DOI:
10.1039/D1NJ04836H
(Paper)
New J. Chem., 2022,
46, 334-344
Eu(III), Tb(III) activated/co-activated K2NaAlF6 host array: simultaneous approach for improving photovoltaic efficiency and tricolour emission†
Received
9th October 2021
, Accepted 20th November 2021
First published on 22nd November 2021
Abstract
Rare earth-activated fluoride phosphors have generated attention in recent years in the field of solid-state lighting and solar cell efficiency enhancement. In the present study, RE (RE = Eu3+, Tb3+) activated/co-activated K2NaAlF6 phosphor was synthesized using a low-temperature wet chemical method. The photoluminescence (PL) properties of this sample were investigated using RF-5301 PC spectrofluorophotometer. The structural features of samples were analyzed using X-ray diffraction (XRD), which showed that the samples were crystallized in a well-known structure. Morphological studies of the prepared phosphors were conducted using a scanning electron microscope (SEM). Photoluminescence characterization of Eu3+, Tb3+ co-doped phosphor shows a blue emission at λmax ∼ 450 nm and three peaks at 544 nm, 588 nm and 614 nm at the excitation of 377 nm. This phosphor was then coated on a solar cell for efficiency enhancement. The efficiency of the phosphor-coated solar cell was measured under artificial radiation via an LED solar simulator and also under direct sunlight. The enhancement in the actual intensity of solar cells was observed to be 23.95% and 30.44% for artificial and direct solar illumination, respectively. The as-synthesized phosphor shows a broad emission spectrum in the visible region, this might make it a potential candidate for WLEDs and the reason behind solar cell efficiency enhancement. Hence this material being a broad spectral converter is suitable for next-generation solar cells.
1. Introduction
In recent times, rare-earth activated luminescent phosphors have become an essential theme of research because of their high application scope in solar cells, bio-imaging and solid-state lighting.1–5 Silicon-based solar cells have tremendous demand in the market due to their extensive light conversion efficiency, however, the expected efficiency is not received due to the spectrum mismatch observed between the silicon absorption region and actual solar radiations.6,7 The absorption of high or low energy incident photons, which causes thermalization is the foremost loss by which energy is exhausted in Si-solar cells. Using a down-converting or up-converting layer of phosphors on solar cells for the enhancement of efficiency these losses can be minimized. In this work, a propitious approach of solar spectrum conversion by the coating of down-converting phosphors for efficiency improvement in PV cells has been attempted. Down-conversion is the phenomenon involving the conversion of high-energy photons into low-energy photons. Down-conversion can be proficient through the use of host lattice or ions, which can lead to quantum efficiency greater than unity.8–10 When an analogous process transpires in a material with a quantum efficiency smaller than unity the process is said to be downshifting.11–13 A study in lanthanides for down-conversion started with single-ion doped-fluorides such as Pr3+,14 Tm3+,15 Er3+,16 Gd3+,17 Eu3+
18 capable of cascade emission. In some lanthanide ions, d–f optical transitions are present and exhibit broader emission spectra than the ions with f optical transitions.19 Energy transfer from a lanthanide ion to another ion takes place using different methods, including (i) relocation of electrons and holes; (ii) migration of excitons; (iii) reverberation between atoms with adequate overlap integrals; and, (iv) re-absorption of photons emitted by another activator ion or sensitizer.20,21 The energy absorbed by a lanthanide ion can move around host lattice defects and then recombine non-radiatively affecting down-conversion performance.10 Consequently, luminescent host materials ought to be highly crystalline and have few lattice defects and impurities.22 Various lanthanides are used as co-doping agents for the proficient down-conversion occurrence to enhance the efficiency of the solar cell such as Mn2+/Yb3+,23 Tb3+/Yb3+,24 Tm3+/Yb3+,9 Pr3+/Yb3+,25 Nd3+–Yb3+,26 and Er3+/Yb3+.27 Out of these co-doping rare earth combinations, Eu3+–Tb3+ is found to be less reported for solar cell efficiency enhancement. Furthermore, the K2NaAlF6 host lattice was selected due to its high chemical, thermal stabilities and strong absorption in the NUV region.
Commercially available silicon-based solar cells are efficient solar energy converters, however, the efficiency improvement is in progress by taking into account device optimization, defect control, and surface modification.28 The major loss of radiation is considered mainly due to the thermalization process; hence, downshifting becomes the best solution for efficiency improvisation. This involves the conversion of high-energy photons from incident radiations into low-energy photons. Lanthanide-based rare earth elements are found to be more effective in the down shift. Le Donne et al. (2011)29 reported ethylene-vinyl-acetate layers doped with a single europium complex for the improvement of Si-based solar cell efficiency. This down shifter compound under the illumination of an air mass ratio of 1.5 enhances efficiency by 2.9%. The same author30 previously also reported the effect of downshifting on the crystalline silicon-based solar cell, where the bottom poly-vinyl-acetate layer doped with tris(dibenzoylmethane) (monophenanthroline) europium(III) and of a top poly-vinyl-acetate layer doped with tris[3(trifluoro methyl hydroxymethylene)-D-camphorate] europium(III). The encapsulating structure showed a 2.8% increase in efficiency. The main reason stated was the downshifting of photons below 400 nm. A. Tahhan et al. recently reported a coating of erbium and terbium-doped gadolinium oxysulfide phosphors encapsulated in ethyl vinyl-acetate binder on commercial single-junction silicon solar cells using blade screen printing technique. This results in an increase in the ultraviolet light conversion as well as electron transition capacity of the PV cell. The enhancement was about 0.54%.31 On similar lines, Ho (2017) employed the spin-on film technique for coating Eu-doped silicate phosphor. The increase in the conversion efficiency was 9.5% using small Eu-doped silicate particles.32
Yet another reason, which makes surface modification an important feature is that commercially available solar cells posse high surface reflection characteristics. 30% of incident radiations are reflected due to this property, which eventually affects the efficiency of the solar cell. Hence, attempts were made to improvise its efficiency using coating materials on the surface of the solar cell. Solar cell efficiency enhancement via coating was reported by Ashok Kumar et al.,33 where they have studied coating of various anti-reflection nanomaterials out of which CNT–TiO2–SiO2 composite showed an increase in the performance by 31.25% in comparison to the bare solar cell. The method of coating includes the removal of polymer coating by commercially available solar cells. Sagar et al. reported surface-modified silicon solar cells with the deposition of amorphous tantalum oxide (Ta2O5) and crystalline titanium oxide (TiO2). Improvement in the efficiency observed was about 1.54% in the antireflection-coated cells.34
Hence, in this work, we deal with the study of Eu3+, Tb3+ activated/co-activated K2NaAlF6 host lattice, which was synthesized by a wet chemical reaction. The prepared phosphors were then characterized using different characterization techniques such as XRD and SEM to confirm their crystallinity. The photoluminescence phenomenon in all samples showed broad emission in the blue region and sharp peaks in the green and red region. The as-synthesized phosphor was coated on commercially available silicon solar cells effectively and I–V characteristics of the phosphor-coated solar cell were evaluated using a solar simulator with an air mass ratio of 1.5 at 40 °C and also under direct sunlight. The efficiency of the coated solar cell was enhanced by 23.95% under artificial illumination and by 30.44% under direct solar radiation. These envisage the use of synthesized phosphor as an effective candidate for solid-state lighting and also for solar cell efficiency enhancement.
2. Experimental and characterization
A series of K2NaAlF6:Eu3+,Tb3+ activated/co-activated luminescent down-conversion phosphors were synthesized by a wet chemical reaction.35 Precursors used during synthesis are as follows; potassium nitrate (99% extra pure), sodium nitrate (99% extra pure), aluminium nitrate nanohydrate (98% extra pure), ammonium fluoride (95% extra pure), europium oxide 99.9% AR grade, dysprosium oxide 99.9% AR grade. All chemicals were obtained from Loba Chemie. All precursor chemicals were first dissolved in a separate beaker with constant stirring by keeping the temperature at 60 °C. After complete dissolution, all the materials were mixed in one beaker under constant stirring and temperature. It is to be noted that all precursors except ammonium fluoride were dissolved in Borosil beakers. Ammonium fluoride was dissolved in a Teflon beaker as fluoride reacts with glass. Stirring was continued by keeping the temperature constant until precipitate was formed. The precipitate formed was separated from the solution. This wet precipitate was then dried in a hot air oven for 12 hours at 80 °C. After that, it was allowed to cool at room temperature. Samples were collected and crushed for 5 min and again kept in a muffle furnace for annealing at 150 °C for 12 hours. These samples were then cooled at room temperature and used for further characterization.
The phase and crystalline nature of the powered sample were confirmed using a Rigaku mini flex d 600 X-ray diffractometer with Cu Kα radiation (λ = 0.154056 nm) operated at 40 kV, 15 mA. The XRD pattern was recorded in the range of 10–90° with the step size 0.02°. The morphology of the prepared phosphor was confirmed using the SEM, JSM-6360LV instrument (JEOL, USA). The surface morphology of the coated solar cell surface was investigated using a Leica dissecting microscope. A 150 W xenon lamp as the excitation source was used to perform the photoluminescence (PL) measurements. These characterizations were performed at room temperature. I–V characteristics of the blank and coated solar cell were evaluated using a Jetspin solar simulator and under direct sunlight at 40 °C for comparison.
3. Results and discussion
3.1 XRD analysis and crystal structure
Fig. 1 shows the XRD pattern of K2NaAlF6 phosphors, which is in good agreement with standard ICSD card no. 98-000-6027. XRD profiles showed that all the peak positions were in good agreement with the standard data. No impurity was found in the XRD pattern and it was well-matched with standard data. Therefore, it was confirmed that K2NaAlF6 phosphors were synthesized successfully. The XRD pattern of Eu3+, Tb3+ co-activated K2NaAlF6 phosphor can be tabulated to the Fm
m space group with space group number 225 of the cubic phase of the K2NaAlF6 crystal.
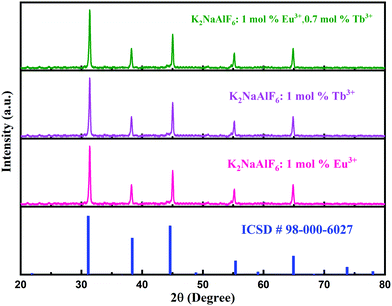 |
| Fig. 1 XRD patterns of Eu3+, Tb3+ activated/co-activated K2NaAlF6 well-matched with standard ICSD data. | |
To get a scrupulous sign of the changes taking place in the cell parameters of the K2NaAlF6 phosphor with the doping of the Eu3+, Tb3+ ions, structural refinements of the optimum sample was performed using the Rietveld method. Fig. 2 demonstrates the X-ray diffraction pattern for the K2NaAlF6:1 mol%Eu3+,0.7 mol%Tb3+ phosphor obtained from the Rietveld refinement.36,37 The refinement process was implemented using the Full-Proof suite using the Pseudo-Voigt peak profile function.38,39 The accuracy factors and the cell parameters resulting from the structural refinements are shown in Table S1 in the ESI.†
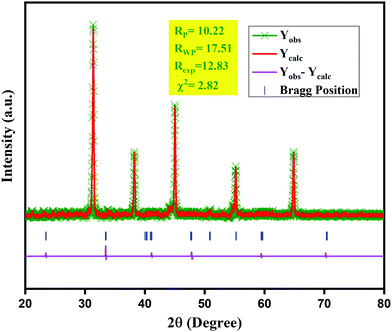 |
| Fig. 2 Rietveld refinement of 1 mol%Eu3+, 1 mol%Tb3+ activated K2NaAlF6 phosphor. | |
Fig. 3 shows crystal structures of the K2NaAlF6 host lattices. K2NaAlF6:1 mol%Eu3+,0.7 mol%Tb3+ crystallizes into a cubic phase with the Fm
m space group and its lattice constants are, a = b = c = 8.1154 Å, α = β = γ = 90°. The volume of the cubic unit cell of K2NaAlF6:1 mol%Eu3+,0.7 mol%Tb3+ is 535.11 Å3. No impurity peaks were found, representing that the incorporation of rare-earth ions has no noteworthy cause on the phase of K2NaAlF6 because of the little disparity of valence states and ionic radius of Al3+ and Eu3+, Tb3+ ions. This compound is associated with a cubic structure, in which, each Al3+ ion or Na+ ion is octahedrally encircled by six F− ions to form two different kinds of octahedrons. Each K+ ion is bounded by 12 nearest neighboring F− ions to construct an almost regular polyhedron. In contrast to Na+, Al3+ not only shares the same valence states but also have similar ionic radii with Eu3+, Tb3+ ions, indicating that Eu3+, Tb3+ have higher probability to form [Eu/TbF6]2− octahedron by replacing Al3+ and capitulate its typical white light without changing the crystal structure of K2NaAlF6.40
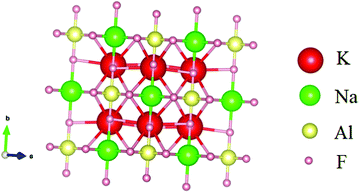 |
| Fig. 3 Crystal structure of K2NaAlF6:1 mol%Eu3+,1 mol%Tb3+ phosphor. | |
3.2 SEM
Fig. 4 shows SEM micrographs of K2NaAlF6 phosphors synthesized using the wet chemical reaction method. As observed in Fig. 4(a), the sample shows the smooth fluffy nature of the phosphors having some voids in it. Fig. 4(b) shows that samples have some rod-shaped particles on the surface having a particle size in the nanometer range. The smallest size of the rod-shaped particle was 146.5 nm recorded in the micrograph with a magnification of 1 μm.
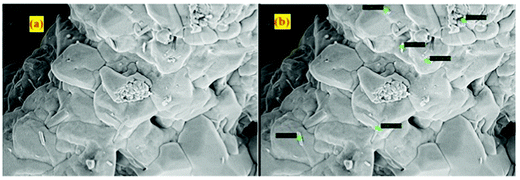 |
| Fig. 4 SEM micrograph of K2NaAlF6 phosphor. | |
This fleecy nature and irregular particle size of the phosphors were observed in the phosphor synthesized via wet chemical synthesis. Voids created in the phosphors observed in micrographs are due to improper allocation of temperature and removal of excess gases during the annealing process. The prepared phosphor shows sintered structures in which accurate particles could hardly be recognizable.41 All these physical manifestations were beneficial in consideration of their application as phosphor powders for cell coating, which ultimately results in the efficiency enhancement of solar cells.
3.3 Surface morphology of coated cell
The surface morphology of the Eu3+–Tb3+ activated/co-activated K2NaAlF6 phosphor coated on the solar cell surface was investigated using a Leica dissecting microscope and displayed in Fig. 5. As observed in Fig. S1 (ESI†), the surface of the coated solar cell shows uniform coating all over the surface of a solar cell, however, it also displays coating with fine cracks. These fine cracks and voids may be developed during the drying of the coated cell at room temperature. These cracks created in the solid phosphor coating are due to improper allocation of temperature during the annealing of phosphor.
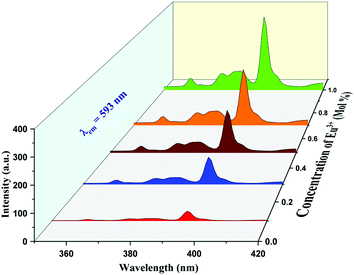 |
| Fig. 5 PL excitation spectrum of K2NaAlF6:x mol%Eu3+ phosphor under emission of 593 nm (x = 0.1, 0.3, 0.5, 0.7, 1 mol%). | |
3.4 Photoluminescence studies
Eu3+, Tb3+ ion-activated materials have technical advantages and numerous applications because of their good spectroscopic properties and ability to fuse rare earth particles into various host materials. These materials have properties such as high quantum yield, fast fluorescence decay, high thermal stability and favorable emission wavelength, which makes them fruitful for utilization in luminescent studies. Thus, based on excellent luminescence properties, Eu3+, Tb3+ activated inorganic phosphors are applied in solid-state lighting devices. Fig. 5 shows the excitation spectrum of Eu3+-activated K2NaAlF6 phosphor under the emission wavelength of 593 nm shows four excitation peaks at 361, 374, 382 and 394 nm due to 4f–4f transition and harmonic of monitoring wavelength.42,43
The PL emission spectra obtained for the following phosphor at the excitation of 361, 374, 382 and 394 nm are displayed in Fig. 6(a), (b), (c) and (d), respectively, shows broad intense peaks of 445 nm ranging from 410 nm to 575 nm and two narrow emissions were observed at 593 nm and 615 nm. The broad peak observed at 445 nm is due to 4f6–5d1 → 4f7 transitions and narrow emissions at 593 nm and 613 nm are due to 5D0 → 7F1 and 5D0 → 7F2 transitions, respectively.44 The most intense emission peak is obvious at 593 nm; another peak is observable at 613 nm.42,43 A similar profile can be identified in the emission spectra for different Eu3+ concentrations. Since the valence electrons of Eu3+ are shielded by the outer electrons in 5s and 5p orbitals, the f–f transitions of Eu3+ are weakly affected by ligand ions in the host lattice. As a result, the line shape of the emission did not change with the variation of Eu3+ concentration. The peak intensity was found to increase with increasing Eu3+ concentration. Although the nature of the emission peaks is similar for all the dopant concentrations, there is an increase in the peak intensity when the dopant concentration varies from x = 0.1 mol% to 1 mol%. The optimum peak intensity of emission is recognized at x = 1 mol%. The intensity considerably suffers from the symmetry in native environments around Eu3+ ions, as explained by Judd–Ofelt theory, the electrical dipole transition is allowed only if the Eu3+ particle occupies a site rather than having an association with the inversion center.45
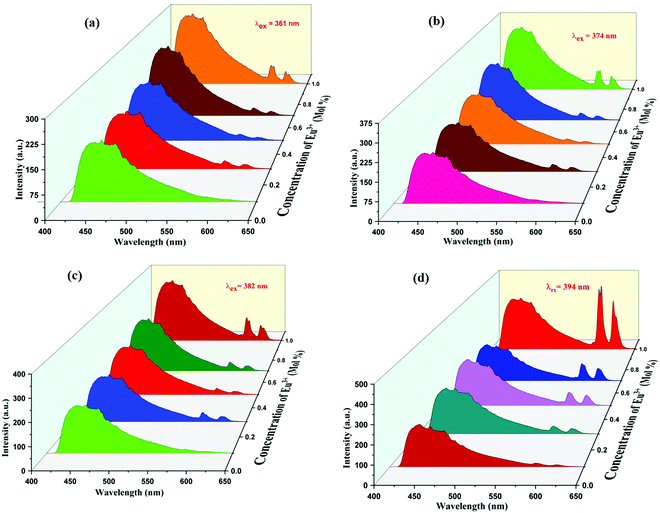 |
| Fig. 6 PL emission spectra of K2NaAlF6:x mol%Eu3+ phosphor under excitations of (a) 361 nm, (b) 374 nm, (c) 382 nm and (d) 394 nm, respectively (x = 0.1, 0.3, 0.5, 0.7, 1 mol%). | |
PL emission and excitation spectra were recorded for the same host lattice on doping Tb3+ ions. PL excitation spectra show four peaks in the NUV range having peak positions at 352 nm, 368 nm, and 377 nm. Peaks at 352 nm and 368 nm are due to 7F6 → 5L9 transitions while PL excitation spectra observed at 377 nm is arise due to 7F6 → 5D3 transition46 as displayed in Fig. 7(a). Peaks at 377 nm are optimum intense peaks hence we record PL emission spectra on these two wavelengths and it is observed that two strong emission peaks were observed at 452 nm and 545 nm with some small peaks at 452 nm and 488 nm, which are broad in nature. PL emission spectra observed at 452 and 488 nm are attributable to 5D4 → 5F6 and the one at 544 nm is due to 5D4 → 5F5 transition47–49 as displayed in Fig. 7(b).
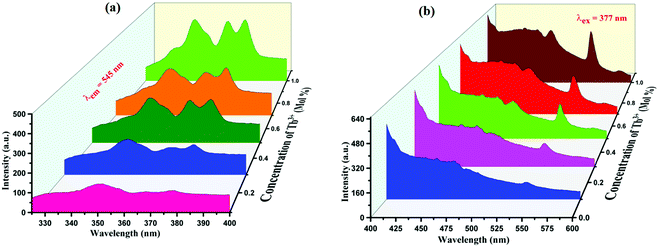 |
| Fig. 7 (a) PL excitation spectra of K2NaAlF6:x mol%Tb3+ phosphor under the emission of 545 nm. (b) PL emission spectra of K2NaAlF6:x mol%Tb3+ phosphor under excitation of 377 nm (x = 0.1, 0.3, 0.5, 0.7, 1 mol%). | |
As there are three kinds of spectral overlap for the confirmation of energy transfer in the photoluminescence, namely, the excitation–excitation overlap of the donor and acceptor, emission–emission overlap of the donor and acceptor and emission overlap of the donor to excitation of the acceptor.50,51 Out of all these three criteria, an excitation–excitation criterion has been satisfied in the present work. The spectral overlap between the excitations of two activators is necessary for the energy transfer to take place.52,53 The first activator accepts the incoming photon and emits further, which is again absorbed by the second activator ion to re-emit in the visible region. Here, in the present case, Eu3+ acts as the first activator, whereas Tb3+ acts as the second activator. The Eu3+ ion excitation shows peaks at 361, 374, 382 and 394 nm, while the excitation of Tb3+ ions showed peaks at 351 nm, 368 nm and 377 nm, the overlapping of these excitations are at two peak positions at 374 nm and 377 nm for Eu3+ and Tb3+, respectively. These overlap of excitations are displayed in Fig. 8. Hence, Eu3+ remained constant while Tb3+ ions got varied for a series and the energy got transferred from Eu3+ ions to Tb3+ ions.
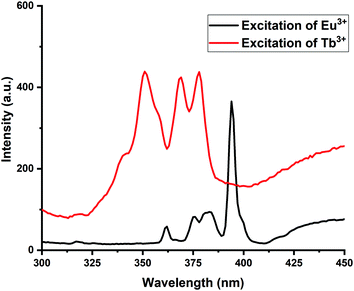 |
| Fig. 8 Overlapping of excitation spectra of Eu3+ and Tb3+ ions. | |
PL emission spectra of Eu3+, Tb3+ co-activated K2NaAlF6 phosphors are displayed in Fig. 9(a). On exciting K2NaAlF6:1 mol%Eu3+,x mol%Tb3+ phosphor at excitation of 377 nm, the observed broad emission peak was centered at 455 nm and several more peaks were seen at 544 nm, 588 nm and 614 nm due to the same transitions for Tb3+ and Eu3+, as mentioned above. Maximum intense peaks were observed at a concentration of 0.7 mol% of Tb3+ ion and beyond that sample get quenched. The concentration quenching in Eu3+, Tb3+ co-activated K2NaAlF6 phosphors are displayed in Fig. 9(b).
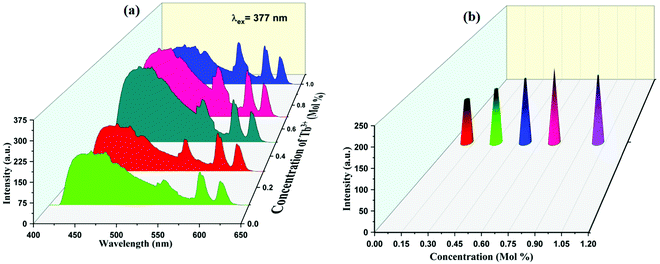 |
| Fig. 9 (a) PL emission spectra of K2NaAlF6:1 mol%Eu3+,x mol%Tb3+ phosphor under excitation of 377 nm (x = 0.1, 0.3, 0.5, 0.7, 1 mol%). (b) Concentration quenching in Eu3+, Tb3+ co-activated K2NaAlF6 phosphors. | |
Energy levels of the probable energy transfer mechanism between Eu3+ and Tb3+ are shown in Fig. 10. As shown in Fig. 10, Eu3+ electrons in the 7F0 ground state energy level can be excited using UV light and they jump to the 5L6 excited state level. These electrons are then down to 5D0 transition state because of multi-photon relaxation. As it is well known, the excited states are unstable, and Eu3+ can exhibit red light when the electrons jump back from 5D0 to 7FJ (J = 0, 1, 2, 3 and 4) with non-radiative processes.
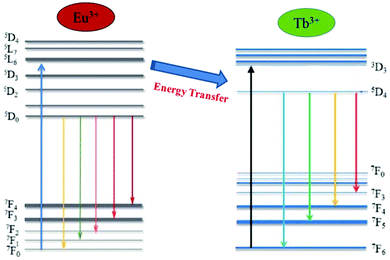 |
| Fig. 10 Energy-level diagram of Eu3+ to Tb3+ energy transfer. | |
PL intensity of Tb3+ was enhanced with increasing concentration of Tb3+ ions. The energy transfer mechanism from Eu3+ to Tb3+ ions was established by the above occurrence in the emission intensities of Eu3+ and Tb3+. The energy transfer efficiency (ηT) from Eu3+ to Tb3+ can be deliberated calculated using the following eqn (1);54
|  | (1) |
where
IS and
IS0 are the luminescence intensities of Eu
3+ with and without the presence of Tb
3+, correspondingly. The
ηT from Eu
3+ to Tb
3+ in K
2NaAlF
6 phosphors was calculated as a function of the Tb
3+ concentration, as shown in
Fig. 11. The value of η
T was determined and it is enhanced progressively with an increase in the Tb
3+ content. When the Tb
3+ ion concentration was increased to 0.7 mol%, the transfer efficiency improved to 76%, signifying an efficient energy transfer from Eu
3+ to Tb
3+.
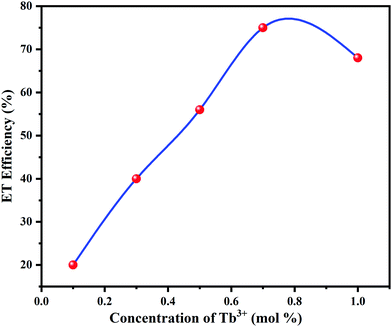 |
| Fig. 11 Energy transfer efficiency between Eu3+ to Tb3+ ions in K2NaAlF6 phosphors. | |
The critical transfer distance (Rc) is the reason behind the concentration quenching process, which is the minimum standard distance between the nearest activator ions at an optimum concentration Xc. Usually, there are two diverse characteristics that result in the resonant energy transfer among the host and an activator ion. This energy transfer may take place owing to an exchange interaction or an electric multipole–multipole interaction. The exchange interaction occurs because the value of Rc is less than 5 Å, beyond this value electric multipole–multipole interaction is responsible for the concentration quenching process. The critical distance can be calculated using the Blasse formula.55 The critical distance (Rc) can be given as;
| 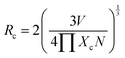 | (2) |
Here V is the volume of the unit cell, Xc is the concentration of Tb3+ ions, and N is the number of available sites for the activator in the unit cell. The volume of the unit cell, determined from the refined structure, is 535.11 Å3 and N = 4. In the present study, the value of Xc is 0.7. Therefore, the value of Rc was found to be 7.1477 Å and this eliminates the chances of the exchange interaction between the hosts and rare-earth ions. This shows that the concentration quenching occurs due to nonradiative transition between host lattice and rare earth ions take place through electric multipolar–multipolar interaction.
The concentration of the activator is significantly high, the luminescence intensity I and the activator concentration x are related to the equation,
| 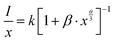 | (3) |
This equation is fairly accurate too,
| 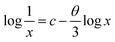 | (4) |
where,
k,
β and
c are constants for the same excitation circumstance for a given host matrix. As per the van Uitert theory, the (
θ) value indicates the electric multipolar character.
56 The relation between log(
x) and log(
I/
x) is displayed in
Fig. 12. Since the interaction of ions after the concentration quenching is further complex only three points were utilized for plotting.
57 As displayed in the graph, data was established to fit a straight line with a slope. Value of the slope,
i.e.,
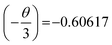
, therefore
θ = 1.8185, which is nearly equal to 3 that means exchange interaction is the dominant interaction responsible for the concentration quenching in rare earth emission for K
2NaAlF
6:1 mol%Eu
3+,
x mol%Tb
3+ (
x = 0.1 ≤
x ≤ 1.0) phosphors.
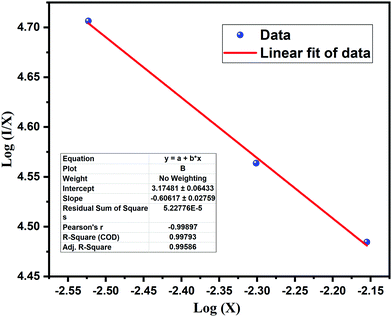 |
| Fig. 12 A linear plot of log(X) vs. log(I/X) for K2NaAlF6:1 mol%Eu3+,X mol%Tb3+ phosphors. | |
3.5 CIE chromaticity
CIE chromaticity of Eu3+–Tb3+ co-activated K2NaAlF6 phosphor's spectrum under 377 nm light excitation was calculated using a CIE 1931 color matching function. Fig. 13 shows CIE chromaticity diagram of Eu3+–Tb3+ co-activated K2NaAlF6 phosphor. In order to understand the variation in PL intensity with the concentration of Eu3+–Tb3+ co-activated K2NaAlF6 host, a series was synthesized. The blue emission intensity increases with the increase in Tb3+ ion concentration. Intensity is enhanced up to 0.7 mol%Tb3+ ion concentration, beyond this, the PL emission intensity decreases with the increase in Tb3+ concentration due to concentration quenching phenomena.58,59 The CIE coordinates of each concentration for Eu3+–Tb3+ co-activated K2NaAlF6 phosphor are displayed in Table 1 to show the change in emission intensity more intuitively. Chromaticity coordinates depict that the color is tuned from whitish blue to white as color coordinates shift towards the white region.
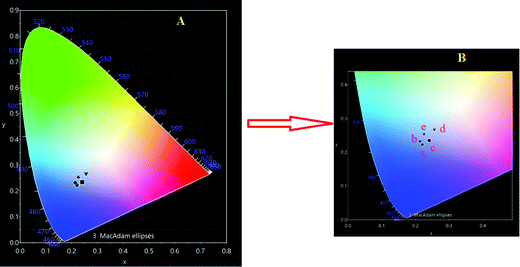 |
| Fig. 13 CIE chromaticity diagram of Eu3+–Tb3+ co-activated K2NaAlF6 phosphor. | |
Table 1 Overview of the CIE color coordinates for Eu3+–Tb3+ co-activated K2NaAlF6 phosphor
S. no. |
Concentration of Eu3+–Tb3+ (mol%) |
Symbol on CIE diagram |
CIE color coordinates |
1 |
0.1 |
a |
(0.2204, 0.2227) |
2 |
0.3 |
b |
(0.2138, 0.2327) |
3 |
0.5 |
c |
(0.2414, 0.2346) |
4 |
0.7 |
d |
(0.2560, 0.2680) |
5 |
1 |
e |
(0.2255, 0.2680) |
3.6 Comparison of PL emission spectra of Eu3+–Tb3+ co-activated K2NaAlF6 phosphor coated on solar cell
Fig. 14(a) shows PL emission spectra of (a) optimized K2NaAlF6:1%Eu3+,0.7%Tb3+ phosphor and Fig. 14(b) shows PL emission spectra of K2NaAlF6:1%Eu3+,0.7%Tb3+ phosphor coated on the solar cell. If the two spectra are inspected closely it is observed that the peaks of as-synthesized K2NaAlF6:1%Eu3+,0.7%Tb3+ phosphor around 455 nm, 544 nm, 588 nm and 614 nm are retained in PL emission spectra of the coated phosphor. This indicates that the UV conversion efficiency has been retained by the coated phosphor. Peak sharpening is also observed in the coated sample this may be the reason behind the enhancement in the efficiency of the solar cell.
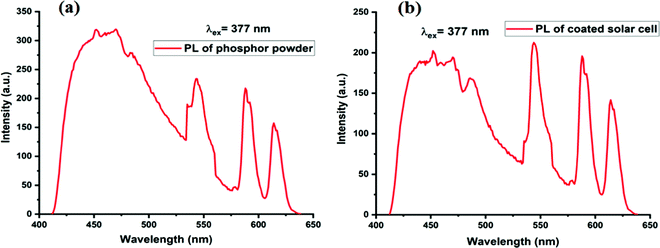 |
| Fig. 14 PL emission spectra of (a) optimized K2NaAlF6:1%Eu3+,0.7%Tb3+ phosphor. (b) PL emission spectra of K2NaAlF6:1%Eu3+,0.7%Tb3+ phosphor coated on solar cell. | |
3.7
I–V characteristics
Enhancement in the photovoltaic efficiency was determined by the solar simulator as well as in direct sunlight at 40 °C. The direct sunlight temperature was measured by a thermometer. The photovoltaic cell was coated by the doctor blade method.60,61 The blank solar cell and solar cell after coating of Eu3+–Tb3+ co-activated K2NaAlF6 down-conversion phosphor are displayed in Fig. 15. The enhanced efficiency of the solar cell was determined by comparing the I–V characteristic of coated solar cell with the blank one in both conditions. It was observed that the photovoltaic efficiency was enhanced by 23.95% under the solar simulator and 30.44% under direct sunlight. The proposed enhancements in the efficiencies were determined by the given formula;62 |  | (5) |
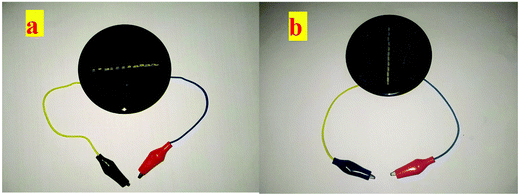 |
| Fig. 15 (a) Silicon solar cell without coating, (b) silicon solar cell coated with Eu3+–Tb3+ co-activated K2NaAlF6 down-conversion phosphor. | |
Fig. S2 and S3 in the ESI† demonstrate the plot of I–V characteristics under solar simulator and under direct sunlight correspondingly.
3.8 Spectrum response
The downshifting layer of the proposed phosphor was coated on the solar cell, which absorbs high energy and transfers it to a low energy range. This part of the spectrum response reasonably and does not absorb the energy, which results in thermal losses.63,64 These thermal losses in the solar cell have been controlled by the downshifting layer coated on the solar cell and act as a spectral converter that converts high energy photons into low energy photons helping the solar cell in the generation of the electron–hole pair. In addition, the K2NaAlF6:1%Eu3+,xTb3+ spectral converter can also diminish the reflection losses caused by its renowned antireflection coating. Fig. 16 demonstrates the spectral converter for K2NaAlF6:1%Eu3+,0.7%Tb3+ phosphor for efficiency enhancement of the solar cell. Hence it was concluded that the proposed phosphor can act as a spectral converter in future solar cell technology.
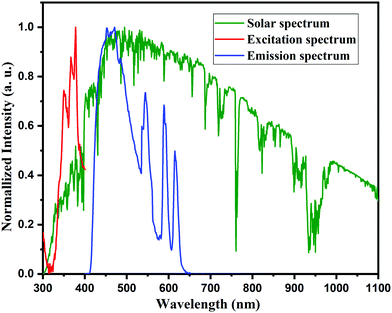 |
| Fig. 16 Excitation and emission spectra of K2NaAlF6:1%Eu3+,0.7%Tb3+ phosphor used in down conversion compared with the solar spectrum for air mass 1.5. | |
4. Possible mechanism
Fig. 17 depicts the possible mechanism for enhancement in the efficiency of the commercially available solar cells after surface coating with synthesized phosphor via the doctor's blade method. Eu3+–Tb3+ activated/co-activated K2NaAlF6 phosphor being a UV active compound takes up the radiation around 377 nm and has conversion emissions in the visible range covering the RGB region. The phosphor layer is the immediate layer above the Si-based solar cell, so, the emitted radiations are quickly taken up by the single-junction Si and result in the ultraviolet region energy conversion. This in turn increases the electron transfer capacity of the solar cell.
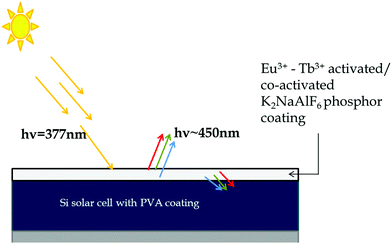 |
| Fig. 17 Possible mechanism for efficiency improvement shown by commercial solar cell coated with Eu3+–Tb3+ activated/co-activated K2NaAlF6 phosphor. | |
5. Conclusion
A series of Eu3+–Tb3+ activated/co-activated K2NaAlF6 phosphor was synthesized by a simple combustion method using urea as a fuel. The proposed phosphor was characterized using several characterization techniques. The crystalline nature of the proposed phosphor was characterized using XRD, which was then compared with the standard ICSD card no. 98-005-0292. XRD profile shows that all peak positions are in good agreement with the standard data. The PL emission spectrum of Eu3+–Tb3+ co-activated K2NaAlF6 phosphor shows a broad emission spectrum in the visible range from 400 nm to 650 nm, which covers all RGB regions and gives a white light emission. PL emission spectrum of the as-synthesized Eu3+–Tb3+ co-activated K2NaAlF6 phosphor coated on the solar cell shows retention in the PL intensity peaks as that of the bare phosphor. CIE chromaticity of the proposed phosphors shows white light, which confirms that the Eu3+–Tb3+ co-activated K2NaAlF6 phosphor is a suitable candidate for the white light emission. Moreover, Eu3+–Tb3+ co-activated K2NaAlF6 phosphor was coated on solar cells and tested for IV characteristics. Results show enhancement in its efficiency by 30.44% under direct sunlight and about 23.95% under solar simulator (AM1.5). Therefore with all these results, we can conclude that Eu3+–Tb3+ co-activated K2NaAlF6 phosphor is a potential candidate for w-LEDs, solar cell efficiency enhancement, and also a broad spectral converter for next-generation solar cells.
Conflicts of interest
There are no conflicts to declare.
Acknowledgements
One of the authors, SJD, is thankful to the Department of Science and Technology (DST), India (Nano Mission) (Project Ref. No. DST/NM/NS/2018/38(G), dated 16/01/2019 for financial assistance and R. T. M. Nagpur University, Nagpur for constant encouragement.
References
- N. Hamon, A. Roux, M. Beyler, J. C. Mulatier, C. Andraud, C. Nguyen, M. Maynadier, N. Bettache, A. Duperray, A. Grichine, S. Brasselet, M. Gary-Bobo, O. Maury and R. Tripier, J. Am. Chem. Soc., 2020, 142, 10184–10197 CrossRef CAS.
- R. Cao, W. Wang, J. Zhang, S. Jiang, Z. Chen, W. Li and X. Yu, J. Alloys Compd., 2017, 704, 124–130 CrossRef CAS.
- Y. Li, Y. Sun and Y. Zhang, Renewable Energy, 2020, 160, 127–135 CrossRef CAS.
- M. Rafiee, S. Chandra, H. Ahmed and S. J. McCormack, Opt. Mater., 2019, 91, 212–227 CrossRef CAS.
- K. Li and R. Van Deun, Phys. Chem. Chem. Phys., 2019, 21, 4746–4754 RSC.
- T. G. Allen, J. Bullock, X. Yang, A. Javey and S. De Wolf, Nat. Energy, 2019, 4, 914–928 CrossRef CAS.
- A. Padhy, B. Vishal, P. Verma, G. Dwivedi and A. K. Behura, Mater. Today Proc., 2021, 38, 56–62 CrossRef CAS.
- M. J. Crane, D. M. Kroupa and D. R. Gamelin, Energy Environ. Sci., 2019, 12, 2486–2495 RSC.
- W. Chang, L. Li, M. Dou, Y. Yan, S. Jiang, Y. Pan, M. Cui, Z. Wu and X. Zhou, Mater. Res. Bull., 2019, 112, 109–114 CrossRef CAS.
- C. S. Erickson, M. J. Crane, T. J. Milstein and D. R. Gamelin, J. Phys. Chem. C, 2019, 123, 12474–12484 CrossRef CAS.
- A. Solodovnyk, C. Kick, A. Osvet, H.-J. Egelhaaf, E. Stern, M. Batentschuk, K. Forberich and C. J. Brabec, Phys., Simul., Photon. Eng. Photovolt. Devices V, 2016, 9743, 97430G Search PubMed.
- S. P. Bremner, M. Y. Levy and C. B. Honsberg, Sol. Cells, 2008, 225–233 Search PubMed.
- Y. H. Ghymn, K. Jung, M. Shin and H. Ko, Nanoscale, 2015, 7, 18642–18650 RSC.
- L. Aarts, B. van der Ende, M. F. Reid and A. Meijerink, Spectrosc. Lett., 2010, 43, 373–381 CrossRef CAS.
- J. C. Boyer, F. Vetrone, L. A. Cuccia and J. A. Capobianco, J. Am. Chem. Soc., 2006, 128, 7444–7445 CrossRef CAS.
- J. W. Stouwdam and F. C. J. M. Van Veggel, Nano Lett., 2002, 2, 733–737 CrossRef CAS.
- G. Chen, C. Yang and P. N. Prasad, Acc. Chem. Res., 2013, 46, 1474–1486 CrossRef CAS PubMed.
- R. T. Wegh, H. Donker, K. D. Oskam and A. Meijerink, J. Lumin., 1999, 82, 93–104 CrossRef CAS.
- H. Hafez, M. Saif and M. S. A. Abdel-Mottaleb, J. Power Sources, 2011, 196, 5792–5796 CrossRef CAS.
- A. Shalav, B. S. Richards and M. A. Green, Sol. Energy Mater. Sol. Cells, 2007, 91, 829–842 CrossRef CAS.
- W. J. Ho, G. Y. Li, J. J. Liu, Z. X. Lin, B. J. You and C. H. Ho, Appl. Surf. Sci., 2018, 436, 927–933 CrossRef CAS.
- H. Liu, D. Moronta, L. Li, S. Yue and S. S. Wong, Phys. Chem. Chem. Phys., 2018, 20, 10086–10099 RSC.
- Y. Yan, Z. Chen, X. Jia and S. Li, Opt. Mater., 2018, 75, 465–470 CrossRef CAS.
- F. Enrichi, C. Armellini, S. Belmokhtar, A. Bouajaj, A. Chiappini, M. Ferrari, A. Quandt, G. C. Righini, A. Vomiero and L. Zur, J. Lumin., 2018, 193, 44–50 CrossRef CAS.
- W. B. Dai, Y. F. Lei, J. Zhou, M. Xu, L. L. Chu, L. Li, P. Zhao and Z. H. Zhang, J. Alloys Compd., 2017, 726, 230–239 CrossRef CAS.
- R. Zhou, Y. Kou, X. Wei, C. Duan, Y. Chen and M. Yin, Appl. Phys. B: Lasers Opt., 2012, 107, 483–487 CrossRef CAS.
- M. S. Morassaei, A. Salehabadi, A. Akbari, S. H. Tavassoli and M. Salavati-Niasari, J. Alloys Compd., 2018, 769, 732–739 CrossRef CAS.
- S. Binetti, M. Acciarri, A. Le Donne, M. Morgano and Y. Jestin, Int. J. Photoenergy, 2013, 249502 Search PubMed.
- A. Le Donne, M. Dilda, M. Crippa, M. Acciarri and S. Binetti, Opt. Mater., 2011, 33, 1012–1014 CrossRef CAS.
- S. Marchionna, S. Binetti, A. Le Donne, M. Acciarri and D. Narducci, Prog. Photovoltaics, 2009, 20, 519–525 Search PubMed.
- J. Sarkar and S. Bhattacharyya, Arch. Thermodyn., 2012, 33, 23–40 CAS.
- W. J. Ho, Y. J. Deng, J. J. Liu, S. K. Feng and J. C. Lin, Materials, 2017, 10, 21 CrossRef.
- B. Ashok Kumar, G. Sivasankar, B. Sangeeth Kumar, T. Sundarapandy and M. Kottaisamy, Mater. Today Proc., 2018, 5, 1759–1765 CrossRef CAS.
- R. Sagar and A. Rao, Appl. Phys. A: Mater. Sci. Process., 2019, 125, 1–8 CrossRef.
- N. Baig, A. R. Kadam, K. Dubey, N. S. Dhoble and S. J. Dhoble, Radiat. Eff. Defects Solids, 2021, 176(5–6), 493–507 CrossRef CAS.
- V. Singh, A. R. Kadam and S. J. Dhoble, Optik, 2021, 243, 167437 CrossRef CAS.
- V. Singh, A. R. Kadam, S. J. Dhoble and H. Jeong, Optik, 2021, 243, 167396 CrossRef CAS.
- K. K. Gupta, R. M. Kadam, N. S. Dhoble, S. P. Lochab, V. Singh and S. J. Dhoble, J. Alloys Compd., 2016, 688, 982–993 CrossRef CAS.
- A. R. Kadam, G. C. Mishra and S. J. Dhoble, J. Mol. Struct., 2021, 1225, 129129 CrossRef CAS.
- X. Yi, R. Li, H. Zhu, J. Gao, W. You, Z. Gong, W. Guo and X. Chen, J. Mater. Chem. C, 2018, 6, 2069–2076 RSC.
- M. S. Ahmad, N. A. Rahim and A. K. Pandey, Optik, 2018, 157, 134–140 CrossRef CAS.
- A. R. Kadam and S. J. Dhoble, Luminescence, 2019, 34, 846–853 CrossRef CAS PubMed.
- A. R. Kadam, G.
C. Mishra, S. J. Dhoble and R. S. Yadav, Ceram. Int., 2020, 46(3), 3264–3274 CrossRef CAS.
- A. R. Kadam, R. L. Kohale, G. C. Mishra and S. J. Dhoble, New J. Chem., 2021, 45(16), 7285–7307 RSC.
- S. C. Lal, A. M. Aiswarya, K. S. Sibi and G. Subodh, J. Alloys Compd., 2019, 788, 1300–1308 CrossRef CAS.
- H. Liu, L. Mei, L. Liao, Y. Zhang, Q. Guo, T. Zhou, Y. Wang and L. Li, J. Alloys Compd., 2019, 770, 1237–1243 CrossRef CAS.
- J. Zhou and Z. Xia, J. Mater. Chem. C, 2015, 3, 7552–7560 RSC.
- Z. Yahiaoui, M. A. Hassairi, M. Dammak, E. Cavalli and F. Mezzadri, J. Lumin., 2018, 194, 96–101 CrossRef CAS.
- K. Dubey, A. R. Kadam, N. Baig, N. S. Dhoble and S. J. Dhoble, Radiat. Eff. Defects Solids, 2021, 176(5–6), 431–440 CrossRef CAS.
- K. Li and R. Van Deun, Dyes Pigm., 2019, 162, 990–997 CrossRef CAS.
- B. Li and X. Huang, Ceram. Int., 2018, 44, 4915–4923 CrossRef CAS.
- J. Xiong, Q. Meng and W. Sun, J. Rare Earths, 2016, 34, 251–258 CrossRef CAS.
- Z. Li, B. Zhong, Y. Cao, S. Zhang, Y. Lv, Z. Mu, Z. Hu and Y. Hu, J. Alloys Compd., 2019, 787, 672–682 CrossRef CAS.
- X. Fu, L. Fang, S. Niu and H. Zhang, J. Lumin., 2013, 142, 163–166 CrossRef CAS.
- G. B. Nair and S. J. Dhoble, J. Lumin., 2017, 192, 1157–1166 CrossRef CAS.
- W. Zhang, R. Zhang, S. Yang, R. Wang, L. Na and R. Hua, Mater. Res. Bull., 2020, 122, 110689 CrossRef CAS.
- J. Papan, D. Jovanović, M. Sekulić, E. Glais and M. D. Dramićanin, Powder Technol., 2019, 346, 150–159 CrossRef CAS.
- A. R. Kadam, G. C. Mishra, A. D. Deshmukh and S. J. Dhoble, J. Lumin., 2021, 229, 117676 CrossRef CAS.
- X. Huang, H. Guo, L. Sun, T. Sakthivel and Y. Wu, J. Alloys Compd., 2019, 787, 865–871 CrossRef CAS.
- Y. Deng, Q. Wang, Y. Yuan and J. Huang, Mater. Horiz., 2015, 2, 578–583 RSC.
- M. Karbassi, S. Baghshahi, N. Riahi- Noori and R. Siavash Moakhar, Ceram. Int., 2020, 46, 2325–2331 CrossRef CAS.
- A. R. Kadam and S. J. Dhoble, J. Alloys Compd., 2021, 884, 161138 CrossRef CAS.
- P. Liu, J. Liu, X. Zheng, H. Luo, X. Li, Z. Yao, X. Yu, X. Shi, B. Hou and Y. Xia, J. Mater. Chem. C, 2014, 2, 5769–5777 RSC.
- R. Lopez-Delgado, Y. Zhou, A. Zazueta-Raynaud, H. Zhao, J. E. Pelayo, A. Vomiero, M. E. Álvarez-Ramos, F. Rosei and A. Ayon, Sci. Rep., 2017, 7, 1–8 CrossRef CAS.
Footnote |
† Electronic supplementary information (ESI) available. See DOI: 10.1039/d1nj04836h |
|
This journal is © The Royal Society of Chemistry and the Centre National de la Recherche Scientifique 2022 |