qm01303c |
|
This journal is © the Partner Organisations 2022 |