DOI:
10.1039/D1NJ04761B
(Paper)
New J. Chem., 2022,
46, 263-275
Catalytic degradation of methylene blue using iron and nitrogen-containing carbon dots as Fenton-like catalysts†
Received
6th October 2021
, Accepted 10th November 2021
First published on 11th November 2021
Abstract
Wastewater pollution is an existing and serious environmental issue. One of the main wastewater pollution sources is from the dyestuff industries and its discharge poses a serious threat to the ecosystem due to its adverse effects on human and environmental health. Conventional wastewater treatments are limited in terms of effectively treating such non-biodegradable contaminants; thus, this work aimed to investigate the degradation of the commercial dye methylene blue (MB) via advanced oxidation processes (AOPs) using carbon dots as Fenton-like catalysts. Iron and nitrogen-containing carbon dots (Fe, N-CDs) were synthesised through a hydrothermal reaction and utilised as catalysts in the degradation of MB. Multiple characterisation techniques were employed to describe the dot properties including FTIR, XPS, TEM, ζ-potential, PL and UV-Vis analyses. Characterisation revealed the synthesis of 7 nm diameter, spherical negatively charged dots rich in iron and nitrogen surface functionalities. Methylene blue was degraded under mild conditions using an innovative green remediation technology which removed nearly 100% of the dye (20 mg L−1) under optimised conditions (at 50 °C, pH 8, H2O2 147 mM, and a catalyst concentration of 0.5 mg mL−1) in only 60 minutes. The active mechanism of the Fe, N-CDs was deduced suggesting the participation of free radicals such as hydroxyl radicals, singlet oxygens and holes. Importantly, the effluent toxicity assessed using Microtox® confirmed the generation of a non-toxic effluent. This investigation showed that using Fe, N-CDs is an effective way to degrade wastewater pollution whilst significantly minimising its toxicity.
1. Introduction
Global industrial wastewater poses a serious threat to the environment if left untreated. Worldwide, industry discharges an estimated 300–400 Mt of waste into water bodies every year which if inadequately treated before release, adversely affects both the ecosystem and human health.1 Among the discharged industrial pollutants, wastewater containing dyes from textile, leather and food industries is one of the main pollution sources.2–4 When coloured wastewater is discharged in aquatic systems, it causes a series of problems. Firstly, it reduces light penetration in the water bodies, affecting photosynthesis which consequently impacts flora and fauna health by reducing the dissolved oxygen levels.3 Secondly, synthetic dyes such as methylene blue are of great concern due to their toxic and adverse health effects.5 Moreover, dyes persist as environmental pollutants and in food chains leading to undesired biomagnification.2 Despite efforts to improve the efficiency of the wastewater systems to remove these contaminants, driven by increasingly stringent legislation, conventional wastewater treatments can be limited, costly and inefficient for such hazardous compounds. Consequently, efficient and cost-effective wastewater treatment technologies are required to remove these industrial pollutants from water before its safe release into the natural environment.
Over recent decades, advanced oxidation processes (AOPs) have been widely used to degrade and remediate organic pollutants from water and wastewater.6 Advanced oxidation processes generally use hydroxyl radicals (˙OH), powerful oxidants, to reduce organic compounds to harmless end products such as water and carbon dioxide. Among the different AOPs employed, the Fenton reaction represents a promising high performance water treatment, based on the formation of ˙OH by a catalytic reaction between Fe2+ and H2O2 which can fully oxidise organic compounds.7,8 However, there exist obvious drawbacks of the Fenton process including: (i) the requirement of a narrow acidic pH range (2.0–4.0) for activity, necessitating the need for neutralisation before discharge into the environment; (ii) the toxicity of the iron released during the process which can negatively impact aquatic living organisms and also lead to secondary pollution; and (iii) the need for an external source of energy such as high temperatures or light-dependent systems leading to an increase in the overall costs.9 To overcome these impractical application issues, Fenton-like reactions have emerged as an interesting alternative to the traditional Fenton process. Fenton-like reactions are started using a Fe3+ source or other transition metals, instead of the traditional Fenton reaction which is initiated using Fe2+.10–12 These reactions have demonstrated interesting and innovative possibilities for water treatment due to their efficient performance under mild conditions such as neutral pH, and for the possibility of using carbon based catalysts.12,13
Recently, carbon dots (CDs) have attracted attention due to their unique physicochemical properties that have been exploited in catalysis.14,15 As a new class of carbon nanomaterials, with low toxicity and rapid electron transfer features, carbon dots show significant potential for application in AOPs as Fenton-like catalysts for environmental remediation since they can be easily manufactured on a large scale.16 Over the past ten years, CDs have been investigated because of their superconductivity and high performance in photocatalysis when combined with metal oxides.17 Dyes such as methyl orange, p-nitrophenol and other complex molecules have been successfully degraded using this approach;18 however, as with Fenton reactions, some drawbacks limit their application including dependence on external energy and the generation of toxic sludges.
Only a few studies have investigated the catalytic properties of lone CDs in the presence of H2O2.19–21 These investigations showed the potential of CDs for application in catalysis due to their successful use at different pHs and temperatures which, along with their low toxicity overcome some of the main limitations of current Fenton-like catalysts. Due to the high number of oxygen-related groups on the surface of CDs, they can act as electron donors which can increase due to the presence of H2O2, a strong oxidant that can readily capture electrons.15 In addition, the introduction of metal ions is likely to induce new physicochemical properties and create more active sites on the surface of CDs which would greatly increase their catalytic activity.22 However, research to date has not yet determined whether CDs can act solely as Fenton-like catalysts in the degradation of environmental pollutants such as methylene blue (MB). In particular, the possibility that metal ion-containing carbon dots, with their enhanced catalytic properties could enhance degradation of pollutants is both intriguing and largely unexplored.
Evaluation of the ecotoxicity of effluents generated during environmental remediation is necessary as it may predict their adverse effects on the environment.23 However, this is often an overlooked aspect in remediation studies. Although some AOPs applied to the treatment of dyes showed high pollutant degradation performance; they generated highly toxic effluents which were due to the by-products formed during degradation.24–26 A recent study on the use of the Fenton process in dye degradation showed that degraded MB had a stronger toxic effect when compared to the untreated dye;26 however, for Fenton-like processes, in particular using CDs as catalysts, the ecotoxicity of the effluents remains unknown. Importantly, this may provide critical information for assessing and ultimately controlling the ecotoxicological risks of such an emergent technology. Thus, it is mandatory to evaluate such fundamental features in AOPs studies to avoid further environmental damage.
In previous work we developed ecofriendly CDs made of green material, which were able to efficiently decolourise hazardous cationic dyes at neutral pH, 22 °C, at low catalyst and H2O2 concentrations in only 60 minutes.27 Herein, we aimed to develop a one-step green synthesis of carbon dots using citric acid, urea and ferric citrate as precursors and investigated their degradation efficiency as Fenton-like catalysts for the first time on MB. Following characterisation to determine the key catalytic properties of the nanoparticles, the effects of temperature, initial pH, catalyst and H2O2 concentrations were studied and optimised using the Taguchi method. Moreover, to evaluate the ecotoxicity aspects of this approach and whether it has an impact on aquatic organisms, the effluents were assessed using a bioluminescence inhibitory assay with the marine bacteria Aliivibrio fischeri (Microtox®).
2. Materials and methods
2.1 Reagents
All chemicals and reagents used in this study were of analytical grade and used without further purification. The following chemicals were purchased from Sigma-Aldrich: methylene blue (MB), hydrogen peroxide (30% w/w), citric acid, urea, ferric citrate, isopropanol (IPA), dimethyl sulfoxide (DMSO), tert-butyl alcohol (TBA), sodium azide and ethylenediaminetetraacetic acid (EDTA). Ultrapure water (18.3 MΩ cm−1) was used in all the degradation experiments.
2.2 Hydrothermal synthesis of CDs
The CDs were synthesised via a green route using a hydrothermal approach. Citric acid (1.0 g), urea (0.5 g) and ferric citric (0.1 g, 0.5 g and 1.0 g) were used as precursors. The reagents were dissolved in ultrapure water (30 mL) and stirred until homogeneous. The solution was transferred to a poly(para-phenol)-lined (PPL) stainless-steel autoclave (50 mL) and heated at 230 °C for 4 h. After cooling to room temperature, the brownish CD suspension was centrifuged at 10
000 rpm for 15 min and the supernatant was filtered with a 0.22 μm membrane to remove larger particles. Finally, the obtained solution was freeze dried and kept at −20 °C for further use.
2.3 Physicochemical characterisation of samples
Photoluminescence (PL) spectra and ultraviolet visible (UV–vis) absorption spectra were analysed using CLARIOstar®, BMG LABTECH. Zeta potential measurements of CDs were performed using a Zetasizer Nano ZS (Malvern Panalytical), ZS series/Model Zen3600. Zeta potential was measured using M3-PALStechnology and Disposable Folded Capillary Cells DTS1070. Samples for transmission electron microscopy (TEM) were prepared by drop coating the samples on a carbon coated copper grid and analysed using a JEOL1010 TEM operated at 100 kV. Fourier-transformed infrared (FTIR) spectra were determined by FTIR spectroscopy (PerkinElmer Frontier). An average of 32 scans was collected for each measurement with a resolution of 4 cm−1 in the range of 4000–400 cm−1. A Thermo Scientific K-Alpha XPS was used and peak fitting was performed using the Thermo Scientific Avantage XPS software package. Background signal subtraction of each core peak region of each sample was carried out using a Shirley background model.
2.4 Experimental set up using the Taguchi method
The Taguchi method is a robust statistical method widely used to improve the quality of manufactured goods and design processes while identifying and optimising the dominant parameters with a minimum of experiments. In this study, an orthogonal array L16 of the Taguchi method of experimental design was used to determine the best conditions for MB dye removal. The L16 model consisted of four factors and four levels which resulted in sixteen experiments. The design of the experiments was performed using the Minitab 18 software package. The factors tested were temperature, pH, catalyst dose and H2O2 dose. Table S1 (ESI†) shows the factors and levels utilised in this study and Table S2 (ESI†) shows the combination of 16 experiments generated by the Taguchi method of experimental design.
The Taguchi method uses the signal-to-noise ratio (S/N) which measures how the response varies relative to the nominal or target value under different noise conditions. Noise factors cannot be controlled during production or product use but can be controlled during experimentation. In this study, we used the “larger is better” which follows the signal-to-noise ratio formula below:
S/N = −10 × log(Σ(1/Y2)/n) |
where
Y is the characteristic property, and
n is the replication number of the experiment (Minitab 18 software).
For this study, larger degradation of MB is better hence a higher signal-to-noise ratio (S/N) was chosen as the best result.
2.5 Catalytic degradation of MB
The catalytic activity of the as-prepared CDs was tested by catalytic degradation of MB in the presence of H2O2 under several conditions as detailed in Table S2 (ESI†). All experiments were conducted in an orbital shaker at 120 rpm, under natural light, in triplicate and respective controls were used. Typically, 10 mL of MB solutions at 20 mg L−1 were prepared at different pH values (4.0, 6.0, 8.0 and 10.0) using HCl and NaOH solutions to adjust the pH accordingly. The CDs were dispersed in the solutions and shaken in the dark for 1 hour to achieve adsorption equilibrium. Hydrogen peroxide (H2O2) was added to start the degradation reaction and samples were taken every 10 min to measure the degree of degradation, recorded by measuring a decrease in the maximum absorbance of the solution (λmax 665 nm) using a UV-Vis spectrophotometer. The degradation ratio was defined as:
Degradation ratio (%) = [(Ai − At)/Ai] × 100; |
where, Ai is the initial absorbance of the dye solutions and At is the absorbance of the dye at any time interval.
2.6 Reactive oxygen species (ROS) assays
The generation of reactive oxygen species (ROS) during the catalytic reaction of the experiment was determined by treating with their respective quenchers. Isopropyl alcohol (IPA), dimethyl sulfoxide (DMSO) and tert-butyl alcohol (TBA) were used as hydroxyl radical quenchers. Sodium azide (SA) was used as a singlet oxygen quencher. Ethylenediaminetetraacetic acid (EDTA) acted as a hole (h+) scavenger. If dye degradation was triggered due to the generation of any of these ROS, then the catalytic dye degradation would be inhibited in the presence of their respective quenchers.
Solutions of MB at 20 mg L−1 were mixed with 5% of IPA, TBA and DMSO, SA at 50 mM and EDTA at 4 mM. The solutions were then treated with CDs at 0.50 mg m L−1 and H2O2 at 147 mM at 50 °C. The inhibition of the degradation was studied by monitoring the intensity of the UV-visible absorption spectrum of the solutions for 30 min. A control experiment was run without adding any of the respective radical quenchers.
2.7 Bioluminescence inhibition testing of effluents (Microtox assay)
The toxicity of the samples before, during and after MB degradation was assessed by inhibition of Aliivibrio fischeri (A. fischeri) using a Microtox® Model 500 Analyser following the manufacturer's instructions. In this test, the marine bacteria A. fischeri (Microtox reagent – MODERN WATER Microtox®) and the reconstitution medium were supplied by Streamline Hydro Pty. Ltd. Solutions containing 2% and 22% of NaCl were used as the diluent and osmotic solution, respectively. Following reconstitution of the freeze-dried A. fischeri, the marine bacteria in solution were exposed to the samples and inhibition of bioluminescence was measured at 5, 10 and 15 min. The EC50 of each sample was calculated using the provided software and expressed as a percent effluent concentration (ASTM, 2004).
2.8 Statistical analysis
Degradation experiments were independently repeated three times, and the results are presented as mean ± standard deviation (SD). One-way analysis of variance (ANOVA) was conducted to evaluate the effects of different parameters. p < 0.05 was considered significantly different.
3. Results and discussion
3.1 Characterisation of CDs
To determine the key properties of the CDs, a series of characterisation techniques were utilised. Surface chemistry is generally one of the most important properties affecting the catalytic performance of CDs; therefore, the assessment of this property and identification of the main surface functional groups is necessary. An FTIR spectrum was used to study the functional groups on the surface of CDs. The surface chemical composition of the CDs analysed by FTIR provides fundamental information on whether chemical groups were created and incorporated onto the surface of CDs, consequently confirming their functionalisation (Fig. 1a).
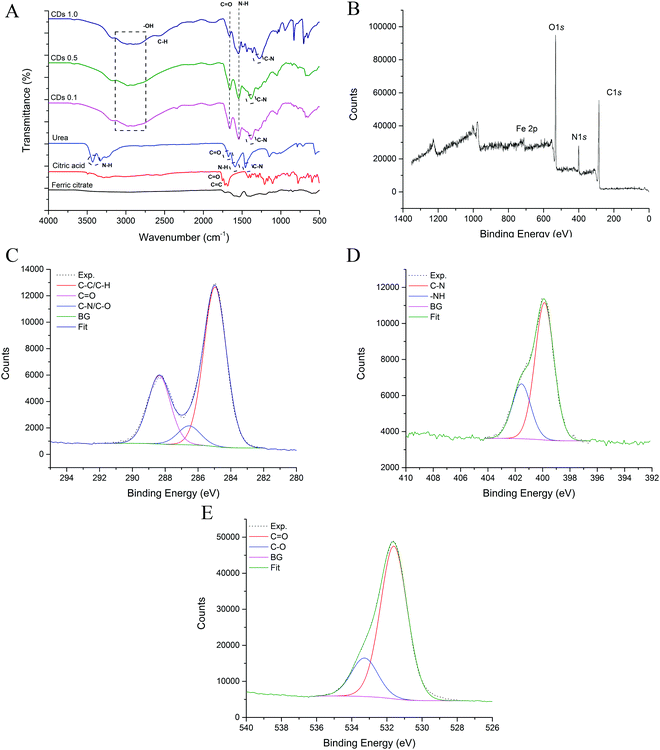 |
| Fig. 1 Spectroscopic characterisation of Fe, N-CDs. (a) Fourier-transform infrared (FTIR) spectra of CDs and precursors. (b) XPS full spectra of CDs 1. (c) C 1s spectra of CDs 1. (d) N 1s spectra of CDs 1. (e) O 1s spectra of CDs 1. | |
Urea and citric acid are popular precursors for the synthesis of CDs.28,29 In this study, ferric citrate was also incorporated into the synthesis in order to introduce metal ions onto the CDs, potentially enhancing their catalytic activity.22 As shown in Fig. 1, the FTIR spectrum of the CDs displays a broad peak at 3000 cm−1, attributed to the stretching vibration of –OH and the –NH group.30 The peaks at 1550 cm−1 and 1660 cm−1 can be assigned to the stretching vibration of N–H and C
O, respectively,27 while the absorption at 1265 cm−1 can be assigned to C–N.31 These are peaks shared among the CD samples; however, there are additional peaks in the CDs 1.0 sample including an absorption peak at 2562 cm−1, attributed to C–H and a peak in the fingerprint region at 707 cm−1 that may correspond to Fe–O bonding.32 Another peak at 830 cm−1 can be attributed to triazine units.33 Notably, functional groups were embedded on the surface of the nanoparticles which endowed them with excellent water-dispersibility, an important feature for efficient catalysis.
To determine the chemical composition and provide further information on the chemical bonds and functionalisation of the CDs, XPS was performed. The calculated XPS elemental atomic composition was 42.40% C, 43.68% O, 13.00% N, and 0.93% Fe (survey spectrum in Fig. 1b) for Fe, N-CDs 1.0. As shown in Fig. 1c, the C 1s spectrum consists of three peaks that can be assigned to C
O (288.37 eV), C–N/C–O (286.54 eV) and C–C/C–H (284.96 eV) which is in agreement with the results obtained from FTIR. Such carbon bonds are commonly found among many CDs.27,33,34 As shown in Fig. 1d, there were two main peaks in the N 1s spectrum assigned to C–N (399.86 eV) and –NH (401.55 eV) which were present due to the introduction of urea during the hydrothermal synthesis of the CDs. The speciation of O 1s was mainly C
O (531.60 eV) and C–O (533.27 eV) (Fig. 1e). The presence of such O-containing functional groups on their surfaces resulted in good dispersion of CDs in water and therefore the potential active sites during catalysis.35,36 Spectroscopy revealed the presence of key functional groups that could endow CDs with rapid electron transfer properties. Such a feature is due to the presence of multiple C, O, N and Fe bonds that increases the electron density and mobility in the CDs. This would potentially accelerate the electron transfer from the CDs to H2O2 during Fenton-like catalysis, increasing the degradation or oxidation rate of pollutants.35
Transmission Electron Microscopy (TEM) was performed to visualise the morphology and size of the CDs. Fig. 2a shows a typical TEM image of the Fe, N-CDs 1.0 prepared using hydrothermal synthesis. The Fe, N-CDs were spherical and distributed uniformly; a histogram of nanoparticle size distribution displays an average diameter of 7.12 nm (Fig. 2b). Importantly, the small size of CDs may provide a large specific surface area which is an important feature for enhancing catalysis. In natural and UV light at 365 nm the solution containing CDs showed brownish and blue colours, respectively, as depicted by the inset photo (Fig. 2a).
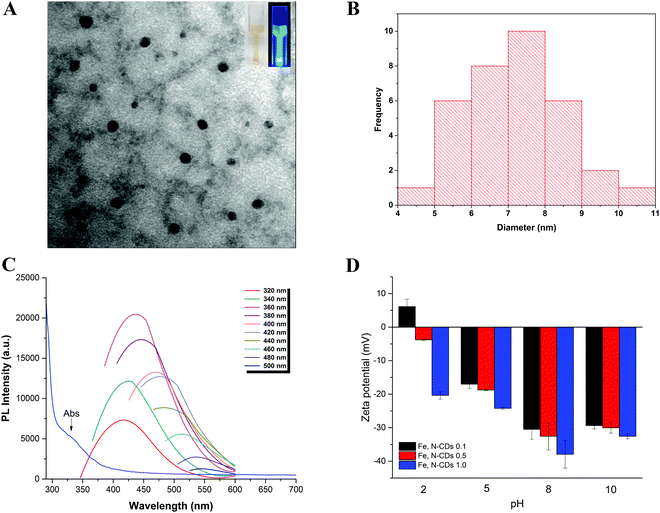 |
| Fig. 2 (a) TEM of Fe, N-CDs at a magnification of 100.000× and inset photos of solutions under natural and UV light. (b) Histogram of particle size distribution. (c) Absorbance and PL intensity measurements of the Fe, N-CDs solution. (d) Zeta potential measurements of CDs across a range of pH values. | |
The optical properties of CDs are their classic signature. The main purpose of studying the photoluminescence properties of CDs in this work was to confirm the formation of such luminescent carbon nanoparticles ensuring their quality by checking the bandwidth consistency in the PL spectrum. The PL spectra of CDs are usually broad which indicates the presence of different particle sizes and a variety of surface functional groups.37–40 Both absorbance and PL spectra of the Fe, N-CDS were studied (Fig. 2c). The dots exhibited an absorption peak at 310–330 nm which can be associated with n–π*(C
O) transition.41 The PL emission of the CDs was dependent on the excitation wavelength and showed bandwidth consistency among the different wavelengths analysed. The emission peak intensity firstly increased, and then decreased significantly as excitation shifted to longer wavelengths. Optimum emission was detected at 420 nm under excitation at 360 nm which is also characteristic of other CDs.31 The origins of the fluorescence of CDs are still under investigation and remain unclear although several studies are underway; some recent studies have suggested that it may be associated with the presence of different structures and chemical composition of CDs.42,43
Another important property that may contribute to the catalytic activity along with the surface active sites of the CDs is their surface charge.44 A stronger electrostatic affinity between the negatively charged catalyst and the cationic dye is likely to contribute positively to the catalytic reaction. We speculate that due to the ROS they will have a relatively short lifetime in solution and thus can only travel a short distance from the CD with their concentration falling dramatically with distance from this source. Thus, the closer the dye to the dot the higher the ROS concentration and the more effective its degradation. A high surface charge on the dot will attract the dye maximizing its degradation efficiency. The surface charge of the dots was assessed through measurement of their zeta potential across a range of pH values from 2 to 10 (Fig. 2d). The pH-dependent surface charge shows a general trend of increasing negative character of the surface with increasing iron content which is believed to be due to the chemistry of iron.45 A similar trend was also observed in other studies with iron based nanoparticles.46 The negative zeta potential measurements may be associated with the presence of carboxylic acids, carboxyl and hydroxyl groups, and iron(III), but become less negative at low pH due to a higher hydrogen ion concentration at acidic pH values.47 The most negative zeta potential for all samples was observed at pH 8, −30.5 mV, −32.56 mV and −37.93 mV for CDs 0.1, CDs 0.5 and CDs 1.0, respectively.
3.2 Optimisation of MB degradation by Fe, N-CDs using the Taguchi approach
The catalytic efficiency of CDs in the presence of hydrogen peroxide is determined by environmental factors such as temperature, pH, as well as H2O2 and catalyst concentrations. In order to reach the maximal degradation rate of pollutants, Taguchi analysis was performed to optimise the environmental factors influencing MB degradation. A Taguchi based experiment design with an L16 orthogonal array was used. The experiments were performed in triplicate, and S/N ratios were determined according to the responses where the highest mean S/N ratio of each level implied the optimal value for the individual factor (Table 1). Furthermore, the factors were distributed by ranking methodology derived from the calculation of the delta statistics value. The delta statistics value of each factor was derived from the difference between the maximum and minimum average response (signal-to-noise ratio or standard deviation) for the factor.
Table 1 Response table for signal to noise ratios
Level |
Temperature |
pH |
Catalyst dose (mg mL−1) |
H2O2 dose (mM) |
1 |
33.23 |
35.42 |
32.52 |
33.24 |
2 |
34.93 |
35.73 |
35.62 |
35.79 |
3 |
36.13 |
35.42 |
37.94 |
37.46 |
4 |
39.33 |
37.05 |
37.54 |
37.12 |
Delta |
6.10 |
1.63 |
5.42 |
4.22 |
Rank |
1 |
4 |
2 |
3 |
The Taguchi analysis demonstrated that temperature had the most significant effect on the degradation of MB which was determined by the highest delta value (Table 1). The efficacy of the treatment of effluents by AOPs is known to be highly affected by temperature. Although the temperature used for Fenton-like processes is generally around 25–30 °C,8 the effect of higher temperatures is still investigated by many researchers due to the fact that (i) textile effluents are usually approximately 40–50 °C,3 and (ii) higher temperature could be beneficial to reduce the catalyst dose. Followed by temperature, the catalyst and H2O2 dose showed the second and third highest delta values, 5.42 and 4.22, respectively. Regarding catalyst dose, studies have reported similar catalytic behaviour of CDs in systems where it appears the catalytic efficiency is controlled by stoichiometry.48 The concentration of CDs should be optimised for each individual reaction as there is no general concentration ratio to be applied. Finally, pH showed the lowest delta value (1.63); however, it is widely known that pH can have significant effects on the treatment of effluents especially for Fenton reactions where degradation benefits from acidic pH values as it facilitates the production of hydroxyl radicals.7,25
Taguchi analysis also revealed the impact of variations of each factor in terms of S/N ratios (Fig. 3). The optimum conditions to achieve the highest degradation efficiency of MB, established using the Taguchi method were: temperature, 50 °C; catalyst dose, 0.50 mg mL−1; H2O2 dose, 147 mM, and pH, 10. Additionally, an assessment of the analysis of variance (ANOVA) was performed for each factor in order to determine whether it was significant (p ≤ 0.05).
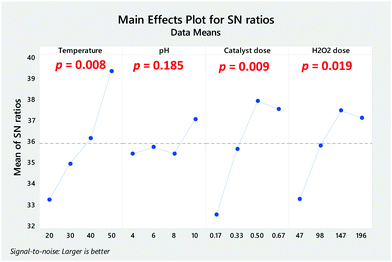 |
| Fig. 3 Main effects plot for SN ratios resulting from the Taguchi optimisation analysis. | |
Fig. 3 shows that all factors were significant except for pH (p = 0.185). The international pH standards for dye effluent discharge in the environment stand between 6 and 9.3 Therefore, investigating catalysts that are able to operate and accelerate in situ production of ROS at near-neutral and slightly basic values are highly desired.
3.3 Evaluation of the catalytic degradation of MB by Fe, N-CDs
The efficiency of Fe, N-CDs as nanocatalysts in the Fenton-like degradation of MB was tested (Fig. 4a). As a result of the Taguchi optimisation analysis, the conditions used in the experiment were mild as the temperature was set to 50 °C, pH at 8, catalyst dose at 0.50 mg mL−1 and H2O2 at 147 mM. The degradation rate of MB was enhanced by the addition of Fe, N-CDs in the presence of H2O2. More than 97% of MB was removed within only 60 min by the Fe, N-CDs while its control removed only 22%, and within 120 min 100% removal was reached.
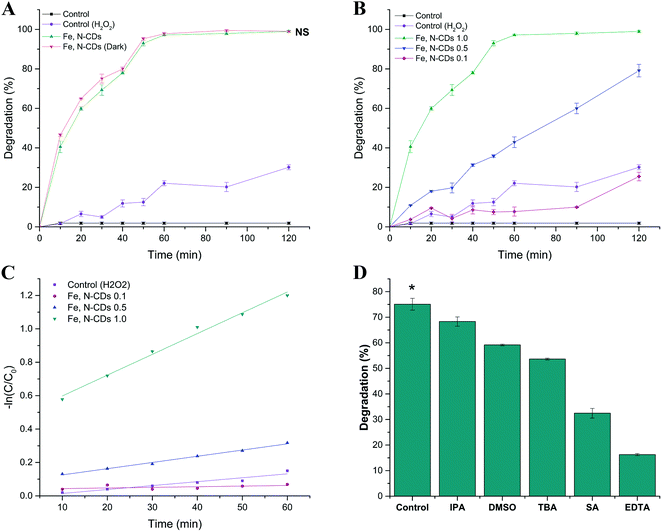 |
| Fig. 4 Fenton-like degradation of methylene blue by Fe, N-CDs. (a) MB dye degradation efficiencies by Fenton-like reaction using Fe, N-CDs 1.0 at a temperature at 50 °C, 120 rpm, H2O2 concentration, 147 mM, under natural and dark conditions. (b) MB dye degradation efficiencies by Fenton-like reaction of different iron concentrations: Fe, N-CDs 0.1, Fe, N-CDs 0.5 and Fe, N-CDs 1.0. (c) Apparent first-order linear transforms, −ln(C/C0), of MB in the presence of different Fe, N-CDs samples. (d) Scavenger test using IPA, DMSO and TBA for hydroxyl radicals, sodium azide for singlet oxygen and EDTA for holes. Time of reaction: 30 minutes. | |
The mild conditions used in the reaction are preferred over harsher reaction conditions employed in other studies.49–51 Several nanoparticles have been tested as Fenton-like catalysts for MB degradation; however, a much higher temperature (90 °C) and very acidic pH (3.5) were employed to achieve a relatively lower degradation rate when compared to this study.50
To determine if light had any effect on the catalytic degradation using dots, reactions were also performed in the dark. As shown in Fig. 4a, the experiment conducted under light and dark conditions did not exhibit any significant difference (p = 0.27). Therefore, light did not influence the degradation. Catalytic activities were also observed for nanozymes (nanomaterial-based artificial enzymes) in the absence of any special light source.19,52,53 In these studies, catalytic performance was attributed to the doped Fe elements in the Fe-CDs where they exhibited superior catalytic performance over horseradish peroxidase (HRP) which is the natural standard enzyme.19,52 The non-dependence of light in this system may be considered as an additional economical advantage over the metallic nanocomposites usually used in photocatalysis.
The effect of different iron(III) ratios in the composition of CDs was also evaluated in the degradation of MB. When compared to the general CDs, the introduction of metal ions may result in new physicochemical characteristics of the CDs that can provide them with new catalytic active centres, improved catalytic activity, and increased binding selectivity, which explains their potential use in the catalysis field.19,22,54 Thus, three different citric acid
:
urea
:
ferric citrate ratios were used to study the effect of the iron on the catalytic activity of the CDs. The ratios were as follows: 1.0
:
0.5
:
0.1; 1.0
:
0.5
:
0.5 and 1.0
:
0.5
:
1.0.
Fig. 4b shows that maximum degradation was achieved using a 1.0
:
0.5
:
1.0 ratio. The increased loading of iron(III) in the CDs led to a corresponding enhancement in their catalytic property for MB degradation. Methylene blue removal increased from 42.85% using Fe, N-CDs 0.5 to 97.20% using Fe, N-CDs 1.0 after 60 min under the same conditions. A positive relationship between the catalytic activity and the iron content on the dots was observed, confirming that CDs functionalised with the correct iron ratio should be considered for successful catalysis.
As shown in Fig. 4c, kinetics plots show an apparent first-order linear form (−ln(C/C0) = kappt) where C0 is the initial concentration of MB and C is the concentration of MB at t-min reaction. The activities of the nanoparticles were evaluated by comparing the apparent first-order rate constants (kapp) listed as follows: 0.02 min−1, 0.005 min−1, 0.001 min−1 and 0.001 min−1 for Fe, N-CDs 1.0; Fe, N-CDs 0.5; Fe, N-CDs 0.1; and control, respectively. Linear transformations confirmed that Fe, N-CDs 1.0 showed the greatest degradation rate. In comparison with data from the literature reporting the degradation of MB using catalysts, this study shows comparable results, achieved using environmentally friendly conditions (Table 2). In terms of kinetics, faster catalysts for the MB degradation have been reported; however, these catalysts showed dependence on higher H2O2 doses ranging from 3.2 to 6.8 times more than the Fe, N-CDs.12,50,55 Moreover, some of the Fenton-like catalysts were only efficient under UV light irradiation which may increase the overall costs of the technology due to the need of an additional energy source.49,56 Furthermore, harsh reaction conditions and the use of expensive precursors were employed for the synthesis of some catalysts;57,58 this is rather disadvantageous from both economic and environmental points of view. Green synthesis concepts should be considered when developing and implementing catalysts to overcome such drawbacks.
Table 2 Use of different catalysts and parameters in the degradation of MB via Fenton-like reactions
Catalyst |
MB concentration (mg L−1) |
H2O2 |
Temperature (°C) |
pH |
Reaction time (min) |
Removal (%) |
K (min−1) |
Ref. |
Photo-Fenton-like barium M-hexaferrites nanoparticles SMN-NP (0.75 mg mL−1) |
10 |
8 mM |
Room temperature |
3.0 |
140 |
98.9 |
0.032 |
56
|
Fenton-like MnMgFe-layered double hydroxide (1.0 mg mL−1) |
20 |
∼1 M |
25 |
7.0 |
300 |
68 |
— |
55
|
Photo-Fenton-like CuFe2O4 nanoparticles (0.2 mg mL−1) |
50 |
20 mM |
25 |
5.0 |
80 |
52 |
0.0117 |
49
|
Fenton-like Fe3O4/SiO2/C nanospheres (1.0 mg mL−1) |
100 |
480mM |
50 |
6.0 |
60 |
90 |
0.036 |
12
|
Fenton-like Cu9S5 nanospheres (0.2 mg mL−1) |
20 |
∼60 mM |
50 |
From 5.0 to 9.0 |
21 |
98 |
— |
58
|
Fenton-like iron oxide nanoparticles (M-NPs) (2.0 mg mL−1) |
100 |
560 mM |
90 |
3.5 |
90 |
100 |
0.15 |
50
|
Fenton-like gold nanoparticles deposited on ZnO (Au/ZnO) (1.05 mg mL−1) |
15 |
∼500mM |
Room temperature |
7.5 |
120 |
90 |
0.0032 |
57
|
Fenton-like Fe, N-CDs (0.5 mg mL−1) |
20 |
147 mM |
50 |
8 |
60 |
97.5 |
0.02 |
This study |
3.4 Investigation of the mechanism of action
The formation of free radicals during the degradation of MB was studied using the free radical quenching technique. In general, free radicals are known as key active species in the catalytic oxidation processes. Free radicals such as hydroxyls, singlet oxygens and holes are powerful oxidants for many organic molecules and are widely involved in the mechanism of action of various catalysts for water and wastewater remediation.59,60
Hydroxyl scavengers such as IPA, TBA and DMSO are commonly used.61,62 These reagents were added to the catalytic reaction system in order to capture ˙OH during the oxidation of MB under pH 8, 50 °C for 30 min. Fig. 4d shows that the degradation rate of MB was inhibited using IPA, TBA and DMSO by 75.0% to 68.3%, 53.6%, and 59.2%, respectively. Similarly, SA was added to quench singlet oxygen and EDTA to quench holes formed during the reaction. The results showed that the addition of singlet oxygen and hole scavengers suppressed the degradation to 32.4% and 16.2%, respectively. These results demonstrated that the addition of such scavengers caused a significant decrease in the degradation of MB. Notably, the presence of free radicals formed during the Fenton-like catalysis by the Fe, N-CDs led to the degradation of MB.
To support the investigation on the mechanism of action of the Fe, N-CDs, spectroscopic analyses were performed on CDs following exposure to H2O2. Fig. 5a shows the FTIR spectrum of Fe, N-CDs following the addition of H2O2. Overall, the data was similar to its original spectrum (Fig. 1a) where a broad band at 3000 cm−1 was attributed to the stretching vibrations of –OH and –NH groups. An absorption peak at 2340 cm−1 was assigned to C–H and the peaks at 1550 cm−1, 1660 cm−1, and 1265 cm−1 could be assigned to the stretching vibration of N–H, C
O, and C–N, respectively. Additionally, the total XPS spectrum showed four peaks attributed to C 1s, O 1s, N 1s and Fe 2p at 284.92 eV, 531.72 eV, 400.02 eV and 710.51 eV, respectively (Fig. 5b). Fig. 5e shows the O 1s spectrum where two peaks were assigned as C
O (at 531.64 eV) and C–O (at 533.31 eV). A reduction in the total O 1s atomic concentration from 43.68% to 35.22% was observed, indicating that H2O2 interacted with the C
O and C–O surface groups of the dots.
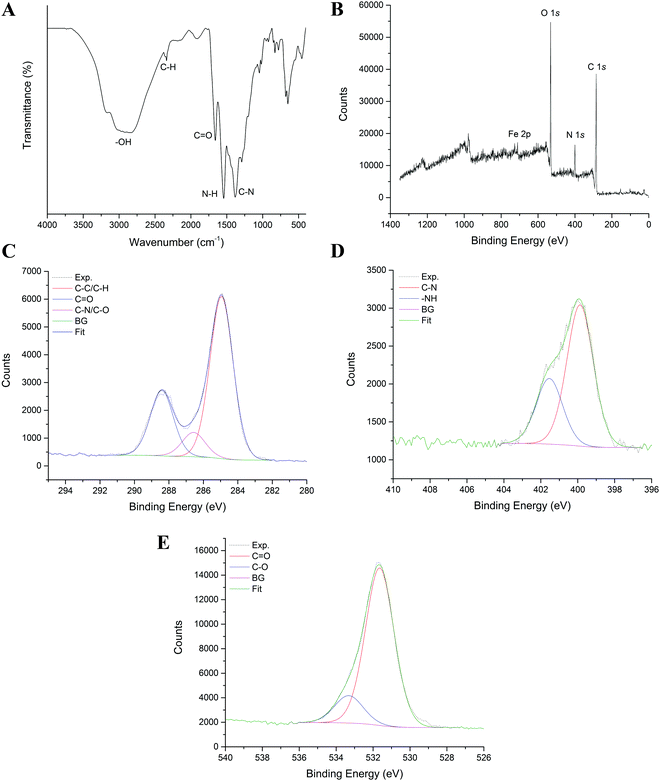 |
| Fig. 5 Spectroscopic characterisation of CDs after exposure to H2O2. (a) FTIR spectra of CDs after addition of H2O2. (b) XPS full scan spectra of CDs. (c) C 1s spectra of CDs. (d) N 1s spectra of CDs. (e) O 1s spectra of CDs. | |
Some slight changes were also observed for nitrogen bonds (Fig. 5d). The binding energies of the N 1s spectrum at 399.87 eV and 401.54 eV were related to C–N and –NH groups, respectively. Following H2O2 reaction, no new peaks were observed, and there were only small changes in the atomic concentration of the original peak such as the reduction from 9.38% to 6.61% for C–N and from 3.75% to 3.07% for –NH groups. These changes implied that both groups participated in the catalytic reaction along with oxygen groups potentially by mediating electron transfer with H2O2.27,36,63 The high-resolution C 1s spectrum can be divided into three peaks, corresponding to C
O (14.16%), C–N/C–O (5.28%), and C–C/C–H (35.12%) (Fig. 5c). There were small changes following H2O2 addition, although the total C 1s atomic concentration increased from 42.40% to 54.60%. This increase might be due to carbon surface oxidation reactions. Finally, the atomic concentration of iron was reduced from 0.93% to 0.62% suggesting that iron participated in the catalytic activity of the CDs.
Based on the results and discussion above, the mechanism of action of Fe, N-CDs towards the degradation of MB is likely due to the catalytic functionalities of the surface groups of the CDs. Such physicochemical properties play a crucial role in the Fenton-like degradation of the dye. Firstly, the negative charge and high surface area of the dots may influence the electrostatic attraction with the positively charged MB. Following H2O2 addition, a series of reactions were triggered involving the inherent ability of CDs to donate and accept electrons which is an important feature for Fenton-like catalysts.30,35,64 Nitrogen, oxygen and iron groups participated as potential active sites during the catalytic reaction. Previous studies have reported that tuning the surface properties of nanomaterials resulted in high catalytic activities44,65 and according to the current investigation this also applies to CDs. Thus, similar strategies should be further examined in CDs which may likely result in enhanced environmental remediation.
3.4 Ecotoxicity analysis
The toxicity of the by-products formed during the remediation process is important to determine in order to evaluate whether the approach is effective and environmentally friendly or not. To assess the ecological risk of the samples subjected to Fenton-like degradation by the dots in this study, a standard toxicity assay (Ecotox) was performed based on the bioluminescence of the bacteria A. fischeri. The toxicity of the samples was expressed as the effective concentration at which 50% (EC50) reduction in luminescence occurs relative to the blank during the test time (15 min). The EC50 was expressed as a percent effluent concentration.
Fig. 6 shows changes in the EC50 during the reaction evaluated by the Ecotox test. As seen, at time 0, both the treated sample and the respective control presented an EC50 of 0.21% and 0.88%, respectively, confirming that both samples showed initially high toxicity. After 2 hours of treatment, the inhibition effects declined for the remediated samples by Fe, N-CDs, with EC50 values increasing up to 6 times the initial value (1.20%) while the control treatment (H2O2 alone) became more toxic (0.53%). After 24 h, the remediated sample by Fe, N-CDs had an EC50 value of 97.80% confirming its significantly reduced toxicity whilst the control (H2O2 alone) remained highly toxic (EC50 of 1.20%).
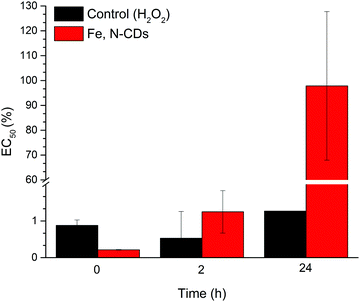 |
| Fig. 6 EC50 values (%) of the remediated (Fe, N-CDs) and control (H2O2) after time 0, 2 and 24 h. | |
Many studies that have applied AOPs to remove dyes showed higher toxicity in treated effluents24,25,66 or the same toxicity levels as the non-treated effluents and/or their parent compounds.67,68 The consequent breakdown of the dyes during the degradation might lead to the formation of toxic by-products23,24 which implies the need of further treatment to minimise its toxicity and deliver a safe discard into the environment. Our results suggest that the catalytic degradation performed by Fe, N-CDs did not generate harmful by-products to aquatic organisms, and therefore represents an alternative treatment for wastewater containing cationic dyes and other pollutants which significantly reduces the toxicity of the effluent.
In summary, these findings indicate that the remediation strategy applied in this study represents an ecofriendly approach from its synthesis to its final release in the environment. With the potential application of CDs in several fields of study, their interactions with the environment need to be assessed. Therefore, understanding of the toxicological impacts of CDs will enable better environmental deployment of nanomaterials.
4. Conclusions
This study investigated the applicability of Fe, N-CDs as nanocatalysts for the degradation of MB in water. Iron and nitrogen-containing carbon dots were successfully synthesised via a simple, economical and green route which generated spherical 7 nm dots negatively charged and rich in amines, iron, hydroxyls and carboxyl functional groups. The application of the dots as catalysts in the Fenton-like degradation resulted in almost 100% removal of MB in only 60 min under pH 8 at 50 °C. Spectroscopic analyses revealed that nitrogen, oxygen and iron groups participated as potential active sites during the catalytic reaction. Moreover, the high catalytic activity of the dots was investigated in terms of the formation of free radicals during the reaction, revealing that hydroxyl radicals, singlet oxygen and holes were formed during the degradation. This remediation strategy aimed to remove the contaminants in a mild and eco-friendly way; therefore, it was fundamental to determine changes in the toxicity of the effluents following remediation. The use of Fe, N-CDs resulted in a significant reduction in the toxicity of the effluent. It thus appears that the intriguing question asked in the introduction is answered in the affirmative: that is the application of the newly investigated metal ion-containing carbon dots, with their enhanced catalytic activity do indeed result in more rapid degradation of contaminants. Thus, the application of Fe, N-CDs shows promise as a potential approach for treating wastewater containing methylene blue resulting not only in a decolourised effluent but also one with significantly reduced ecotoxicity. Furthermore, the synthesis of Fe, N-CDs is simple and thus in principle can be readily scaled up, unlike some more complex nano-structures proposed for similar applications. This method could be applied for a variety of other hazardous contaminants on a large scale as a cost-effective, efficient, feasible, and green remediation approach.
Conflicts of interest
There are no conflicts to declare.
Acknowledgements
We would like to thank the Brazilian National Research Council (CNPq) for the PhD Scholarship granted to Sabrina A. Beker (process number #200697/2017-0/GDE). The authors acknowledge the facilities, and the scientific and technical assistance of the RMIT Microscopy & Microanalysis Facility (RMMF), a linked laboratory of Microscopy Australia.
References
- N. B. Singh, G. Nagpal, S. Agrawal and Rachna, Water purification by using Adsorbents: A Review, Environ. Technol. Innovation, 2018, 11, 187–240 CrossRef.
- B. Lellis, C. Z. Fávaro-Polonio, J. A. Pamphile and J. C. Polonio, Effects of textile dyes on health and the environment and bioremediation potential of living organisms, Biotechnol. Res. Innovation, 2019, 3(2), 275–290 CrossRef.
- V. Katheresan, J. Kansedo and S. Y. Lau, Efficiency of various recent wastewater dye removal methods: A review, J. Environ. Chem. Eng., 2018, 6(4), 4676–4697 CrossRef CAS.
- M. Behera, J. Nayak, S. Banerjee, S. Chakrabortty and S. K. Tripathy, A review on the treatment of textile industry waste effluents towards the development of efficient mitigation strategy: An integrated system design approach, J. Environ. Chem. Eng., 2021, 9(4), 105277 CrossRef CAS.
- M. Rani and U. Shanker, Sun-light driven rapid photocatalytic degradation of methylene blue by poly(methyl methacrylate)/metal oxide nanocomposites, Colloids Surf., A, 2018, 559, 136–147 CrossRef CAS.
- D. B. Miklos, C. Remy, M. Jekel, K. G. Linden, J. E. Drewes and U. Hubner, Evaluation of advanced oxidation processes for water and wastewater treatment - A critical review, Water Res., 2018, 139, 118–131 CrossRef CAS.
- C. S. D. Rodrigues, O. S. G. P. Soares, M. T. Pinho, M. F. R. Pereira and L. M. Madeira, p-Nitrophenol degradation by heterogeneous Fenton's oxidation over activated carbon-based catalysts, Appl. Catal., B, 2017, 219, 109–122 CrossRef CAS.
- N. Wang, T. Zheng, G. Zhang and P. Wang, A review on Fenton-like processes for organic wastewater treatment, J. Environ. Chem. Eng., 2016, 4, 762–787 CrossRef CAS.
- A. Mirzaei, Z. Chen, F. Haghighat and L. Yerushalmi, Removal of pharmaceuticals from water by homo/heterogonous Fenton-type processes - A review, Chemosphere, 2017, 174, 665–688 CrossRef CAS PubMed.
- S. Wang, A Comparative study of Fenton and Fenton-like reaction kinetics in decolourisation of wastewater, Dyes Pigm., 2008, 76(3), 714–720 CrossRef CAS.
- M. Arshadi, M. K. Abdolmaleki, F. Mousavinia, A. Khalafi-Nezhad, H. Firouzabadi and A. Gil, Degradation of methyl orange by heterogeneous Fenton-like oxidation on a nano-organometallic compound in the presence of multi-walled carbon nanotubes, Chem. Eng. Res. Des., 2016, 112, 113–121 CrossRef CAS.
- X. Liu, C. Sun, L. Chen, H. Yang, Z. Ming, Y. Bai, S. Feng and S.-T. Yang, Decoloration of methylene blue by heterogeneous Fenton-like oxidation on Fe3O4/SiO2/C nanospheres in neutral environment, Mater. Chem. Phys., 2018, 213, 231–238 CrossRef CAS.
- Z. Su, J. Li, D. Zhang, P. Ye, H. Li and Y. Yan, Novel flexible Fenton-like catalyst: Unique CuO nanowires arrays on copper mesh with high efficiency across a wide pH range, Sci. Total Environ., 2019, 647, 587–596 CrossRef CAS PubMed.
- H. Sun, A. Zhao, N. Gao, K. Li, J. Ren and X. Qu, Deciphering a nanocarbon-based artificial peroxidase: chemical identification of the catalytically active and substrate-binding sites on graphene quantum dots, Angew. Chem., Int. Ed., 2015, 54(24), 7176–7180 CrossRef CAS PubMed.
- D. M. Wang, M. X. Gao, P. F. Gao, M. X. Gao, H. Yang and C. Z. Huang, Carbon Nanodots-Catalyzed Chemiluminescence of Luminol: A Singlet Oxygen-Induced Mechanism, J. Phys. Chem. C, 2013, 117(37), 19219–19225 CrossRef CAS.
- N. Dhenadhayalan, K. C. Lin and T. A. Saleh, Recent Advances in Functionalized Carbon Dots toward the Design of Efficient Materials for Sensing and Catalysis Applications, Small, 2020, 16(1), e1905767 CrossRef PubMed.
- A. Habibi-Yangjeh, S. Feizpoor, D. Seifzadeh and S. Ghosh, Improving visible-light-induced photocatalytic ability of TiO2 through coupling with Bi3O4Cl and carbon dot nanoparticles, Sep. Purif. Technol., 2020, 238, 116404 CrossRef CAS.
- W. Li, C. Zhao and Q. Zhang, Synthesis of Bi/BiOCl-TiO2-CQDs quaternary photocatalyst with enhanced visible-light photoactivity and fast charge migration, Catal. Commun., 2018, 107, 74–77 CrossRef CAS.
- W. Yang, T. Huang, M. Zhao, F. Luo, W. Weng, Q. Wei, Z. Lin and G. Chen, High peroxidase-like activity of iron and nitrogen co-doped carbon dots and its application in immunosorbent assay, Talanta, 2017, 164, 1–6 CrossRef CAS.
- C. Zheng, X. An and T. Yin, New metal-free catalytic degradation systems with carbon dots for thymol blue, New J. Chem., 2017, 41(22), 13365–13369 RSC.
- J. Shi, T. Yin and W. Shen, Effect of surface modification on the peroxidase-like behaviors of carbon dots, Colloids Surf., B, 2019, 178, 163–169 CrossRef CAS.
- L. Lin, Y. Luo, P. Tsai, J. Wang and X. Chen, Metal ions doped carbon quantum dots: Synthesis, physicochemical properties, and their applications, TrAC, Trends Anal. Chem., 2018, 103, 87–101 CrossRef CAS.
- L. Rizzo, Bioassays as a tool for evaluating advanced oxidation processes in water and wastewater treatment, Water Res., 2011, 45(15), 4311–4340 CrossRef CAS PubMed.
- B. L. Alderete, J. Silva, R. Godoi, F. R. Silva, S. R. Taffarel, L. P. Silva, A. L. H. Garcia, H. M. Junior, H. L. N. Amorim and J. N. Picada, Evaluation of toxicity and mutagenicity of a synthetic effluent containing azo dye after Advanced Oxidation Process treatment, Chemosphere, 2021, 263, 128291 CrossRef CAS PubMed.
- E. O. Marson, V. A. B. de Paiva, B. R. Goncalves, O. Gomes Junior, W. Borges Neto, A. E. H. Machado and A. G. Trovo, Degradation of Direct Red 81 mediated by Fenton reactions: multivariate optimization, effect of chloride and sulfate, and acute ecotoxicity assessment, Environ. Sci. Pollut. Res. Int., 2017, 24(7), 6176–6186 CrossRef CAS PubMed.
- M. M. Vieira, A. S. Pereira Dornelas, T. D. Carlos, A. Pallini, C. Gravato, D. H. Pereira, R. A. Sarmento and G. S. Cavallini, When treatment increases the contaminant’s ecotoxicity: A study of the Fenton process in the degradation of methylene blue, Chemosphere, 2021, 283, 131117 CrossRef CAS PubMed.
- S. A. Beker, A. Truskewycz, I. Cole and A. S. Ball, Green synthesis of Opuntia-derived carbon nanodots for the catalytic decolourization of cationic dyes, New J. Chem., 2020, 44(46), 20001–20012 RSC.
- R. Sendão, M. D. V. M. D. Yuso, M. Algarra, J. C. G. Esteves da Silva and L. Pinto da Silva, Comparative life cycle assessment of bottom-up synthesis routes for carbon dots derived from citric acid and urea, J. Cleaner Prod., 2020, 254 Search PubMed.
- V. Strauss, H. Wang, S. Delacroix, M. Ledendecker and P. Wessig, Carbon nanodots revised: the thermal citric acid/urea reaction, Chem. Sci., 2020, 11(31), 8256–8266 RSC.
- J. Chen, J. Shu, J. Chen, Z. Cao, A. Xiao and Z. Yan, Highly luminescent S,N co-doped carbon quantum dots-sensitized chemiluminescence on luminol-H2 O2 system for the determination of ranitidine, Luminescence, 2017, 32(3), 277–284 CrossRef CAS PubMed.
- P. Das, S. Ganguly, S. Mondal, U. K. Ghorai, P. P. Maity, S. Choudhary, S. Gangopadhyay, S. Dhara, S. Banerjee and N. C. Das, Dual doped biocompatible multicolor luminescent carbon dots for bio labeling, UV-active marker and fluorescent polymer composite, Luminescence, 2018, 33(6), 1136–1145 CrossRef CAS PubMed.
- C. S. Stan, A. Coroaba, E. L. Ursu, M. S. Secula and B. C. Simionescu, Fe(III) doped carbon nanodots with intense green photoluminescence and dispersion medium dependent emission, Sci. Rep., 2019, 9(1), 18893 CrossRef CAS PubMed.
- L. Fang, Z. Liu, C. Zhou, Y. Guo, Y. Feng and M. Yang, Degradation Mechanism of Methylene Blue by H2O2 and Synthesized Carbon Nanodots/Graphitic Carbon Nitride/Fe(II) Composite. The, J. Phys. Chem. C, 2019, 123(44), 26921–26931 CrossRef CAS.
- Y. Hu, J. Yang, J. Tian and J. S. Yu, How do nitrogen-doped carbon dots generate from molecular precursors? An investigation of the formation mechanism and a solution-based large-scale synthesis, J. Mater. Chem. B, 2015, 3(27), 5608–5614 RSC.
- B. B. Chen, M. L. Liu and C. Z. Huang, Carbon dot-based composites for catalytic applications, Green Chem., 2020, 22(13), 4034–4054 RSC.
- H. Wang, C. Liu, Z. Liu, J. Ren and X. Qu, Specific Oxygenated Groups Enriched Graphene Quantum Dots as Highly Efficient Enzyme Mimics, Small, 2018, 14(13), e1703710 CrossRef PubMed.
- S. Hu, A. Trinchi, P. Atkin and I. Cole, Tunable photoluminescence across the entire visible spectrum from carbon dots excited by white light, Angew. Chem., Int. Ed., 2015, 54(10), 2970–2974 CrossRef CAS PubMed.
- P. M. Gharat, J. M. Chethodil, A. P. P. K. P. Srivastava, H. Pal and S. D. Choudhury, An insight into the molecular and surface state photoluminescence of carbon dots revealed through solvent-induced modulations in their excitation wavelength dependent emission properties, Photochem. Photobiol. Sci., 2019, 18(4), 375–385 Search PubMed.
- Z. Zhu, Q. Li, P. Li, X. Xun, L. Zheng, D. Ning and M. Su, Surface charge controlled nucleoli selective staining with nanoscale carbon dots, PLoS One, 2019, 14(5), e0216230 CrossRef CAS PubMed.
- T. Yoshinaga, M. Shinoda, Y. Iso, T. Isobe, A. Ogura and K. I. Takao, Glycothermally Synthesized Carbon Dots with Narrow-Bandwidth and Color-Tunable Solvatochromic Fluorescence for Wide-Color-Gamut Displays, ACS Omega, 2021, 6(2), 1741–1750 CrossRef CAS PubMed.
- M. Zulfajri, G. Gedda, C. J. Chang, Y. P. Chang and G. G. Huang, Cranberry Beans Derived Carbon Dots as a Potential Fluorescence Sensor for Selective Detection of Fe(3+) Ions in Aqueous Solution, ACS Omega, 2019, 4(13), 15382–15392 CrossRef CAS PubMed.
- C. M. Carbonaro, R. Corpino, M. Salis, F. Mocci, S. V. Thakkar, C. Olla and P. C. Ricci, On the Emission Properties of Carbon Dots: Reviewing Data and Discussing Models, C—J. Carbon Res., 2019, 5, 60 CrossRef CAS.
- B. Yao, H. Huang, Y. Liu and Z. Kang, Carbon Dots: A Small Conundrum, Trends Chem., 2019, 1(2), 235–246 CrossRef CAS.
- Y. Bai, H. Huang, C. Wang, R. Long and Y. Xiong, Engineering the surface charge states of nanostructures for enhanced catalytic performance, Mater. Chem. Front., 2017, 1(10), 1951–1964 RSC.
- M. Kosmulski, Isoelectric points and points of zero charge of metal (hydr)oxides: 50years after Parks' review, Adv. Colloid Interface Sci., 2016, 238, 1–61 CrossRef CAS PubMed.
- F. Vereda, A. Martin-Molina, R. Hidalgo-Alvarez and M. Quesada-Perez, Specific ion effects on the electrokinetic properties of iron oxide nanoparticles: experiments and simulations, Phys. Chem. Chem. Phys., 2015, 17(26), 17069–17078 RSC.
- L. Gines, S. Mandal, I. A. Ashek, C. L. Cheng, M. Sow and O. A. Williams, Positive zeta potential of nanodiamonds, Nanoscale, 2017, 9(34), 12549–12555 RSC.
- Y. Long, X. Wang, D. Shen and H. Zheng, Detection of glucose based on the peroxidase-like activity of reduced state carbon dots, Talanta, 2016, 159, 122–126 CrossRef CAS PubMed.
- X. Guo, K. Wang and Y. Xu, Tartaric acid enhanced CuFe2O4-catalyzed heterogeneous photo-Fenton-like degradation of methylene blue, Mater.
Sci. Eng., B, 2019, 245, 75–84 CrossRef CAS.
- F. L. Rivera, F. J. Recio, F. J. Palomares, J. Sánchez-Marcos, N. Menéndez, E. Mazarío and P. Herrasti, Fenton-like degradation enhancement of methylene blue dye with magnetic heating induction, J. Electroanal. Chem., 2020, 879 Search PubMed.
- Q. Qin, Y. Liu, X. Li, T. Sun and Y. Xu, Enhanced heterogeneous Fenton-like degradation of methylene blue by reduced CuFe2O4, RSC Adv., 2018, 8(2), 1071–1077 RSC.
- D. Zhu, S. Zhuo, C. Zhu, P. Zhang and W. Shen, Synthesis of catalytically active peroxidase-like Fe-doped carbon dots and application in ratiometric fluorescence detection of hydrogen peroxide and glucose. Analytical, Methods, 2019, 11(20), 2663–2668 CAS.
- M. Vazquez-Gonzalez, W. C. Liao, R. Cazelles, S. Wang, X. Yu, V. Gutkin and I. Willner, Mimicking Horseradish Peroxidase Functions Using Cu(2+)-Modified Carbon Nitride Nanoparticles or Cu(2+)-Modified Carbon Dots as Heterogeneous Catalysts, ACS Nano, 2017, 11(3), 3247–3253 CrossRef CAS PubMed.
- X. Li, Y. Fu, S. Zhao, J. Xiao, M. Lan, B. Wang, K. Zhang, X. Song and L. Zeng, Metal ions-doped carbon dots: Synthesis, properties, and applications, Chem. Eng. J., 2021, 133101 CrossRef.
- R. G. L. Gonçalves, H. M. Mendes, S. L. Bastos, L. C. D’Agostino, J. Tronto, S. H. Pulcinelli, C. V. Santilli and J. L. Neto, Fenton-like degradation of methylene blue using Mg/Fe and MnMg/Fe layered double hydroxides as reusable catalysts, Appl. Clay Sci., 2020, 187, 105477 CrossRef.
- G. Abbas Ashraf, M. Hassan, R. Tur Rasool, W. Abbas and L. Zhang, Mesoporous SnMgNd substituted M-hexaferrite catalyzed heterogeneous photo-Fenton-like activity for degradation of methylene blue, J. Colloid Interface Sci., 2019, 557, 408–422 CrossRef CAS PubMed.
- L. Wolski, A. Walkowiak and M. Ziolek, Formation of reactive oxygen species upon interaction of Au/ZnO with H2O2 and their activity in methylene blue degradation, Catal. Today, 2019, 333, 54–62 CrossRef CAS.
- X. Luo, H. Hu, Z. Pan, F. Pei, H. Qian, K. Miao, S. Guo, W. Wang and G. Feng, Efficient and stable catalysis of hollow Cu9S5 nanospheres in the Fenton-like degradation of organic dyes, J. Hazard. Mater., 2020, 396, 122735 CrossRef CAS PubMed.
- A. Asghar, A. A. Raman and W. M. A. Daud, Advanced oxidation processes for in-situ production of hydrogen peroxide/hydroxyl radical for textile wastewater treatment: a review, J. Cleaner Prod., 2015, 87, 826–838 CrossRef CAS.
- G. Boczkaj and A. Fernandes, Wastewater treatment by means of advanced oxidation processes at basic pH conditions: A review, Chem. Eng. J., 2017, 320, 608–633 CrossRef CAS.
- N. Keikha, A. Rezaeifard and M. Jafarpour, Heterogeneous Fenton-like activity of novel metallosalophen magnetic nanocomposites: significant anchoring group effect, RSC Adv., 2019, 9(57), 32966–32976 RSC.
- L. Ge, Z. Peng, W. Wang, F. Tan, X. Wang, B. Su, X. Qiao and P. K. Wong, g-C3N4/MgO nanosheets: light-independent, metal-poisoning-free catalysts for the activation of hydrogen peroxide to degrade organics, J. Mater. Chem. A, 2018, 6(34), 16421–16429 RSC.
- O. S. G. P. Soares, C. S. D. Rodrigues, L. M. Madeira and M. F. R. Pereira, Heterogeneous Fenton-Like Degradation of p-Nitrophenol over Tailored Carbon-Based Materials, Catalysts, 2019, 9 DOI:10.3390/catal9030258.
- X. Dou, Z. Lin, H. Chen, Y. Zheng, C. Lu and J. M. Lin, Production of superoxide anion radicals as evidence for carbon nanodots acting as electron donors by the chemiluminescence method, Chem. Commun., 2013, 49(52), 5871–5873 RSC.
- X. Duan, H. Sun, Y. Wang, J. Kang and S. Wang, N-Doping-Induced Nonradical Reaction on Single-Walled Carbon Nanotubes for Catalytic Phenol Oxidation. ACS, Catalysis, 2014, 5(2), 553–559 Search PubMed.
- X. Yu, J. Sun, G. Li, Y. Huang, Y. Li, D. Xia and F. Jiang, Integration of *SO4(-)-based AOP mediated by reusable iron particles and a sulfidogenic process to degrade and detoxify Orange II, Water Res., 2020, 174, 115622 CrossRef CAS PubMed.
- F. Parolin, U. M. Nascimento and E. B. Azevedo, Microwave-enhanced UV/H2O2 degradation of an azo dye (tartrazine): optimization, colour removal, mineralization and ecotoxicity, Environ. Technol., 2013, 34(9–12), 1247–1253 CrossRef CAS PubMed.
- B. P. Dojcinovic, G. M. Roglic, B. M. Obradovic, M. M. Kuraica, M. M. Kostic, J. Nesic and D. D. Manojlovíc, Decolorization of reactive textile dyes using water falling film dielectric barrier discharge, J. Hazard. Mater., 2011, 192(2), 763–771 CrossRef CAS PubMed.
Footnotes |
† Electronic supplementary information (ESI) available. See DOI: 10.1039/d1nj04761b |
‡ Postal address: School of Science, RMIT University, Melbourne, Victoria, 3083, Australia. |
|
This journal is © The Royal Society of Chemistry and the Centre National de la Recherche Scientifique 2022 |