DOI:
10.1039/D1NJ05105A
(Paper)
New J. Chem., 2022,
46, 370-384
A veratraldehyde-appended organosilicon probe and its hybrid silica nanoparticles as a dual chemosensor for colorimetric and fluorimetric detection of Cu2+ and Fe3+ ions†
Received
26th October 2021
, Accepted 14th November 2021
First published on 15th November 2021
Abstract
This article highlights the design and synthesis of the Schiff bases of veratraldehyde-based organosilatranes and organosilocanes (3a–3c), which were meticulously characterized with the aid of nuclear magnetic resonance (NMR, 1H and 13C) and mass spectroscopy techniques. Moreover, compound 3a was fabricated over silica nanoparticles by a one-pot method, which was authenticated by infrared (IR) spectroscopy, X-ray diffractometry (XRD), energy dispersive X-ray spectroscopy (EDX), thermogravimetric analysis (TGA), and field emission scanning electron microscopy (FE-SEM) analysis. The colorimetric and fluorimetric detection of 3a and its hybrid silica nanoparticles (V-NPs) revealed their significant sensing ability towards Cu2+ and Fe3+ ions over other relevant competitive metal ions. Interestingly, the binding of sensor 3a and the V-NPs with Cu2+ and Fe3+ ions manifested an instant color change from yellow to light green and colorless, respectively, in daylight, which is detectable by the naked-eye, and a fluorescence turn-off response under UV light. The results demonstrate that the organic–inorganic nanohybrids exhibited better sensitivity, greater affinity and lower limit of detection leading to better response time than the parent 3a towards Cu2+ and Fe3+ ions in the optimum physiological pH range. A computational analysis using the DFT approach was performed to gain insight into the complexation mode of 3a with Cu2+ and Fe3+ ions. In addition to this, anti-oxidant properties were also investigated for compounds 3a–3c. Altogether, these findings pave the way for the design and synthesis of dual metal ion sensors.
1. Introduction
Prolonged efforts have been devoted to the design of innovative chemosensors with high selectivity and remarkable sensitivity due to their indispensable role in detecting transition and heavy metal ions efficiently. Among many bioactive metal ions, Cu2+ and Fe3+ ions play a significant role in a variety of biological and environmental processes, but they become a serious threat to all life forms beyond an optimum limit, leading to debilitating diseases, and thus they act as a double-edged sword.1 Moreover, copper is an essential nutrient and induces the activation of dioxygen, which is necessary to all living organisms. It assists in the maintenance of brain tissues, cellular respiration and the formation of heme in the blood, and acts as a catalytic cofactor in metalloenzymes.2 An aberrant amount of copper ions can trigger severe health abnormalities, such as neurodegenerative diseases3 like Alzheimer's disease,4 teratogenicity, myelodysplasia, Parkinson's disease, anaemia, chromosomal aberrations,5 leukopenia (low white blood cell count), neutropenia,6 Menkes syndrome and Wilson's disease.7 Furthermore, Fe3+ ions serve as an important intracellular element and play a crucial role in oxygen uptake and transportation, electron transfer, oxygen metabolism and enzyme catalysis.8 Biological disorders like insomnia, anaemia, decreased immunity and low blood pressure are caused due to a deficiency of oxygen, whereas its superfluous accumulation in humans may result in damaging biomolecules by generating reactive oxygen species (ROS), which can cause damage to proteins, lipids and nucleic acids.9 It is also a thing of serious concern for persons suffering from hemochromatosis, Parkinson's disease10 and Alzheimer's disease.
The instrument-based detection methods for Cu2+ and Fe3+ ions, like inductively coupled plasma mass spectrometry,11 chromatography, neutron activation analysis,12 atomic absorption spectroscopy,13etc., are limited as these are uneconomical, time-consuming and susceptible to difficulties during functioning, which restricts their application in sensing metal ions. Therefore, the designing of simple, yet selective sensor molecules with marvelous optical responses (colorimetric and fluorimetric) towards numerous analytes is imminently required to develop a more proficient sensing system. In fact, it is a quite challenging task for a single chemosensor to detect various analytes,14 but the eminent advantages of photophysical detection have stimulated the designing of chemosensors for Cu2+/Fe3+ ions. Moreover, Schiff base-appended “silatrane units” have gained appreciable attention due to their enhanced biological properties and promising optical response. This is an important research endeavour as nitrogen atoms on the azomethine linkage have a lone pair of electrons for efficient coordination with metal cations.15 As a result, numerous organic molecules have been used to design Schiff bases with a silatranyl motif, and in our present work veratraldehyde is the most promising moiety. It finds applications in agrochemicals, food aromatizers and the preparation of certain drugs,16 and has various pharmacological activities, like anti-fungal, anti-stress and anti-microbial activities.17 It is also used as a primary component in many perfume compounds for the synthesis of fragrance substances.18 Furthermore, silicon-capped nanomaterials have opened a new avenue in the medical field resulting in increased therapeutic drug efficiency.19 They are also drawing attention from every branch of research due to their characteristic optical response.20
In light of the aforementioned facts, we have synthesized veratraldehyde-based organosilanes, as well as their silatranes and silocanes. Moreover, one of the derivatized silatrane modules was embedded over a silica nanosurface to extend our research in the field of nanotechnology. The silica nanoparticles were characterized by X-ray diffraction (XRD), energy dispersive X-ray spectroscopy (EDX) and field emission scanning electron microscopy (FE-SEM). The synthesized compounds were initially screened for their antioxidant activity and then explored for their photophysical properties towards various metal ions.
2. Experimental section
2.1. Materials and methods
Veratraldehyde (Sigma Aldrich), [3-(2-aminoethylamino)propyl] trimethoxysilane (Aldrich), (diethoxy(methyl)silyl) propan-1-amine (Avra), triethanolamine (Merck), triisopropanolamine (Merck), diethanolamine (Merck) and potassium hydroxide (Avra) were directly utilized as received. The organic solvents were commercially bought from Merck and dried before use as per standard procedures. Inorganic chloride salts of metals such as aluminium, cobalt, copper, ferric, nickel, mercury, lead, calcium, stannous, manganese, caesium, cadmium and zinc were purchased from S.D. Fine Chem. Ltd, India. Using sealed capillaries, melting points were measured in a Mel Temp II device. A Thermo-Fisher Scientific NICOLET IS50 spectrophotometer was used to record the Fourier-transform infrared (FT-IR) spectra. The multinuclear 1H and 13C nuclear magnetic resonance (NMR) spectra were recorded on a BRUKER AVANCE II (400 MHz) and a JEOL (AL 300 MHz) spectrophotometer in CDCl3 and chemical shifts (δ) were reported relative to tetramethylsilane. Mass spectrometry analysis was performed using a XEVO-G2-XS QTOF mass spectrometer. Thermogravimetric analysis (TGA) was achieved using a SDT Q 600 V20.9 Build 20 TGA instrument. A JASCO V-530 UV-vis spectrophotometer and a Hitachi F-7000 fluorescence spectrophotometer (5 J1-004) were used to carry out electronic spectral measurements. X-ray diffractometry (XRD) was executed using a PANalytical X'pert PRO spectrophotometer equipped with a Cu-Ka radiation source. A JEOL JSM-6610LV spectrometer was used to perform energy dispersive X-ray (EDX) analysis at a voltage of 15 kV. Scanning electron microscopy (SEM) observations were conducted on a HITACHI SU8010 microscope.
2.2. Synthesis and characterization of veratraldehyde-based silanes, silatranes and silocanes
2.2.1. General synthesis of silanes.
The synthetic pathway for the synthesis of the Schiff base of veratraldehyde-based silanes and silatranes is depicted in Scheme 1. Firstly, veratraldehyde was added to dry toluene in a flame dried 50 ml two-neck round bottom flask fitted with a Dean stark assembly. Then, [3-(2-aminoethylamino) propyl] trimethoxysilane and 3-(diethoxy(methyl)silyl) propan-1-amine were added dropwise to the above solution and refluxed for 7 h at 70 °C, which afforded organosilanes 2a and 2b, respectively.
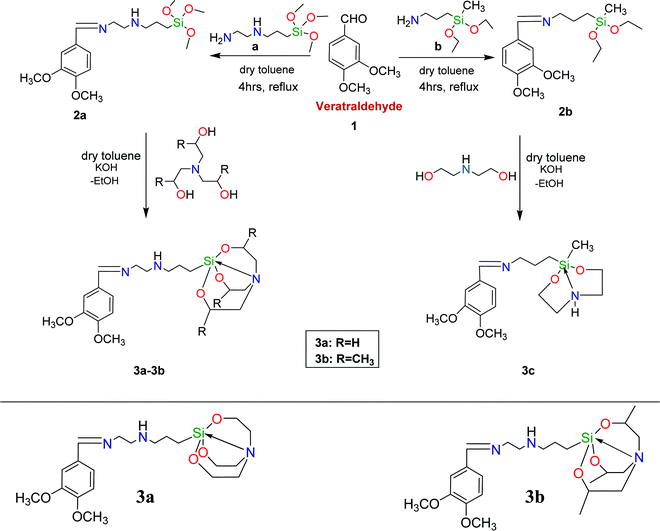 |
| Scheme 1 Synthesis of the Schiff base of veratraldehyde-based silanes and their silatranes and silocanes. | |
2.2.2. General synthesis of silatranes and silocanes.
In a flame dried two-neck round bottom flask (50 ml) fitted with Dean stark apparatus and containing dry toluene, the obtained organosilane 2a was made to react with ligands (triethanolamine and triisopropanolamine) to form 3a and 3b, respectively, and 2b was made to react with diethanolamine to form 3c. This was followed by the addition of KOH pellets in a catalytic amount and refluxing for 6 h at 70 °C. The byproducts formed were removed azeotropically through the Dean stark assembly and then dry ether was added to the reaction mixture when it had cooled down to room temperature. The desired solid products (3a–3c) were obtained after filtration under nitrogen and were dried under vacuum, whereas the product 3a was acquired after evaporation under a vacuum pump.
2.2.3. Characterization of silanes, silatranes and silocanes.
(E)-N1-(3,4-Dimethoxybenzylidene)-N2-(3-(trimethoxysilyl)propyl)ethane-1,2-diamine (2a).
Pale yellow semi-solid, yield: 89%. 1H NMR (400 MHz, CDCl3): δ 0.59 (t, 2H, Si–CH2–C), 1.22 (m, 2H, C–CH2–C), 2.35 (s, 1H, –NH), 2.61 (t, 2H, N–CH2–C), 2.87 (t, 2H, N–CH2–C), 3.46 (s, J = 3.1 Hz, 9H, SiO–CH3), 3.66 (t, 2H, N–CH2–C), 3.90 (s, 6H, –OCH3), 6.82 (d, 1H, Ar–H), 7.18 (s, 1H, Ar–H), 7.25 (d, 1H, Ar–H), 8.16 (s, 1H, –CH
N). 13C NMR (126 MHz, CDCl3): δ 15.27, 23.51, 50.06, 52.38, 56.17, 61.28, 65.82, 108.89, 110.45, 123.00, 128.21, 149.07, 151.36, 161.62. HRMS (ESI): calculated for C17H30N2O5Si [M]+: 370.1968, found: 370.1730.
(E)-N1-(3-(2,8,9-Trioxa-5-aza-1-sila-bicyclo[3.3.3]undecan-1-yl)propyl)-N2-(3,4-dimethoxybenzylidene)ethane-1,2-diamine (3a).
Yellow solid, yield: 87%, M.P. (76–78) °C. 1H NMR (400 MHz, CDCl3): δ 0.39 (t, 2H, Si–CH2–C), 1.13 (m, J = 7.0 Hz, 2H, C–CH2–C), 2.27 (s, 1H, –NH), 2.43 (t, 8H, N–CH2–C), 2.82 (t, 2H, N–CH2–C), 3.42 (t, J = 7.0 Hz, 2H, N–CH2–C), 3.53 (s, 6H, –OCH3), 3.82 (t, 6H, –OCH2–C), 7.08 (d, 1H, Ar–H), 7.17 (s, J = 7.5 Hz, 1H, Ar–H), 7.32 (d, 1H, Ar–H), 8.14 (s, J = 6.5 Hz, 1H, –CH
N). 13C NMR (126 MHz, CDCl3): δ 15.29, 21.46, 55.85, 57.08, 58.55, 65.84, 108.94, 111.06, 123.09, 125.30, 128.23, 137.85, 149.09, 151.43, 161.78. HRMS (ESI): calculated for C20H33N3O5Si [M + H]+: 424.2252, found: 424.2292.
(E)-N1-(3,4-Dimethoxybenzylidene)-N2-(3-(3,7,10-trimethyl-2,8,9-trioxa-5-aza-1-sila-bicyclo[3.3.3]undecan-1-yl)propyl)ethane-1,2-diamine (3b).
Light yellow solid, yield: 83%, M.P. (80–82) °C. 1H NMR (400 MHz, CDCl3): δ 0.44 (t, 2H, Si–CH2–C), 1.09 (s, J = 5.8 Hz, 9H, –CH3), 1.14 (m, J = 6.2 Hz, 2H, C–CH2–C), 2.06 (s, 1H, –NH), 2.43 (d, 3H, C–CH2–N), 2.47 (m, 2H, C–CH2–N), 2.58 (d, 3H, C–CH2–N), 2.98 (t, 2H, N–CH2–C), 3.66 (d, 3H, O–CH–C), 3.81 (t, J = 5.5 Hz, 2H, N–CH2–C), 3.92 (s, 6H, –OCH3), 7.02 (d, 1H, Ar–H), 7.16 (s, 1H, Ar–H), 7.37 (d, 1H, Ar–H), 8.18 (s, 1H, –CH
N). 13C NMR (126 MHz, CDCl3): δ 20.95, 21.04, 24.40, 55.19, 56.11, 63.36, 65.39, 65.89, 67.33, 110.62, 123.17, 125.44, 128.37, 129.18, 149.44, 161.83. HRMS (ESI): calculated for C23H39N3O5Si [M + H]+: 466.27, found: 466.2748.
(Z)-N-(3,4-Dimethoxybenzylidene)-3-(2-methyl-1,3,6,2-dioxazasilocan-2-yl)propan-1-amine (3c).
Light golden yellow semi-solid, yield: 80%. 1H NMR (400 MHz, CDCl3): δ 0.43 (s, 3H, –SiCH3), 1.19 (t, J = 7.0 Hz, 2H, –SiCH2), 1.47 (m, 2H, C–CH2–C), 2.34 (s, 1H, –NH), 2.65 (t, 4H, C–CH2–N), 3.44 (t, J = 7.0 Hz, 2H, C–CH2), 3.58 (s, 6H, –OCH3), 3.89 (t, 4H, O–CH2–C), 6.86 (d, 1H, Ar–H), 7.11 (s, 1H, Ar–H), 7.38 (d, 1H, Ar–H), 8.13 (s, 1H, –CH
N). 13C NMR (126 MHz, CDCl3): δ −0.56, 15.37, 25.72, 51.07, 56.06, 60.99, 65.96, 108.85, 110.55, 123.07, 129.03, 149.45, 151.37, 160.63. HRMS (ESI): calculated for C17H28N2O4Si [M]+: 352.18, found: 352.1484.
2.3. Synthesis of organosilatrane 3a functionalized hybrid silica nanoparticles (V-NPs) (Scheme 2)
When the appropriateness of the organosilatrane 3a towards Cu2+ and Fe3+ ions was confirmed, its applications were further explored by embedding 3a onto a silica surface. The Stober method was followed for the synthesis of the silica:3a conjugate. The nanoparticles were prepared by mixing 1.5 ml of TEOS in 45 ml of ethanol along with 5 ml of distilled water, followed by slow addition of a 3 ml aliquot of 25% aqueous ammonia solution to catalyze TEOS hydrolyzation, and stirring for 1 h. The solution turned milky, which indicated the formation of bare silica nanoparticles (SiO2), and then the compound 3a was added to it for post-coating treatment and stirred continuously for 24 h at room temperature. The V-NPs formed were subjected to centrifugation followed by washing with dichloromethane and then dried in air.
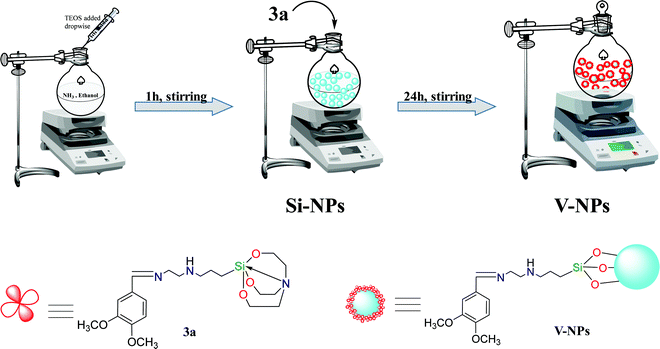 |
| Scheme 2 Schematic diagram of the synthesis of 3a-coated silica nanoparticles (V-NPs). | |
2.4. Synthesis of metal (Cu2+/Fe3+) complexes
Equimolar amounts of organosilatrane 3a and anhydrous metal chlorides of Cu2+ and Fe3+ in 25 ml of methanol were stirred for 4 h in a 50 ml round bottom flask. Then, the resultant metal complexes of Cu2+ and Fe3+ salts were filtered using a filtration unit and dried under vacuum, which yielded a green and brown precipitate, respectively. 3a–Cu2+ complex yield: 92%; 3a–Fe3+ complex yield: 89%.
2.5. UV-vis and fluorescence spectra analysis
First of all, stock solutions of various metal ions (aluminium, cobalt, copper, ferric, nickel, mercury, lead, calcium, stannous, manganese, caesium, cadmium and zinc) of 10−3 M concentration were prepared in methanol solution using chloride salts. The absorption and emission spectra were recorded on addition of 10 μL of all metal ion solutions consecutively into a 3 ml solution of 3a (1 × 10−6 M) and V-NPs (0.000483 g in 1 L of methanol), but only Cu2+ and Fe3+ ions could induce obvious changes in the absorbance and fluorescence intensity. Furthermore, titration experiments were performed to inquire about the binding properties of 3a and V-NPs towards Cu(II) and Fe(III) ions and the spectra were observed upon incremental addition of Cu2+ and Fe3+ ions up to 10 equiv. The limit of detection was calculated using a linear-calibration curve to prove the sensitivity of organosilatrane 3a and the V-NPs towards Cu2+ and Fe3+ ions, whereas the association constant (Ka) and Stern–Volmer quenching constant (KSV) were calculated by using a Benesi–Hildebrand plot and Stern–Volmer plot, respectively.
2.6. Total antioxidant activity (TAA)
The method of Arnao, Cano and Acosta (1999) was used to determine the TAA of the particles21 and is based on the ability of the anti-oxidants in the sample compound to curtail the radical cation of 2,20-azino-bis-3-(ethylbenzothiazoline-6-sulphonic acid) (ABTS). In an aqueous medium, the capacity of the compound to scavenge free radicals can be predicted from the TAA parameter. The decolouration of the ABTS radical cation indicates the scavenging of free radicals by the anti-oxidants and it was observed that quenching of the absorbance took place at 730 nm. The prepared solution contains 0.25 μM horseradish peroxidase, 2 mM ABTS and 35 μM H2O2. The solvents chloroform or DMSO were used to dissolve the particles making the final concentration of 100 mM, and then 25 μL of the prepared 100 mM solution was added to 950 μL of the ABTS solution. Furthermore, the change in the value of absorbance was recorded for 5 min at 730 nm. Henceforth, by comparing the values of the compounds (3a–3c) with the standard curve of ascorbic acid, the TAA values can be estimated. They are presented as ascorbic acid equivalents (mmol) per particle solution (100 mM). All the compounds under observation were evaluated in triplicate.
3. Results and discussion
3.1. Synthetic approach
Herein, the synthesis of the Schiff base of veratraldehyde-based silatranes and silocanes is discussed, which involves the refluxing of veratraldehyde with [3-(2-aminoethylamino) propyl] trimethoxysilane or 3-(diethoxy(methyl)silyl) propan-1-amine in dry toluene as a solvent to afford organosilanes 2a and 2b, respectively. Then, these organosilanes were coupled with different ligands via a transesterification reaction in the presence of a catalytic amount of potassium hydroxide to form the desired products (3a–3c). Furthermore, the veratraldehyde-based organosilatrane (3a) was immobilized over silica nanospheres, leading to the formation of hybrid nanoparticles (V-NPs).
3.2. Characterization
The multinuclear 1H and 13C NMR spectra were inspected and found to be consistent with the spectroscopic data of the synthesized compounds. In the 1H-NMR spectrum of compound 3a, the methoxy group (3.46 ppm) present in compound 2a is replaced by an atranyl moiety, which is confirmed by the appearance of triplets at 3.82 ppm and 3.40 ppm due to –OCH2 and –NCH2, respectively. However, the 1H-NMR spectrum of compound 3b shows complex splitting of the signals due to the presence of three stereogenic carbon atoms in the silatranyl moiety, which results in geminal as well as vicinal couplings between the OCH–CH2N and OCH–CH3 pairs. This leads to the appearance of an extremely complex multiplet at 1.09 ppm, 3.81 ppm and 3.66 ppm due to –CH3, –NCH2 and –OCH protons, respectively. Likewise, the formation of compound 3c was evidenced by the appearance of peaks at 3.47 ppm and 3.89 ppm corresponding to –CH2N and –OCH2 of the silatrane cage. The aromatic protons were observed in the range of 6.82–7.25 ppm and the protons of the Schiff base (CH
N) were observed in the range of 8.11–8.18 ppm. Moreover, proton signals due to the ethyl and propyl chains around the –NH group were also observed in the desired region. In the 13C NMR spectra of the compounds (3a–3c), carbon signals corresponding to aromatic carbon and Schiff base linkage carbon were observed in the range 108–152 ppm and 160–162 ppm, respectively. 3a and 3c showed peaks in the region 51–58 ppm and 65–66 ppm for –CH2N and –OCH2, respectively, whereas 3b exhibited separate signals for –CH3, –CH2N and –OCH2 at 20.95 ppm, 67.33 ppm and 65.89 ppm, respectively.
3.3. Characterization of the nanoparticles
The synthesis of 3a-functionalized silica nanoparticles (V-NPs) was supported by IR spectroscopy as shown in Fig. S13 (ESI†). The absorption peak of the unmodified silica nanoparticles at 1100 cm−1 corresponds to asymmetric stretching vibration of Si–O–Si. The absorption bands at 3450 and 1640 cm−1 are due to stretching and bending vibrations of physically adhered water. In the V-NPs, additional peaks appeared at 1631 cm−1 and 3432 cm−1 corresponding to –C
N and –NH, respectively, and the Si–O–Si peak shifts to 1090 cm−1. These results verify that the organic moiety 3a gets encapsulated over the silica nanoparticles.
Moreover, the phase purity and crystal structure of the V-NPs was observed using XRD. The typical amorphous nature of the V-NPs was indicated by the appearance of a single broad peak centered at a low diffraction angle of 2θ = 23° (Fig. 1a). Furthermore, EDX analysis was carried out to ascertain the elemental composition of the constituent atoms of the V-NPs (Fig. 1b). The elemental mapping showed several characteristic peaks corresponding to carbon, nitrogen, oxygen, and silicon atoms corroborating the presence of the organic precursor and silica unit, respectively. This also offers vital evidence of the covalent anchoring of 3a over the silica nanospheres. FE-SEM micrographs were used to examine the microstructure and surface morphology of the hybrid nanoparticles (V-NPs). The uniform spherical shapes of the 3a-modified silica nanoparticles at different magnifications is portrayed in Fig. 2 and this unambiguously validates the formation of V-NPs.
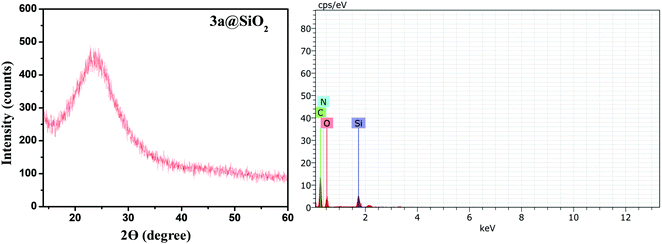 |
| Fig. 1 XRD pattern and EDX spectrum of the V-NPs. | |
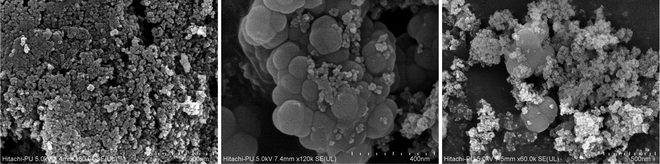 |
| Fig. 2 FE-SEM micrographs of the V-NPs at different magnifications. | |
The thermal properties of the bare silica nanoparticles (SiO2), 3a and V-NPs were investigated using thermo-gravimetric analysis (TGA) and differential scanning calorimetry (DSC). The experiments were accomplished in the temperature range of 25–1000 °C and at a scan rate of 10 °C per minute under a nitrogen atmosphere. Fig. 3a illustrates that the TGA curve of the bare silica nanoparticles does not display any remarkable weight loss up to 1000 °C, except for a weight loss of 17.11% at 50–100 °C that can be attributed to the desorption of physically adhered water and residual solvent and a 1.383% mass decrease from 550 °C due to the condensation of free silanol groups. It can be seen that the TGA curve of 3a is a two-step profile and a total 75.51% weight loss occurred due to the release of solvent and then due to the degradation of 3a leaving behind a stable inorganic residue, i.e. SiO2.
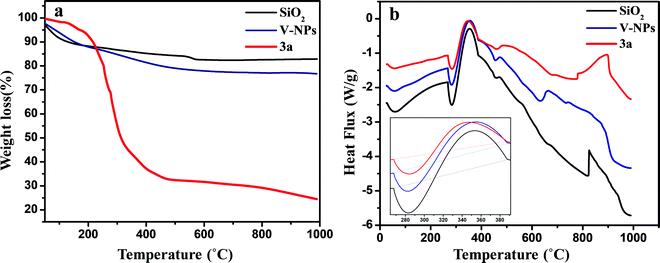 |
| Fig. 3 (a) Thermogravimetric analysis (TGA) curves and (b) DSC curves of 3a, SiO2 and the V-NPs. | |
The thermal analysis of the V-NPs exhibits weight loss of 12.58% up to 230 °C in the first step, corresponding to the loss of ethanol, in addition to water molecules participating in hydrogen bonding between the silanol groups and also that are entrapped in the inorganic matrix. A weight loss of 10.53% from 225 °C occurred in the second step, indicating that the left-over part of the V-NPs is composed of siloxane moieties and the weight loss here is more than that of the bare silica nanoparticles, substantiating the existence of an organic canopy over the surface of the silica nanoparticles. The TGA results indicate that ∼60% of 3a gets immobilized over the silica nanoparticles. Moreover, the results of the DTA curves (Fig. S14, ESI†) are also in agreement with the results of TGA.
The DSC thermograms (Fig. 3b) illustrate the study of exothermic and endothermic events corresponding to thermal decomposition, the melting point of the compounds and their temperature ranges. Basically, all the samples show a similar pattern of degradation up to 500 °C. The DSC graphs exhibit an endothermic curve at 280 °C due to the loss of water and ethanol for all compounds in the first step. In the second step, an exothermic curve appeared after 350 °C corresponding to the decomposition of the compounds. The DSC data also support the TGA–DTA results, where the SiO2, 3a and V-NPs displayed almost the same degradation pattern.
3.4. UV-Vis spectral studies of 3a and the V-NPs
To analyze the photophysical properties of the synthesized compounds, 3a (1 × 10−6 M) and its V-NPs (0.000483 g in 1 L of methanol), UV-Vis spectral analysis was performed in methanol. Compound 3a shows a single strong absorption band at 255 nm and its V-NPs display a single peak at 263 nm with a meagre red shift and an enhancement in absorption intensity as compared to 3a, corresponding to π–π* transitions as shown in Fig S15 (ESI†).
3.5. Chromogenic detection of Fe3+ and Cu2+
To explore the sensing ability of organosilatrane 3a and its V-NPs towards a broad range of metal cations, like Al3+, Fe3+, Mn2+, Cu2+, Ni2+, Hg2+, Zn2+, Sn2+, Co2+, Ca2+, Cs2+, Cd2+ and Pb2+ in their chloride form using methanol solvent, chromogenic detection studies were performed. The spectra illuminate that a change in the peak of organosilatrane 3a was spotted only on the introduction of Cu2+ and Fe3+ ions, while no perturbations were marked on addition of other metal ions (Fig. 4). Moreover, a color change from yellow to light green and colorless was observed only in the presence of Cu2+ and Fe3+ ions, respectively. However, when both the metal ions were added simultaneously, a light greenish-yellow color appeared. This behavior allows us to differentiate Cu2+ ions from Fe3+ ions facilitating naked eye detection. A bar graph representation of compound 3a and the V-NPs showing the change in absorbance on the introduction of various metal ions in methanol at λ = 302 nm is shown in Fig. 5. Furthermore, the influence of the treatment of 3a and the V-NPs upon incremental inclusion of Cu2+ and Fe3+ ions was examined as shown in Fig. 6. The results exhibit that with the successive injection of Cu2+ and Fe3+, a bathochromic shift along with an additional band was witnessed for the original peak and hyperchromic shifts for the new peaks were detected. In the case of titration of 3a and the V-NPs in the presence of Cu2+ ions, a red shift was seen from 255 nm to 269 nm and 263 nm to 272 nm, respectively, and also one additional band appeared at 298 nm and 304 nm, respectively. In the case of the titration of 3a and the V-NPs with Fe3+ ions, a red shift was noticed from 255 nm to 270 nm and 263 nm to 274 nm, respectively, and along with this one additional band was observed at 302 nm and 306 nm, respectively. The appearance of the new bands due to ligand to metal charge transfer in the UV–vis spectra upon titration indicates the complex formation of 3a and the V-NPs with the Cu2+ and Fe3+ ions.
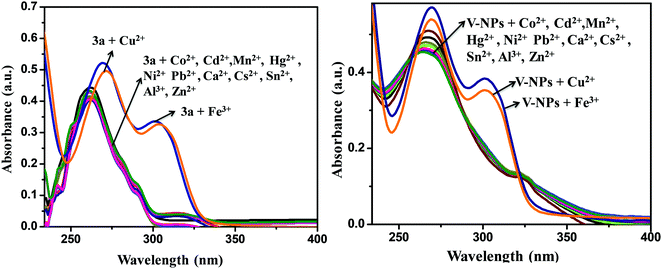 |
| Fig. 4 UV-visible absorption spectra of the compound 3a and V-NPs in the presence of various metal ions in methanol. | |
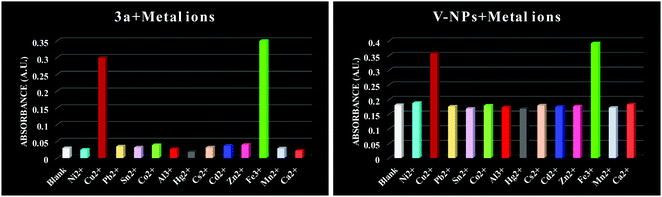 |
| Fig. 5 Bar graph representation of absorbance against various metal ions of compound 3a and the V-NPs in methanol at λ = 302 nm. | |
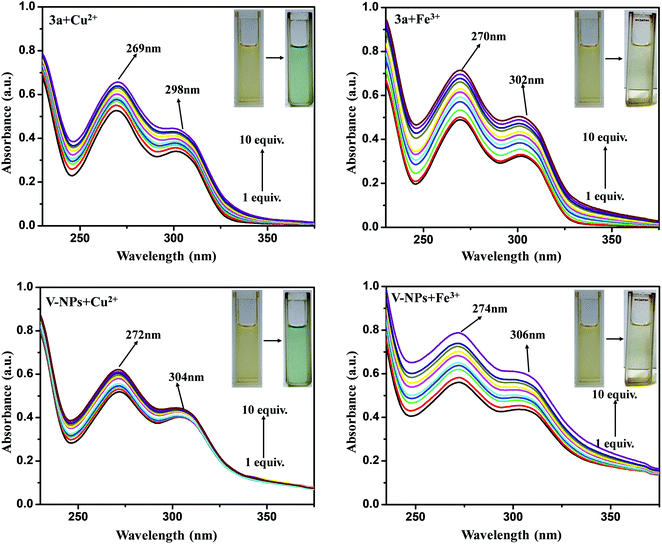 |
| Fig. 6 UV-visible titration spectra of compound 3a and the V-NPs upon the addition of increasing concentrations of Cu2+ and Fe3+ ions in methanol. Insets: their corresponding color change in daylight. | |
pH sensing.
In order to assess the stability of the 3a and V-NP sensors, we also studied their sensitivity to pH in the absence and presence of Cu2+ and Fe3+ ions. pH titration experiments were carried out to evaluate the effect of change in pH on the absorption intensity of the compound 3a and V-NPs at 255 nm in the highly acidic and basic pH range (Fig. S16, ESI†). The pH of the original compound 3a and the V-NPs was 5.3 and 5.8, respectively. The pH values were adjusted with 0.1 N NaOH and 0.1 N HCl solution. The absorbance of the free ligand 3a seems to be the most stable at pH 7.40, whereas the V-NPs remain stable at pH 8.20. Furthermore, the pH-dependence of the absorption intensity of the 3a–Cu2+/Fe3+ and V-NPs–Cu2+/Fe3+ complexes was evaluated (Fig. 7). The results indicate that the absorption intensity of 3a at 269 nm remains almost stable over a pH range of 4–9 and the absorption intensity of the V-NPs at 274 nm remains almost constant over a pH wide range of 4–10. Thus, the results demonstrate that the 3a and V-NP sensors are suitable for detecting Cu2+ and Fe3+ ions under physiological conditions. Moreover, the investigation revealed that the V-NPs remain stable at more pH values than 3a in the absence and presence of Cu2+ and Fe3+ ions.
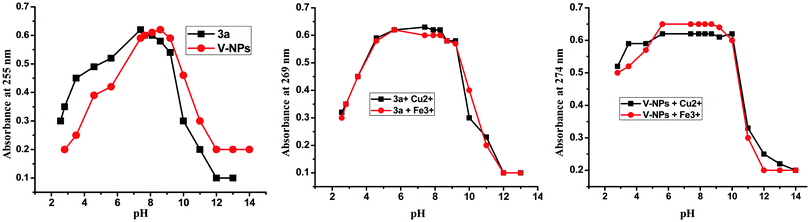 |
| Fig. 7 UV-visible titration plots of compound 3a and the V-NPs in the absence and presence of Cu2+ and Fe3+ ions as a function of pH value. | |
Stoichiometry, binding constant and limit of detection.
Job's continuous variation method monitored by absorbance intensity was employed to determine the binding stoichiometry of the compound 3a and V-NPs with Cu2+ and Fe3+ ions. All the plots demonstrated in Fig. S17 (ESI†) gave a maximum at 0.5, revealing that the stoichiometry of the complex is 1
:
1.
Furthermore, the binding mechanism was employed to depict the association constant (Ka), a measure of the strength of interaction of the probe 3a and V-NPs with Cu2+ and Fe3+ ions. Assuming 1:n stoichiometry, the equilibrium is given by eqn (1).
| 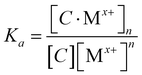 | (2) |
where C =
3a, V-NPs and [M
x+] = Cu
2+, Fe
3+.
The evaluation of the UV-vis titration plot was performed such that the plot between [A − A0]−1vs. [Mx+]n has linearity of regression, where [A − A0]−1 is the change in the intensity of absorbance of the compounds in the presence of metal ions at a particular wavelength.22 The linear graph of [A − A0]−1vs. [Mx+]n with a high regression coefficient (R2 > 0.9) also confirmed 1
:
1 stoichiometry of the complexes. The binding constant for 3a and the V-NPs with Cu2+ and Fe3+ ions was determined by the Benesi–Hildebrand (B–H) method using UV-vis spectrometric titration data at 269 nm (Fig. S18, ESI†). It was found that the association constant Ka for the V-NPs was higher than for 3a, specifying the strong binding ability of the V-NPs with Cu2+ and Fe3+ metal ions. The calculation of the limit of detection was achieved from the calibration plot using LOD = 3σ/K, where σ is the standard deviation and K is the slope of the curve (Fig. S19, ESI†). The results reveal that the V-NPs in the presence of Cu2+ and Fe3+ ions have a lower limit of detection as compared to 3a on addition of Cu2+ and Fe3+ ions. This is because the size of the 3a particles is progressively reduced in the V-NPs, which results in a change in physical properties. The interaction of any 3a particle or V-NP with the metal ions mainly occurs at the surface of the particle. A large surface extension of the V-NPs as compared to 3a at a fixed mass results in larger interaction of the V-NPs with the Cu2+/Fe3+ ions, and hence lower LODs as compared to 3a (Table 1).
Table 1 Binding constant values and LOD values for 3a and the V-NPs towards Cu2+ and Fe3+
Sensors |
Sensing metal ions |
K
a (103 M−1) |
LOD (μM) |
3a
|
Cu2+ |
12.47 |
1.75 |
3a
|
Fe3+ |
3.35 |
1.35 |
V-NPs |
Cu2+ |
15.55 |
0.10 |
V-NPs |
Fe3+ |
32.51 |
0.34 |
Selectivity of 3a towards Cu2+ and Fe3+ ions.
In order to study the interference of coexistent metal ions, competitive experiments were carried out in methanol solution. 3a and V-NPs (10−6 M) were treated with 20 μL solutions of Cu2+ and Fe3+ ions in the presence of 20 μL solutions of other interfering metal ions and their UV–vis spectra were recorded. Fig. 8 indicates that the presence of various competitive metal ions had little impact on the recognition of Cu2+ and Fe3+ ions implying that 3a and the V-NPs are highly selective towards Cu2+ and Fe3+ ions. Therefore, 3a and the V-NPs can act as a selective Cu2+ and Fe3+ ion colorimetric sensor, which can be credited to the size of only the Cu2+/Fe3+ ions fitting in the cage size of the molecule.
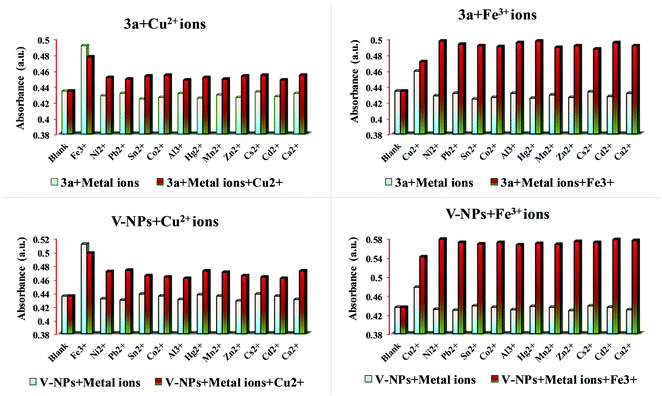 |
| Fig. 8 Metal ion selectivity profiles of 10−6 M of 3a and V-NPs (3.0 ml) in the presence of 10−3 M of various metal ions (20.0 μL for Cu2+/Fe3+ ions and 20.0 μL for other metal ions) at λ = 269 nm. | |
Time-dependent studies.
The time course studies of both the 3a and V-NP sensors in the presence of Cu2+ and Fe3+ ions at 269 nm were investigated. The absorbance intensity for 3a on addition of Cu2+ and Fe3+ ions increased rapidly within 12 s and 10 s, respectively, whereas for the V-NPs, the absorbance intensity reached the maximum within 8 s and 6 s, respectively, and stabilized thereafter, suggesting that the complexation of sensor 3a and the V-NPs with Cu2+ and Fe3+ ions was quite rapid under optimized conditions (Fig. S21, ESI†). Moreover, the V-NPs possess good responsiveness to Cu2+ and Fe3+ ions as compared to 3a and thus can be used as an effective tool for real-time monitoring and detection of Cu2+/Fe3+ ions. The comparison of sensors 3a and the V-NPs with a few reported sensors has been provided in Table 2.
Table 2 Comparison of sensors 3a and the V-NPs with a few reported sensors
Measured signal |
Metal ion |
Response time (s) |
Linear range (s) |
Reference |
Probe |
Fluorescence |
Cu2+ |
120–360 |
100–360 |
23
|
Spirobenzopyran |
Absorbance |
Cu2+ |
180–400 |
100–180 |
24
|
Spiropyran |
Fluorescence |
Cu2+/Fe3+ |
60 |
0–60 |
25
|
Terthiophene–phenylamine–derived fluorescent sensor TTA |
Fluorescence |
Fe3+ |
20 |
0–20 |
26
|
Schiff-base derivative DN |
Fluorescence |
Fe3+ |
120 |
0–120 |
27
|
Rhodamine–pyridine conjugated spectroscopic probe RhP |
Absorbance |
Fe3+ |
10 |
0–10 |
Present Work |
3a
|
Absorbance |
Cu2+ |
12 |
0–12 |
Present Work |
3a
|
Absorbance |
Fe3+ |
6 |
0–6 |
Present Work |
V-NPs |
Absorbance |
Cu2+ |
8 |
0–8 |
Present Work |
V-NPs |
3.6. Fluorimetric response of 3a and the V-NPs
The metal ion sensing properties of 3a (1 × 10−6 M) and the V-NPs (0.000483 g in 1 L of methanol) were scrutinized in methanol solution at an excitation wavelength of 255 nm upon addition of various metal ions (Al3+, Fe3+, Mn2+, Cu2+, Ni2+, Hg2+, Zn2+, Sn2+, Co2+, Ca2+, Cs2+, Cd2+ and Pb2+ in their chloride form) (1 × 10−3 M) by fluorescence spectroscopy (Fig. 9). The original fluorescence intensity of 3a and the V-NPs showed complete quenching behavior upon gradual addition of Cu2+ and Fe3+ ions, denoting an efficient fluorescence turn-off response.28 In contrast, almost trivial changes in fluorescence intensity were observed on injection of various other metal ions. The significant fluorescent quenching and the absence of any kind of peak shift of the 3a and V-NPs could be ascribed to the paramagnetic nature of Cu2+ and Fe3+ ions with unfilled d-shells, which propelled the fluorophore to open a non-radiative deactivation channel. This resulted in the transfer of excitation energy or electron transfer from the fluorophore of our compound 3a and the V-NPs to the empty d-orbitals of the copper and iron metals. Furthermore, fluorescence titration experiments were conducted to understand the binding properties of 3a and the V-NPs with Cu2+ and Fe3+ ions. Upon consecutive addition of Cu2+ and Fe3+ ions, the fluorescence emission intensity of 3a and the V-NPs decreased dramatically on subsequent addition of 10 equiv. of Cu2+ and Fe3+ ion solution (Fig. 10).
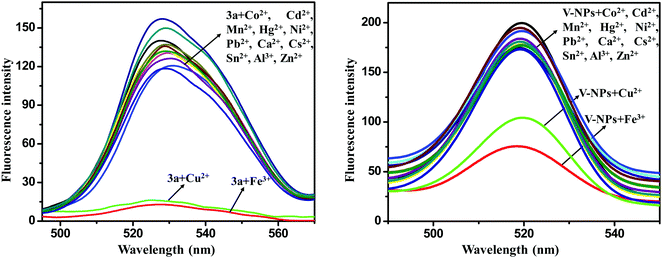 |
| Fig. 9 Fluorescence spectra of the compound 3a and V-NPs in the presence of various metal ions in methanol. | |
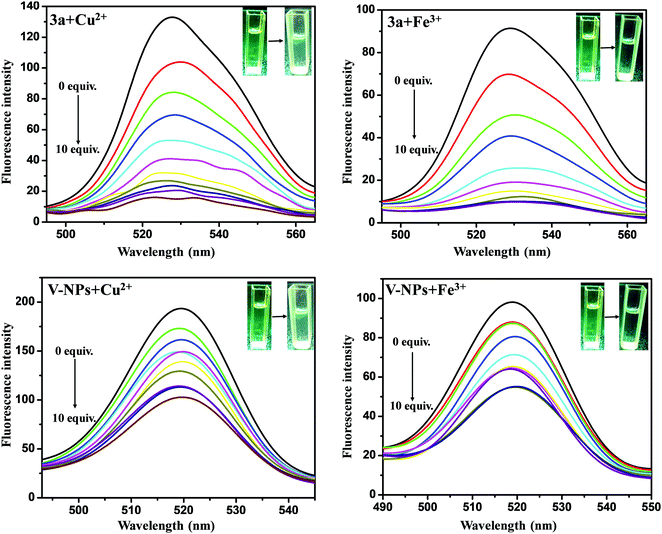 |
| Fig. 10 Changes in the fluorescence titration spectra of 3a and the V-NPs on consecutive addition of Cu2+ and Fe3+ ions in methanol. Insets: their corresponding color change. | |
Limit of detection and Stern–Volmer constant.
Moreover, a deeper insight into the fluorescence quenching behavior of the synthesized receptors was gained by using the Stern–Volmer equation:
where F0 and F are the intensities in the absence and presence of Cu2+ and Fe3+ metal ions. KSV is the Stern–Volmer constant and [Q] is the quencher concentration.29F0/F was plotted against the Cu2+ and Fe3+ ion concentration in an effort to calculate the Stern–Volmer quenching constant and limit of detection to prove the robust connection and sensitivity of 3a and the V-NPs towards Cu2+ and Fe3+ ions, respectively, at 527 nm. The plots as shown in Fig. S21 (ESI†) revealed a good linear relationship, which can be attributed to the static quenching of the ligands by Cu2+ and Fe3+ ions. Furthermore, the highest value of KSV and lowest value of limit of detection (LOD) were shown by the V-NPs with Cu2+ or Fe3+ proving their higher binding affinity and better sensing ability. The obtained limit of detection (LOD) and Stern–Volmer quenching constant (KSV) are given in Table 3.
Table 3
R
2, KSV and LOD values for 3a and the V-NPs towards Cu2+ and Fe3+ from the Stern–Volmer plot
Sensor |
Sensing metal ions |
R
2
|
K
SV (106 M−1) |
LOD (μM) |
3a
|
Cu2+ |
0.98688 |
11.34 |
3.814 |
3a
|
Fe3+ |
0.95240 |
3.09 |
4.077 |
V-NPs |
Cu2+ |
0.98471 |
39.51 |
3.642 |
V-NPs |
Fe3+ |
0.99157 |
7.84 |
1.681 |
The various chemosensors that have been employed to date for the detection of Cu2+ and Fe3+ ions and a comparison of their sensing ability are provided in Table 4.
Table 4 Comparison of a few reported sensors for Cu2+ and Fe3+ ions
Measured signal |
Metal ion |
LOD (μM) |
Ref. |
Sensors |
Fluorescence |
Fe3+ |
50 |
30
|
Sensor based on bis(rhodamine) |
Absorbance |
Cu2+ |
10 |
31
|
Ferrocene chalcone triazole triad |
Absorbance |
Cu2+ |
2.92 |
32
|
Adenine containing organosilicon nucleobase |
Fluorescence |
Fe3+ |
8.54 |
33
|
Arene based fluorescent probe |
Fluorescence |
Cu2+ |
3.98 |
34
|
Luminescent cadmium polymer |
Fluorescence |
Fe3+ |
1.681 |
Present Work |
— |
Fluorescence |
Cu2+ |
3.642 |
Present Work |
— |
Absorbance |
Fe3+ |
0.34 |
Present Work |
— |
Absorbance |
Cu2+ |
0.10 |
Present Work |
— |
Sensor selectivity.
In order to explore the utility and sensor selectivity of the chemosensors 3a and V-NPs towards Cu2+ and Fe3+ ions, competitive experiments were performed with other relevant metal ions (Al3+, Fe3+, Mn2+, Cu2+, Ni2+, Hg2+, Zn2+, Sn2+, Co2+, Ca2+, Cs2+, Cd2+ and Pb2+ in their chloride form) in the presence of Cu2+ and Fe3+ ions. It can be seen from Fig. 11 that the fluorescence quenching caused by the mixture of Cu2+/Fe3+ ions with other metal ions was very similar to that caused by Cu2+ and Fe3+ ions only. The competitive metal ions did not alter the relative emission intensity of 3a and the V-NPs significantly. It was observed that common interfering cations did not interfere in the selective detection of Cu2+ and Fe3+ ions, thereby revealing the excellent selectivity of this method. The excellent selectivity of 3a and the V-NPs can probably be attributed to the strong interaction of 3a and the V-NPs with Cu2+ and Fe3+ ions.
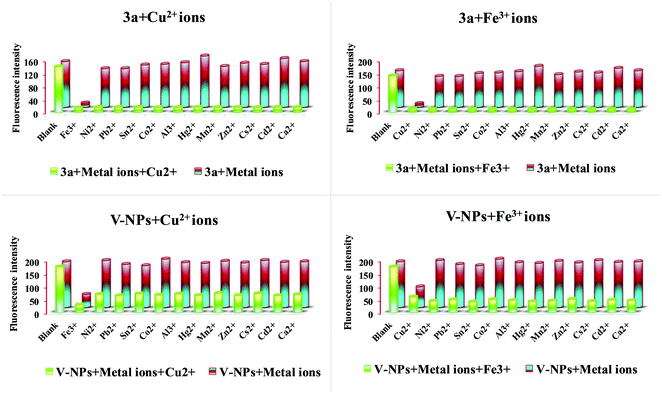 |
| Fig. 11 Metal ion selectivity profiles of 10−6 M of 3a and V-NPs (3.0 ml) in the presence of 10−3 M of various metal ions (20.0 μL for Cu2+/Fe3+ ions and 20.0 μL for other metal ions) at λ = 269 nm. | |
3.7. Biological assay
Anti-oxidants are compounds that curb oxidation processes and are measured in terms of TAA. They also act as a defense system of the human body against free radical attack. The compounds (3a–3c) were studied for antioxidant activity as listed in Table 5 and it was found that the compound 3a shows a good anti-oxidant activity value, i.e. 4.92 ± 0.02, as depicted in Fig. 12.
Table 5 Total antioxidant activity expressed as the equivalent of ascorbic acid (mM) per mg of sample dissolved in chloroform
Sample code |
TAA (eq. ascorbic acid) mM per mg of particle |
3a
|
4.92 ± 0.02 |
3b
|
0.94 ± 0.04 |
3c
|
0.06 ± 0.02 |
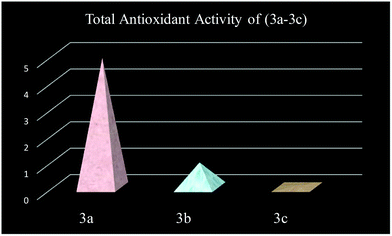 |
| Fig. 12 Total antioxidant activity of compounds 3(a–c). | |
3.8. Plausible sensing mechanism of 3a towards Cu2+/Fe3+
A plausible mode of interaction of 3a with Cu2+ and Fe3+ metal ions is depicted in Scheme 3. The probe 3a possess four metal binding sites (O and N atoms) that can bind with Cu2+ and Fe3+. The sensing mechanism for the selective sensing of 3a towards Cu2+ and Fe3+ may proceed via complexation to form different organometallic compounds 3a–Cu2+ and 3a–Fe3+. The weak fluorescence of 3a may be due to a PET process. The lone pairs of electrons on the nitrogen and oxygen donors are induced in the molecule 3a by the exciting radiation causing a sufficient PET process in the molecule directed from the receptor to the fluorophore leading to weak fluorescence. When the sensor 3a coordinated with Cu2+/Fe3+, the significant fluorescence quenching could be ascribed to the CHEQ effect of ligand-to-metal charge transfer (LMCT) between 3a and the Cu2+/Fe3+ ion. Both Cu2+ and Fe3+ being paramagnetic with an unfilled d-shell evoked the fluorophore to open a non-radiative deactivation channel, which leads to fluorescence quenching through electron and/or energy transfer processes from the fluorophore to the empty d-orbital of the Cu2+/Fe3+ ion. Furthermore, with the aim of deducing the binding sites of the sensor conjugates 3a–Cu2+ and 3a–Fe3+, FT-IR spectroscopy was implemented. The –NH and Schiff base (C
N) peaks shift from 3433 and 1640 cm−1 to 3425 and 1620 cm−1, respectively, whereas the peak at 1023 cm−1 in 3a attributed to the characteristic frequency of Si–O disappears on interaction with Cu2+ ions implying the formation of a 3a + Cu2+ metal complex. Furthermore, the perturbation in –NH, Schiff base (C
N) and –OCH3 peaks from 3433, 1640 and 2771 cm−1 to 3427, 1630 and 2980 cm−1 confirmed that Fe3+ ions are involved in bonding with 3a through nitrogen and oxygen atoms as shown in Fig. S22 (ESI†).
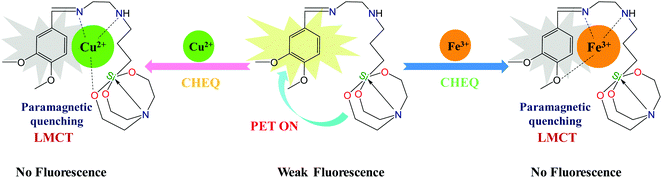 |
| Scheme 3 Plausible mode of interaction of compound 3a with Cu2+ and Fe3+ ions. | |
3.9. Theoretical study and computational details
The optimization and quantum chemical calculations of structures 3a, 3a + Cu2+ and 3a + Fe3+ at the DFT level were investigated using B3LYP in combination with the 6-31 + G(d,p) basis set for H, C, N, Si and O atoms, along with the LANL2DZ basis set for Cu2+ and Fe3+. The energy minimized structures display that the arrangement of binding sites of ligand 3a offered an appropriate cavity for the Cu2+ and Fe3+ metal ions (Fig. 13). The frontier molecular orbitals shed light on the organization of the HOMO and LUMO and it is found that in ligand 3a and 3a + Cu2+, the HOMO resides mainly on the silatrane cage, whereas in 3a + Fe3+ it is delocalized on the veratraldehyde part of the compound. Moreover, the LUMO is allocated over the veratraldehyde part in 3a but in the case of 3a + Cu2+, the LUMO extended over the silatranyl group, –NH and –C
N group. Furthermore, in 3a + Fe3+, the LUMO is distributed over the methoxy group, –NH and –C
N group whereas no electron cloud is envisaged over the silatranyl moiety. The energy gaps between the HOMO and LUMO are 0.16586 eV, 0.10132 eV and 0.13362 eV for 3a, 3a +Cu2+ and 3a + Fe3+, respectively (Fig. 14). Thus, the higher stability of the metal complexes can be elucidated from their lower energy gap as compared to 3a.
 |
| Fig. 13 Optimized structures of 3a and its complexes with Cu2+ and Fe3+. | |
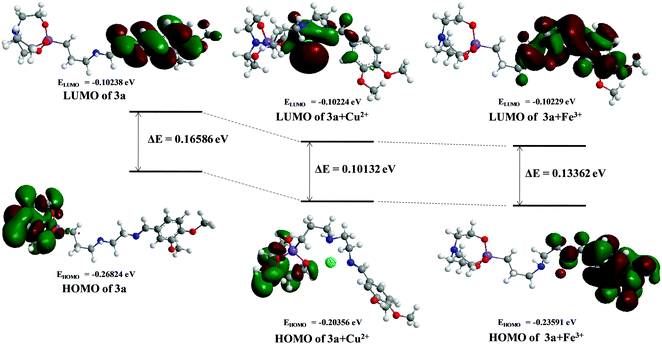 |
| Fig. 14 DFT computed LUMO and HOMO diagrams of ligand 3a, 3a + Cu2+ and 3a + Fe3+. | |
4. Conclusion
In conclusion, we have synthesized the Schiff base of veratraldehyde-based organosilatranes and organosilocanes (3a–3c) and the compound 3a was successfully fabricated over bare silica nanoparticles to yield nanohybrids (V-NPs). The compound 3a and its hybrid nanoparticles (V-NPs) exhibited commendable selectivity towards the detection of Cu2+ and Fe3+ ions over a wide pH range from 4–9. Upon binding with Cu2+ and Fe3+ ions, the compound 3a and V-NPs displayed an obvious color change from yellow to light green and colorless, respectively, which can be readily seen by the naked eye. Moreover, the 3a and V-NPs behave as “turn-off” fluorescent chemosensors on interaction with Cu2+ and Fe3+ ions manifesting entirely different spectral features. The studies suggested 1
:
1 binding stoichiometry of 3a and the V-NPs with Cu2+ and Fe3+ ions. The binding of the Cu2+/Fe3+ ions with 3a was confirmed by FT-IR spectroscopy and a computational study following the DFT approach. The hybrid silica nanoparticles (V-NPs) give a higher association constant value with Cu2+ and Fe3+ ions than compound 3a. Also, the convincingly low detection limit of 3a and the V-NPs for Cu2+ (0.10–1.75 μM) and Fe3+ (0.34–1.35 μM) is far below the guidelines of the WHO (31.5 μM for Cu2+ and 5.37 μM for Fe3+).35 Thus, the fabrication of silica over probe 3a leads to enhancement of the sensing properties towards Cu2+ and Fe3+ ions. Furthermore, the response time and the dual sensing ability for Cu2+ and Fe3+ ions is much better in the case of the nanohybrid, which facilitates the removal of these trace ions, making this protocol useful for practical applications. The total anti-oxidant activity of the compounds (3a–3c) was also explored, which gave marvelous results. Thus, the present work reported a new veratraldehyde-based naked eye dual-responsive chemosensor for the detection of Cu2+ and Fe3+ ions among various metal ions.
Conflicts of interest
There are no conflicts to declare.
Acknowledgements
The authors gratefully acknowledge the financial support provided by the University Grant Commission (UGC), New Delhi, through a SRF, the Council of Scientific and Industrial Research (CSIR), India and DST-PURSE.
References
- E. M. Culbertson, V. M. Bruno, B. P. Cormack and V. C. Culotta, Expanded role of the Cu-sensing transcription factor Mac1p in Candida albican, Mol. Microbiol., 2020, 114, 1006–1018 CrossRef CAS PubMed.
- S. Malkondu, D. Turhan and A. Kocak, Copper(II)-directed static excimer formation of an anthracene-based highly selective fluorescent receptor, Tetrahedron Lett., 2015, 56, 162–167 CrossRef CAS.
- Z. Zhou, H. Tang, S. Chen, Y. Huang, X. Zhu, H. Li, Y. Zhang and S. Yao, A turn-on red-emitting fluorescent probe for determination of copper(II) ions in food samples and living Zebrafish, Food Chem., 2021, 343, 128513 CrossRef CAS PubMed.
- L. Yang, N. Huang, L. Huang, M. Liu, H. Li, Y. Zhang and S. Yao, Electrochemical sensor for highly sensitive detection of copper ion based on a new molecule probe Pi-A decorated on graphene, Anal. Methods, 2017, 9, 618–624 RSC.
- A. Farhi, F. Firdaus, H. Saeed, A. Mujeeb, M. Shakir and M. Owais, Aquinoline-based fluorescent probe for selective detection and real-time monitoring of copper ions – a differential colorimetric approach, Photochem. Photobiol. Sci., 2019, 18, 3008 CrossRef CAS PubMed.
- B. Kaur, N. Kaur and S. Kumar, Colorimetric metal ion sensors – A comprehensive review of the years 2011–2016, Coord. Chem. Rev., 2018, 358, 13–69 CrossRef CAS.
- S. Lutsenko, K. Petrukhin, M. J. Cooper, C. T. Gilliam and J. H. Kaplan, N-terminal Domains of Human Copper-transporting Adenosine Triphosphatases (the Wilson's and Menkes Disease Proteins) Bind Copper Selectively in Vivo and in Vitro with Stoichiometry of One Copper Per Metal-binding Repeat, J. Biol. Chem., 1997, 272, 18939–18944 CrossRef CAS PubMed.
- X. Zhou, X. Guo, L. Liu, H. Zhai, Q. Meng, Z. Shi and X. Tai, Two d10 luminescent metal–organic frameworks as dual functional luminescent sensors for (Fe3+, Cu2+) and 2,4,6-trinitrophenol (TNP) with high selectivity and sensitivity, RSC Adv., 2020, 10, 4817 RSC.
- V. K. Gupta, N. Mergua and L. K. Kumawat, A new multifunctional rhodamine-derived probe for colorimetric sensing of Cu(II) and Al(III) and fluorometric sensing of Fe(III) in aqueous media, Sens. Actuators, B, 2016, 223, 101–113 CrossRef CAS.
- D. W. M. Pincher, C. A. Bader, J. D. Hayball, S. E. Plush and M. J. Sweetman, Graphene Quantum Dot Embedded Hydrogel for Dissolved Iron Sensing, ChemistrySelect, 2019, 4, 9640–9646 CrossRef CAS.
- J. S. Becker, A. Matusch, C. Depboylu, J. Dobrowolska and M. V. Zoriy, Quantitative Imaging of Selenium, Copper, and Zinc in Thin Sections of Biological Tissues (Slugs−Genus Arion) Measured by Laser Ablation Inductively Coupled Plasma Mass Spectrometry, Anal. Chem., 2007, 79, 6074–6080 CrossRef CAS PubMed.
- J. C. Yu, J. M. Lo and K. M. Wai, Extraction of gold and mercury from sea water with bismuth diethyl dithiocarbamate prior to neutron activation—spectrometry, Anal. Chim. Acta, 1983, 154, 307–312 CrossRef CAS.
- M. Ghaedi, K. Mortazavi, M. Montazerozohori, A. Shokrollahi and M. Soylak, Flame atomic absorption spectrometric (FAAS) determination of copper, iron and zinc in food samples after solid-phase extraction on Schiff base-modified duolite XAD 761, Mater. Sci. Eng., C, 2013, 33, 2338–2344 CrossRef CAS PubMed.
- X. Zhu, Y. Duan, P. Li, H. Fan, T. Han and X. Huang, A highly selective and instantaneously responsive Schiff base fluorescent sensor for the “turn-off” detection of iron(III), iron(II), and copper(II) ions, Anal. Methods, 2019, 11, 642–647 RSC.
- Y. Chan, Y. Jin, C. Wu and D. T. Chiu, Copper(II) and iron(II) ion sensing with semiconducting polymer dots, Chem. Commun., 2011, 47, 2820–2822 RSC.
- E. A. Dikusar, N. A. Zhukovskaya, K. L. Moiseichuk, E. G. Zalesskaya, P. V. Kurman and O. G. Vyglazov, Preparative Synthesis of Veratraldehyde and Citral Oxime Esters, Russ. J. Appl. Chem., 2008, 81, 643–646 CrossRef CAS.
- S. Irudhaya Raj, A. Jaiswal and I. Uddin, Tunable porous silica nanoparticles as a universal dye adsorbent, RSC Adv., 2019, 9, 11212–11219 RSC.
-
V. L. Fleisher and M. V. Andryukhova, Preparative synthesis of fragrance substances based on vanillin and veratraldehyde, Chemistry, Technology of Organic Substances, Materials and Goods, 2012, ISSN 1683-0377 Search PubMed.
- I. Khan, K. Saeed and I. Khan, Nanoparticles: Properties, applications and toxicities, Arabian J. Chem., 2019, 12, 908–931 CrossRef CAS.
- P. Rupp, C. Burger, N. G. Kling, M. Kubel, S. Mitra, P. Rosenberger, T. Weatherby, N. Saito, J. Itatani, A. S. Alnaser, M. B. Raschke, E. Ruhl, A. Schlander, M. Gallei, L. Seiffert, T. Fennel, B. Bergues and M. F. Kling, Few-cycle laser driven reaction nanoscopy on aerosolized silica nanoparticles, Nat. Commun., 2019, 10, 4655 CrossRef PubMed.
- M. B. Arnao, A. Cano and M. Acosta, Methods to measure the antioxidant activity in plant material, Free Radical Res., 1999, 31, 389–396 CrossRef PubMed.
- G. Singh, Pawan, A. Singh, Shilpy, Diksha, Suman, G. Sharma, S. C. Sahoo and A. Kaur, Propargyl-functionalized single arm allied Anthracene based Schiff bases: Crystal structure, solvatochromism and selective recognition of Fe3+ ion, J. Mol. Struct., 2021, 1229, 129618 CrossRef CAS.
- N. Shao, J. Y. Jin, H. Wang, Y. Zhang, R. H. Yang and W. H. Chan, Tunable Photochromism of Spirobenzopyran via Selective Metal Ion Coordination: An Efficient Visual and Ratioing Fluorescent Probe for Divalent Copper Ion, Anal. Chem., 2008, 80, 3466–3475 CrossRef CAS PubMed.
- N. Shao, Y. Zhang, S. Cheung, R. Yang, W. Chan, T. Mo, K. Li and F. Liu, Copper Ion-Selective Fluorescent Sensor Based on the Inner Filter Effect Using a Spiropyran Derivative, Anal. Chem., 2005, 77, 7294–7303 CrossRef CAS PubMed.
- J. Wang, T. Wei, F. Ma, T. Li and Q. Niu, A novel fluorescent and colorimetric dual-channel sensor for the fast, reversible and simultaneous detection of Fe3+ and Cu2+ based on terthiophene derivative with high sensitivity and selectivity, J. Photochem. Photobiol., A, 2019, 383, 111982 CrossRef CAS.
- Z. Zuoa, X. Songa, D. Guoa, Z. Guob and Q. Niu, A dual responsive colorimetric/fluorescent turn-on sensor for highly selective, sensitive and fast detection of Fe3+ ions and its applications, J. Photochem. Photobiol., A, 2019, 382, 111876 CrossRef.
- M. Shellaiah, N. Thirumalaivasan, B. Aazaad, K. Awasthi, K. W. Sun, S. Wu, M. Lin and N. Ohta, Novel rhodamine probe for colorimetric and fluorescent detection of Fe3+ ions in aqueous media with cellular imaging, Spectrochim. Acta, Part A, 2020, 242, 118757 CrossRef CAS PubMed.
- A. Fang, H. Chen, H. Li, M. Liu, Y. Zhang and S. Yao, Glutathione regulation-based dual-functional upconversion sensing-platform for acetylcholinesterase activity and cadmium ions, Biosens. Bioelectron., 2017, 87, 545–551 CrossRef CAS PubMed.
- Z. Ma, Y. Sun, J. Xie, P. Li, Q. Lu, M. Liu, P. Yin, H. Li, Y. Zhang and S. Yao, Facile Preparation of MnO2 Quantum Dots with Enhanced Fluorescence via Microenvironment Engineering with the Assistance of Some Reductive Biomolecules, ACS Appl. Mater. Interfaces, 2020, 12, 15919–15927 CrossRef CAS PubMed.
- T. O. Hirayama and K. Nagasawa, A highly selective turn-on fluorescent probe for iron(II) to visualize labile iron in living cells, Chem. Sci., 2013, 4, 1250 RSC.
- G. Singh, A. Arora, S. Rani, P. Kalra and M. Kumar, A click-generated triethoxysilane tethered ferrocene-chalcone-triazole triad for selective and colorimetric detection of Cu2+ ions, ChemistrySelect, 2017, 2, 3637 CrossRef CAS.
- G. Singh, G. Sharma, P. Kalra, Sanchita, V. Verma and V. Ferretti, Synthesis and structural characterization of first adenine containing organosilicon nucleobase for the recognition of Cu2+ ion, Inorg. Chim. Acta, 2018, 479, 74 CrossRef CAS.
- P. Kumar, V. Kumar and R. Gupta, Arene-based fluorescent probes for the selective detection of iron, RSC Adv., 2015, 5, 97874 RSC.
- J. Zhang, J. Wu, G. Tang, J. Feng, F. Luo, B. Xu and C. Zhang, Multi- responsive water-stable luminescent Cd coordination polymer for detection of TNP and Cu2+, Sens. Actuators, B, 2018, 272, 166 CrossRef CAS.
- T. G. Jo, J. M. Jung, J. Han, M. H. Lim and C. Kim, A single fluorescent chemosensor for multiple targets of Cu2+, Fe2+/3+ and Al3+ in living cells and a near-perfect aqueous solution, RSC Adv., 2017, 7, 28723 RSC.
Footnote |
† Electronic supplementary information (ESI) available. See DOI: 10.1039/d1nj05105a |
|
This journal is © The Royal Society of Chemistry and the Centre National de la Recherche Scientifique 2022 |