DOI:
10.1039/D1NJ04728K
(Paper)
New J. Chem., 2022,
46, 228-238
Nano-flower S-scheme heterojunction NiAl-LDH/MoS2 for enhancing photocatalytic hydrogen production†
Received
4th October 2021
, Accepted 17th November 2021
First published on 2nd December 2021
Abstract
The construction of heterojunctions can effectively improve the electron transport rate and photocatalytic activity. In this work, nanoflower-like S-scheme heterojunction MoS2/NiAl-LDH was successfully synthesized. The results indicated that the hydrogen yield of the MoS2/NiAl-LDH composite catalyst reached 229.5 μmol in 5 hours under visible light irradiation by adjusting the pH value of the sacrificial agent and the amount of eosin. The crystal structure and morphological characteristics of the composite were analyzed by XRD, SEM and TEM. The visible light response degree and charge separation and transfer rates of photogenerated carriers of the composite were analyzed by UV-Vis DRS, PL and electrochemistry. The electronic transfer path was also analyzed by XPS. The construction of S-scheme heterojunctions can realize effective separation of electron–hole pairs and ensure that the photo-induced carriers with the strongest redox ability can be retained to participate in the photocatalytic reaction. In addition, the feasible mechanism of photocatalytic hydrogen evolution is given. This work will provide different insights into the enhancement of hydrogen evolution by heterojunctions formed by binary semiconductors.
1 Introduction
Due to the destruction of the environment and waste of energy, the earth's resources have been seriously damaged.1,2 Therefore, seeking a kind of green sustainable development of resources becomes particularly important.3–5 In recent years, hydrogen has attracted wide attention because of its advantages such as cleanness, efficiency, wide range of availability and storage properties.6–8 In 1972, Fujishima A and Honda K9 reported, for the first time, the decomposition of aquatic hydrogen by a TiO2 single-crystal electrode, which opened up a path for hydrogen production by solar photolysis of water. As a precious metal, Pt plays an important role in photocatalysis, but its high price and scarce resources limit the development of Pt in the field of photocatalysis.10–12 Therefore, it is very important to find a class of materials that can replace precious metals in reactions.
Layered double hydroxide (LDH) is a kind of two-dimensional material, in which the plate is positively charged and different anions between the layers contribute to the negative charge, and hence, charge balance can be achieved; as a result, whole LDH is not charged, neutral, and has some H2O molecules.13–15 The structural chemical formula is [M2+1−xM3+x(OH)2]X+ [Ax/n]n−·mH2O, in which the +2 valence metal cations include Ni2+, Mg2+, Cu2+, and Zn2+, and the +3 valence metal cations include Al3+, Fe3+, Cr3+, and Sc3+.16,17 Due to the advantages of unfixed chemical element composition, large specific surface area and good stability of LDHs, its structure is easy to adjust and control, and is widely used in photocatalysis and electrocatalysis.18–20 However, due to the slow transfer rate of the photogenerated carriers generated by single LDH by photoexcitation, LDH itself has no obvious effect on hydrogen evolution.21–23 Therefore, many people promote the hydrogen evolution effect by constructing heterojunctions or strengthening coupling between the two semiconductors. For example, NiAl-LDH/CdS promotes hydrogen evolution by constructing S-schemes and consuming useless electrons and holes.24 CoAl-LDH/RGO nanocomposites were synthesized by a solvothermal method, which can effectively transfer and separate photogenerated charge carriers, thus enhancing the properties of the composites.25 The 2D/2D heterojunction constructed by NiAl-LDH/g-C3N4 accelerates the separation of electron holes and promotes the hydrogen evolution effect.26
In the field of photocatalysis, Mo sulfides have become the most promising Pt equators.27–29 Among them, MoS2 has been widely reported in the field of photocatalysis due to its extensive sources, good electrical conductivity and easy preparation.30–32 Moreover, due to its strong absorption capacity in the visible region, narrow band gap and stable chemical properties, MoS2 has excellent photocatalytic performance. MoS2/Mn0.2Cd0.8S,33 MoS2/Cu2S,34 and MoS2/g-C3N435 can accelerate the separation of photogenerated carriers and promote hydrogen evolution by constructing heterojunctions. In order to improve the performance of photocatalytic water decomposition, different types of heterojunctions can be constructed, such as type I, type II and type Z. The S-scheme heterojunction takes into account band bending, electrostatic interaction between semiconductors and built-in electric field, which enriches the model conditions of other heterojunctions.36–38 The S-scheme heterojunction constructed by contacting NiAl-LDH and MoS2 can inhibit the recombination of photo-generated carriers and also increase hydrogen production.
In this study, MoS2 and NiAl-LDH successfully constructed the S-scheme heterojunction, which accelerated the generation and transfer of photogenerated carriers. The addition of MoS2 broadened the absorption and utilization of available light in the composite catalyst and reduced the composite efficiency of photogenerated carriers. Therefore, the photocatalytic activity of NiAl-LDH was significantly improved.
2 Experimental section
2.1 Materials
Nickel(II) nitrate hexahydrate [(Ni(NO3)2·6H2O)], aluminium nitrate nonahydrate [Al(NO3)3·9(H2O)], urea (CH4N2O), ammonium fluoride (NH4F), ammonium molybdate (H8MoN2O4) and thiourea (CH4N2S) were purchased from Aladdin's official.
2.2 Method of MoS2 preparation
MoS2 was prepared by hydrothermal synthesis. First, 1.124 g ammonium molybdate was dissolved in 40 mL distilled water. After complete dissolution, 3.021 g thiourea was added. After ultrasonic stirring, the solution was evenly mixed and transferred to a 100 mL reactor for 10 h reaction at 180 °C. After cooling to room temperature and centrifugation, the reactor was put into an oven and dried at 80 °C for 12 h. The powder catalyst was obtained.
2.3 Method of nano flower NiAl-LDH preparation
NiAl-LDH was prepared by hydrothermal synthesis. First, 0.139 g nickel nitrate and 0.060 g aluminum nitrate were weighed and dissolved in 80 mL water. After ultrasonic stirring for 10 minutes, 0.047 g ammonium fluoride and 0.192 g urea were added, respectively. After stirring for 30 minutes, it was transferred to a 100 mL high-pressure reactor for 24 h reaction at 120 °C. After the temperature was reduced to room temperature, it was centrifuged and washed several times with deionized water and ethanol until it became neutral. The powder catalyst was obtained after drying in an oven at 80 °C for 12 h.
2.4 Preparation of nanometer flower structure catalysts
The process was carried out by physical stirring. First, a certain amount of NiAl-LDH was weighed and dissolved in 30 mL ethanol. After the mixture was evenly mixed, a certain amount of MoS2 was added, followed by ultrasonication for half an hour and stirring for six hours. Then, they were put it in a water bath to evaporate ethanol and get a mixture of the two. Keeping the amount of NiAl-LDH (0.050 g) fixed and unchanged, different amounts of MoS2 were added (0.100 g, 0.150 g, 0.200 g, 0.250 g, and 0.300 g) to vary the quality, and the products were named NM1, NM2, NM3, NM4, and NM5 accordingly (Scheme 1).
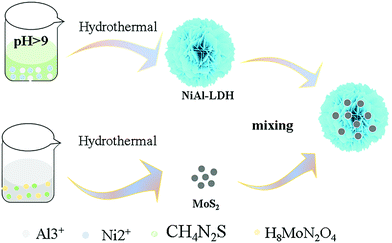 |
| Scheme 1 Schematic diagram of MoS2/NiAl-LDH synthesis. | |
3 Discussion of results
3.1 XRD discussion
Fig. 1(a) shows the XRD patterns of NiAl-LDH. In the XRD pattern of NiAl-LDH alone, the diffraction peak could well correspond to the standard card of NiAl-LDH (JCPDS No. 22-0452).39 The diffraction peaks at 11.54°, 23.27° and 35.08° correspond one-to-one with the diffraction planes of (003), (006) and (012). Fig. 1(b) shows the XRD patterns of MoS2 and NM1 The three diffraction peaks at 14.4°, 32.7° and 58° correspond to the diffraction surfaces of (002), (100) and (110) of MoS2.40,41 As can be seen from Fig. 1b, there are (003) and (012) diffraction surfaces of NiAl-LDH in the composite material. With the increase in MoS2 loading, the diffraction peaks of the (002) crystal plane characteristic of MoS2 gradually increased, but the diffraction peaks of NiAl-LDH became less and less obvious. This may be due to the fact that MoS2 is loaded on the nanoflower structure of NiAl-LDH42 and the high proportion of MoS2 in the composite catalyst. As a result, the crystal structure of NiAl-LDH was changed, and the diffraction peak of NiAl-LDH was covered up.
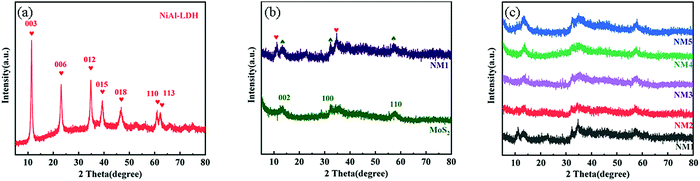 |
| Fig. 1 XRD patterns of (a) NiAl-LDH; (b) MoS2 and NM1; and (c) NMX (X = 1–5). | |
3.2 Surface analysis
3.2.1 SEM analysis.
In order to investigate the morphological characteristics of the samples, field emission scanning electron microscopy (SEM) was performed on the samples. It can be seen from Fig. 2(a) that NiAl-LDH was a nanoflower structure with a diameter of about 3–4 μm. It can be seen from Fig. 2(b) that MoS2 is an irregular nanostructure with a diameter of about 0.4–0.8 μm. Fig. 2(c) and (d) show the morphology of the composite catalyst. It can be seen that there were small nanostructures on the nanostructure of NiAl-LDH, and the sufficient contact between the two provides more paths for electron transfer. The results indicated that the composite catalyst was successfully synthesized.
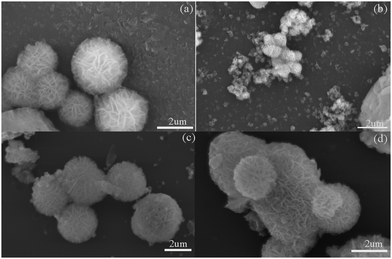 |
| Fig. 2 SEM images of (a) NiAl-LDH; (b) MoS2; (c and d) NM3. | |
3.2.2 TEM analysis.
TEM can better reflect the contact between the two catalysts. Fig. 3(a) shows the TEM image of NM3, and the nano-flower structure of about 3–4 μm can be observed; many MoS2 nano-flower structures are derived at the edge of the nano-flower. The close contact between the two catalysts promotes the charge transfer, which was consistent with the structure analyzed by SEM. The lattice spacing of 0.27 nm corresponds to the (100) crystal plane of MoS2, and 0.261 nm corresponds to the (101) crystal plane of NiAl-LDH. The EDX (S2) image can determine the types of elements in the catalyst (Fig. 3c–h), and the combined element mapping can reflect the uniform distribution of Mo, S, Ni, Al and O elements in the catalyst.
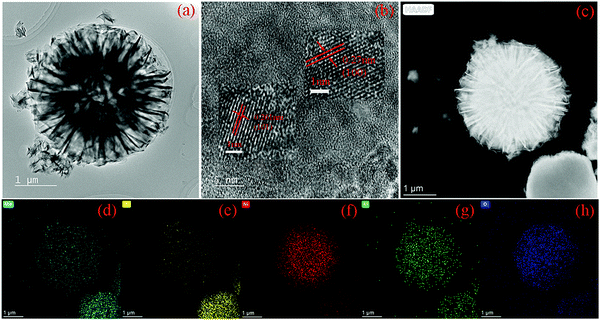 |
| Fig. 3 Images of NM3 (a) TEM and (b) HRTEM, and (c–h) elemental mapping of NM3. | |
3.3 Valence state of elements
The sample was tested by XPS, and the elemental composition and valence state information of the NM3 composite catalytic material was obtained. Five elements, namely, Al, S, Mo, C and Ni, are found in the full spectrum shown in Fig. 4a. In the fine pattern of Al (Fig. 4b), the peaks at 68.2 eV and 74.2 eV correspond to Al 2p1/2 and Al 2p3/2 of Al3+,43 respectively. Mo 3d (Fig. 4c) showed Mo4+ (228.2 eV and 231.4 eV) and Mo6+ (232.2 eV and 235.2 eV), and the binding energy is located at 225.5 eV due to S2s.44–46 The pattern of Ni 2p (Fig. 4d) mainly consists of two principal spectra located at 856.1 eV and 874 eV, which correspond to the spin–orbital pair of Ni 2p3/2 and Ni 2p1/2, and the two satellite peaks correspond to the divalent oxidation state of Ni. The spin energies of Ni 2p3/2 and Ni 2p1/2 were calculated to be 17.9 eV, which is characteristic of the Ni(OH)2 phase in LDH.47,48 The fine pattern of S 2p (Fig. 4f) at 161.2 eV and 162.3 eV further confirmed that the S element reacts to S2− in the composite catalyst.49 The typical peak (531.4 eV) of oxygen is shown in Fig. 4f. It can be seen from S1 that compared with the MoS2 composite catalyst, S 2p and Mo 4d have different degrees of tendency to move towards low binding energies. Similarly, O 1s, Al 2p and Ni 2p have different degrees of tendency to move towards high binding energies compared with the NiAl-LDH composite catalyst, confirming the existence of a built-in electric field, which makes electrons move from NiAl-LDH to MoS2.36,50
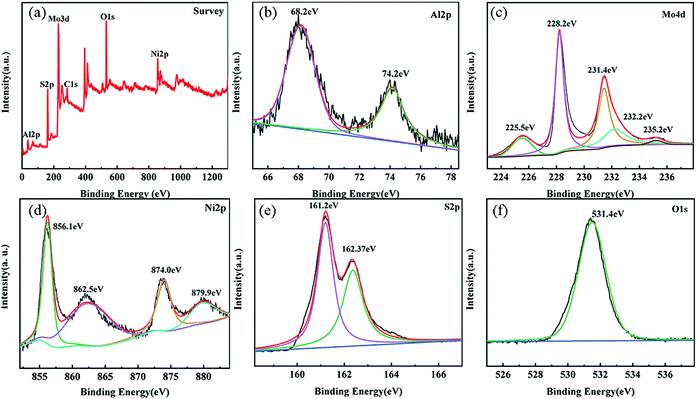 |
| Fig. 4 XPS images of NM3 samples: (a) full spectrum; (b) Al 2p; (c) Mo 4d; (d) Ni 2p; (e) S 2p; and (f) O 1s. | |
3.4 BET analysis
The specific surface area, pore volume and pore diameter of the sample were obtained by measuring the N2 absorption desorption curve of the sample. It can be judged that NiAl-LDH, MoS2 and NM3 are all type IV isotherms (IUPAC classification). It can be judged from the pore diameter distribution curve (Fig. 5b) that all were mesoporous materials with H3 type hysteresis loops. It can be seen from Table 1 that NiAl-LDH has the largest specific surface area, pore volume and pore diameter. The catalyst with a larger specific surface area can expose more active sites and promote the reaction. Compared with NiAl-LDH, the specific surface area, pore volume and pore size of NM3 are smaller, which further proves the successful combination of NiAl-LDH and MoS2. A large number of open space structures exist in the nanoflower structure of NiAl-LDH, which enables the successful embedding of MoS2 nanoparticles. Therefore, the specific surface area of NM3 and NiAl-LDH became smaller.
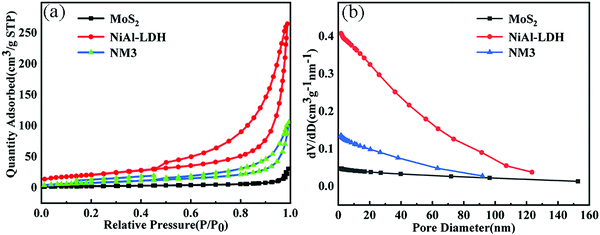 |
| Fig. 5 (a) Adsorption–desorption isotherm of the catalyst. (b) Pore size. | |
Table 1 Surface area (SBET), pore diameter (Dp), and pore volume (Vp) of MoS2, NiAl-LDH and NM3
Samples |
S
BET (m2 g−1) |
Pore volume (cm3 g−1) |
Pore diameter (nm) |
MoS2 |
9.431 |
0.048 |
19.504 |
NiAl-LDH |
75.991 |
0.409 |
21.645 |
NM3 |
30.571 |
0.136 |
15.168 |
3.5 Optical properties
3.5.1 Electrochemistry.
In order to explore the transfer and separation efficiencies of photogenerated carriers and the electrochemical properties of the catalyst itself, transient photo-current (IT), electrochemical impedance spectra (EIS) and polarization curves (LSV) were studied. In Fig. 6(a), due to the accumulation of holes, the NM3 photocurrent intensity decreases slightly, but has the strongest photocurrent intensity, which confirms that the photogenerated carrier transfer efficiency and photocatalytic activity are significantly improved compared with MoS2 and NiAl-LDH. At the same time, the EIS of NM3 has the smallest arc radius (Fig. 6b), which indicates that the composite catalyst is subjected to the least resistance during the transfer of photogenerated carriers. IT and LSV data showed that NM3 had a lower electron coincidence rate and a more efficient electron transfer between the two catalysts, thus increasing the production activity of H2. In addition, the polarization curve (Fig. 6c), the lower the overpotential, the better the hydrogen production effect. Compared with NiAl-LDH and MoS2, the overpotential of the NM3 electrode is significantly reduced, indicating that NM3 has good hydrogen production performance.
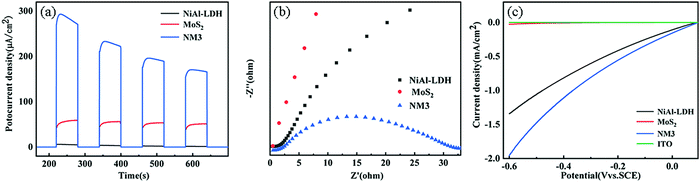 |
| Fig. 6 NiAl-LDH, MoS2 and NM3: (a) IT curves; (b) EIS curves; and (c) LSV curves. | |
3.5.2 UV-vis DRS analysis.
It is an important part to evaluate the performance of catalysts to study the response degree of the catalyst to visible light. In order to study their light absorption capacity, the UV-vis DRS of NiAl-LDH, MoS2 and NM3 were studied. As shown in the Fig. 7(a), NiAl-LDH has three absorption bands in the range of visible light, in the 200–300 nm absorption band was attributable to the ligand to the metal charge transfer (from O 2p orbit to Ni 3dt2g) in 300–500 nm, 600–800 nm visible light range of dual-band attributed to octahedron in the structure (d–d transition of Ni2+).39,51 MoS2 and NMX showed full-spectrum absorption in the visible light range, which was caused by the black MoS2 prepared and the large proportion of MoS2 in the composite catalyst. In addition, the UV-vis DRS data were converted using the formula: αhν = A(hν − Eg)n/2,52 where α is the absorption coefficient and hν is the photon energy, and the band gap values of NiAl-LDH and MoS2 are 2.4 eV and 1.79 eV respectively.
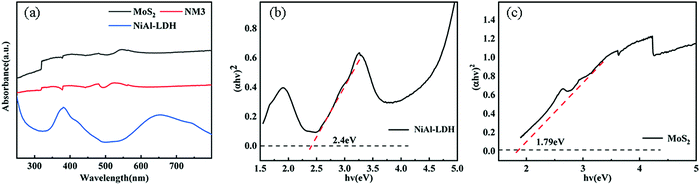 |
| Fig. 7 (a) UV-vis DRS diffuse reflectance spectra of NiAl-LDH, MoS2, and NM3. Band gap plots of (b) NiAl-LDH and (c) MoS2. | |
3.5.3 Structure of energy band.
In order to ensure that H+ can be reduced to H2, the Mott–Schottky (MS) plot was used to test MoS2 and NiAl-LDH. As shown in Fig. 8(a) and (b), the CB potential relative to Ag/AgCl electrode MoS2 and NiAl-LDH was −0.2 V and −0.92 V, respectively. The formula ENHE = EAg/AgCl + 0.197 was used to transform the Ag/AgCl electrode into the standard hydrogen electrode.52,53 It is known that the band gaps of MoS2 and NiAl-LDH are 1.79 eV and 2.4 eV respectively, and the band structure (Fig. 8c) can be obtained.
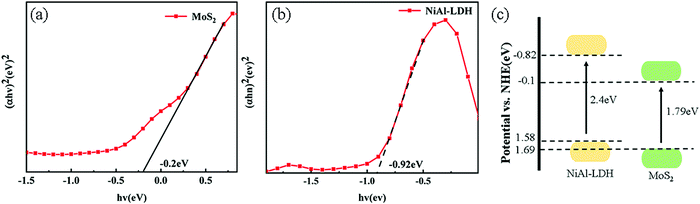 |
| Fig. 8 Mott–Schottky plots for (a) MoS2 and (b) NiAl-LDH. (c) The valence band position. | |
3.5.4 PL analysis.
In order to investigate the separation and transfer efficiency of photogenerated carriers in the hydrogen production process, the PL spectra of different catalysts were tested. Different catalysts have different peak heights. Generally speaking, the lower the peak value of PL, the better the activity of the catalyst and the lower the composite efficiency of photogenerated carriers. Fig. 9(a) shows the PL spectrum of the catalyst when the excitation wavelength is 470 nm. It can be seen that the peak value of NM3 decreases to a large extent compared with that of NiAl-LDH. Fig. 9(b) shows the comparison of peak intensities of different catalysts. It can be observed that the fluorescence quenching intensity of NM3 was the lowest, which proved that the construction of S-scheme heterojunctions can effectively avoid the problem of charge recombination between NiAl-LDH and MoS2, thus improving the photocatalytic hydrogen evolution performance of the composite material.
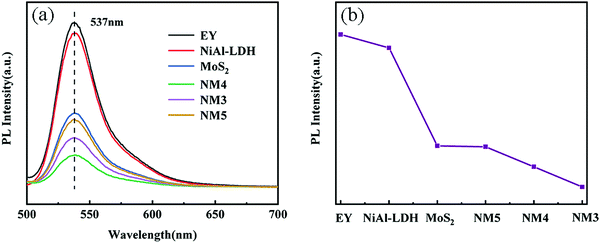 |
| Fig. 9 NiAl-LDH, MoS2, and NMX (X = 3, 4, and 5): (a) PL spectra and (b) PL peak intensity comparison. | |
3.6 Production hydrogen
3.6.1 Photocatalytic activity.
The catalyst was carried out in a reaction system with 10 wt% triethanolamine (TEOA) as the sacrificial agent and EY as the sensitizer. When the pH of TEOA was 10, the hydrogen production of NiAl-LDH, MoS2 and NM3 was compared, and the hydrogen production of NM3 reached 229.5 μmol, which was 200 times and 4 times that of NiAl-LDH and MoS2, respectively. Fig. 10(b) shows the hydrogen production of different ratios of composite catalysts in the EY sensitization system under visible light irradiation for five hours, in which NM3 showed the best hydrogen production effect under visible light irradiation. Fig. 10(c) shows the comparison of hydrogen generation of the NM3 composite catalyst in different pH values of the TEOA solution. It can be seen from the figure that the composite catalyst has the best hydrogen production effect in a mild environment (pH = 10). Excessive acid or alkali environment was not conducive to hydrogen production. Excessive alkali will reduce the content of H+ in the solution, which was not conducive to the hydrogen evolution reaction.54Fig. 10(d) shows the influence of adjusting the amount of EY on hydrogen evolution. It can be seen that when the amount of EY was 24 mg, NM3 has the best effect of hydrogen production, and excessive EY will cover up the active site of the reaction and decrease the hydrogen production capacity. Fig. 10(e) shows the apparent quantum efficiency of NM3 at different wavelengths. When the wavelength is 550 nm, the best apparent quantum efficiency of the sample is 2.78%.
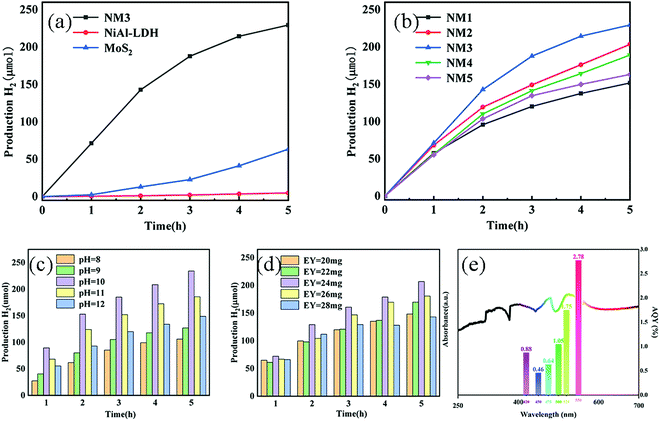 |
| Fig. 10 H2 evolution of (a) NiAl-LDH, MoS2 and NM3; (b) NMX (X = 1–5); (c) EY affected of H2 evolution; (d) pH (TEOA) affected of H2 evolution; and (e) apparent quantum efficiency (AQE) of NM3. | |
3.6.2 Stability experiments.
Whether the catalyst is stable or not is an important part of evaluating its performance. For this, we carried out a hydrogen evolution cycle test. After 5 hours of hydrogen production, the catalyst in the quartz bottle was filtered and recovered, and then the new EY and sacrificial agent were added. It can be seen that the hydrogen production effect has decreased, which may be due to the loss of catalyst in the process of extraction and filtration, resulting in a decrease in the effect. As can be seen from Fig. 11(b), the XRD pattern before and after the reaction did not change significantly. Fig. 11(c) and (d) show SEM images before and after the reaction, with no significant change in size, which could clearly display the structure of nanometer flowers. In conclusion, the composite material NM3 has good stability.
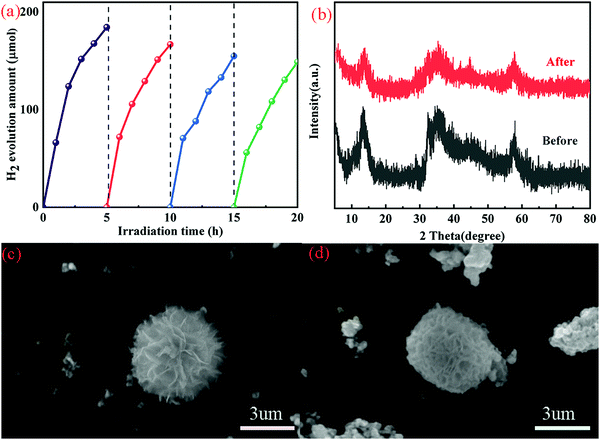 |
| Fig. 11 (a) Cycling test. (b) XRD patterns of NM3 before and after the reaction. SEM of NM3 (c) before the reaction and (d) after the reaction. | |
3.7 Photocatalytic reaction mechanism
Based on the above-mentioned results, the values of CB and VB of NiAl-LDH and MoS2 were determined, as shown in Fig. 8(a). Two kinds of semiconductors with the construction of heterojunctions can be divided into type II heterojunction or S-scheme heterojunction. Assuming that the type II heterojunction was constructed, the photogenerated electrons and holes move to the positions of NiAl-LDH and CB and VB of MoS2 respectively, and the electrons react on CB of MoS2 to generate H2. On the downside, the CB of MoS2 was very low (−0.1 eV), which does not provide sufficient driving force for H2 production. In the process of XPS data processing, the binding energy of S 2p moves to the high and the binding energy of Ni 2p moves to the low, making the electrons move from MoS2 to NiAl-LDH. Based on the analysis of the energy band structure and XPS data, the catalysis mechanism of the S-scheme heterojunction was proposed (Fig. 12). When the two semiconductors are in close contact, the electrons will spontaneously transfer from NiAl-LDH to MoS2 under the influence of the coulombic force. Due to the different positive and negative characteristics of the two semiconductors, an internal electric field is formed at the interface.55,56 Band bending is caused by the transfer and accumulation of electrons. Under the irradiation of visible light, the electrons at the MoS2 CB combine with the holes at the NiAl-LDH VB and consume the useless electrons and holes. The hydrogen evolution process is carried out at the NiAl-LDH CB, and the reaction is carried out by the MoS2 VB.
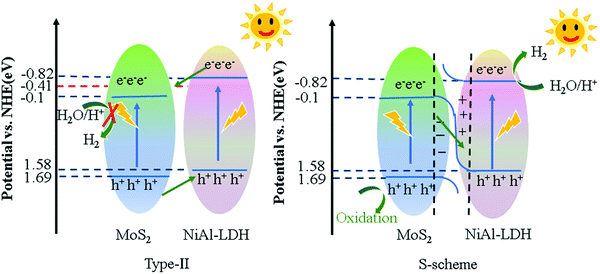 |
| Fig. 12 Reaction mechanism diagram. | |
4 Conclusions
MoS2 and NiAl-LDH have been successfully prepared by a hydrothermal method. The 2D/2D composite MoS2/NiAl-LDH has been synthesized by an ordinary physical mixing method. The S-scheme heterojunction has been constructed according to the band structure and electron transfer of MoS2 and NiAl-LDH. The close contact between MoS2 and NiAl-LDH will cause charge transfer, and the internal electric field will greatly improve the transfer rate of photogenerated carriers. The successful coupling of the two not only accelerates the separation of photogenerated carriers, but also has the minimum resistance in the process of electron transfer, and can avoid charge recombination between NiAl-LDH and MoS2, thus enhancing the effect of hydrogen production. Compared with MoS2 and NiAl-LDH, the MoS2/NiAl-LDH composite showed the highest amount of photocatalytic hydrogen production, which reached 229.5 μmol in 5 h and showed good point stability after a 20 h cycle test. Taken together, this work will provide new insights into the construction of S-type heterojunctions by photocatalysts with a 2D/2D spatial structure.
Conflicts of interest
The authors declare that they have no known competing financial interests or personal relationships that could have appeared to influence the work reported in this paper.
Acknowledgements
This work was financially supported by the Fundamental Research Funds for the Central Universities of North University for Nationalities (2021KJCX02), the Natural Science Foundation of Ningxia Province (2021AAC03180), and also was supported by the Xixia District Science and Technology Plan Project (XXKJ1901).
References
- Y. Ma, X. Wang, Y. Jia, X. Chen, H. Han and C. Li, Titanium Dioxide-Based Nanomaterials for Photocatalytic Fuel Generations, Chem. Rev., 2014, 114(19), 9987–10043 CrossRef CAS PubMed.
- M. A. Idris, Z. A. Z. Jamal, S. B. Jamaludin, N. Nafarizal, A. K. Othman, M. Z. Sahdan, M. P. Zakaria, M. Ionescu and J. Nowotny, Oxide semiconductors for solar to chemical energy conversion: nanotechnology approach, Ionics, 2014, 20(4), 581–592 CrossRef CAS.
- D. Luo, X. Wang, Z. Zhang, D. Gao, Z. Liu and J. Chen, Enhancement of photocatalytic hydrogen evolution from dye-sensitized amide-functionalized carbon nanospheres by superior adsorption performance, Int. J. Hydrogen Energy, 2020, 45(55), 30375–30386 CrossRef CAS.
- J. Pan, Z. Guan, J. Yang and Q. Li, Facile fabrication of ZnIn2S4/SnS2 3D heterostructure for efficient visible-light photocatalytic reduction of Cr(VI), Chin. J. Catal., 2020, 41(1), 200–208 CrossRef CAS.
- Y. Liu, J. Xu, Z. Ding, M. Mao and L. Li, Marigold shaped mesoporous composites Bi2S3/Ni(OH)(2) with n–n heterojunction for high efficiency photocatalytic hydrogen production from water decomposition, Chem. Phys. Lett., 2021, 766 DOI:10.1016/j.cplett.2021.138337.
- Y. Ding, D. Wei, R. He, R. Yuan, T. Xie and Z. Li, Rational design of Z-scheme PtS–ZnIn2S4/WO3–MnO2 for overall photo catalytic water splitting under visible light, Appl. Catal., B, 2019, 258 DOI:10.1016/j.apcatb.2019.117948.
- F. He, A. Meng, B. Cheng, W. Ho and J. Yu, Enhanced photocatalytic H-2-production activity of WO3/TiO2 step-scheme heterojunction by graphene modification, Chin. J. Catal., 2020, 41(1), 9–20 CrossRef CAS.
- K. Wang, B. Liu, J. Li, X. Liu, Y. Zhou, X. Zhang, X. Bi and X. Jiang,
In situ synthesis of TiO2 nanostructures on Ti foil for enhanced and stable photocatalytic performance, J. Mater. Sci. Technol., 2019, 35(4), 615–622 CrossRef.
- A. Fujishima and K. Honda, Electrochemical photolysis of water at a semiconductor electrode, Nature, 1972, 238(5358), 37–38 CrossRef CAS.
- A. Le Goff, V. Artero, B. Jousselme, P. D. Tran, N. Guillet, R. Metaye, A. Fihri, S. Palacin and M. Fontecave, From hydrogenases to noble metal-free catalytic nanomaterials for H2 production and uptake, Science, 2009, 326(5958), 1384–1387 CrossRef CAS PubMed.
- J. Wan, X. Du, E. Liu, Y. Hu, J. Fan and X. Hu,
Z-Scheme visible-light-driven Ag3PO4 nanoparticle@MoS2 quantum dot/few-layered MoS2 nanosheet heterostructures with high efficiency and stability for photocatalytic selective oxidation, J. Catal., 2017, 345, 281–294 CrossRef CAS.
- Y. Wang, X. Ye, G. Chen, D. Li, S. Meng and S. Chen, Synthesis of BiPO4 by crystallization and hydroxylation with boosted photocatalytic removal of organic pollutants in air and water, J. Hazard. Mater., 2020, 399 DOI:10.1016/j.jhazmat.2020.122999.
- B. Luo, R. Song and D. Jing, ZnCr LDH nanosheets modified graphitic carbon nitride for enhanced photocatalytic hydrogen production, Int. J. Hydrogen Energy, 2017, 42(37), 23427–23436 CrossRef CAS.
- X. Guo, Z. Fan, Y. Wang and Z. Jin, CeO2 nanoparticles dispersed on CoAl-LDH hexagonal nanosheets as 0D/2D binary composite for enhanced photocatalytic hydrogen evolution, Surf. Interfaces, 2021, 24 DOI:10.1016/j.surfin.2021.101105.
- M. He, S. Hu, C. Feng, H. Wu, H. Liu and H. Mei, Interlaced rosette-like MoS2/Ni3S2/NiFe-LDH grown on nickel foam: a bifunctional electrocatalyst for hydrogen production by urea-assisted electrolysis, Int. J. Hydrogen Energy, 2020, 45(1), 23–35 CrossRef CAS.
- M. Yang, K. Wang, Y. Li, K. Yang and Z. Jin, Pristine hexagonal CdS assembled with NiV LDH nanosheet formed p–n heterojunction for efficient photocatalytic hydrogen evolution, Appl. Surf. Sci., 2021, 548 DOI:10.1016/j.apsusc.2021.149212.
- M. Gong, Y. Li, H. Wang, Y. Liang, J. Z. Wu, J. Zhou, J. Wang, T. Regier, F. Wei and H. Dai, An Advanced Ni–Fe Layered Double Hydroxide Electrocatalyst for Water Oxidation, J. Am. Chem. Soc., 2013, 135(23), 8452–8455 CrossRef CAS.
- Y. Lee, J. H. Choi, H. J. Jeon, K. M. Choi, J. W. Lee and J. K. Kang, Titanium-embedded layered double hydroxides as highly efficient water oxidation photocatalysts under visible light, Energy Environ. Sci., 2011, 4(3), 914–920 RSC.
- Y. Zhao, B. Li, Q. Wang, W. Gao, C. J. Wang, M. Wei, D. G. Evans, X. Duan and D. O’Hare, NiTi-Layered double hydroxides nanosheets as efficient photocatalysts for oxygen evolution from water using visible Light, Chem. Sci., 2014, 5(3), 951–958 RSC.
- N. Baliarsingh, L. Mohapatra and K. Parida, Design and development of a visible light harvesting Ni–Zn/Cr–CO32-LDH system for hydrogen evolution, J. Mater. Chem. A, 2013, 1(13), 4236–4243 RSC.
- J. Chen, C. Wang, Y. Zhang, Z. Guo, Y. Luo and C.-J. Mao, Engineering ultrafine NiS cocatalysts as active sites to boost photocatalytic hydrogen production of MgAl layered double hydroxide, Appl. Surf. Sci., 2020, 506 DOI:10.1016/j.apsusc.2019.144999.
- S. Nayak and K. M. Parida, Nanostructured CeO2/MgAl-LDH composite for visible light induced water reduction reaction, Int. J. Hydrogen Energy, 2016, 41(46), 21166–21180 CrossRef CAS.
- L. Mohapatra and K. Parida, A review on the recent progress, challenges and perspective of layered double hydroxides as promising photocatalysts, J. Mater. Chem. A, 2016, 4(28), 10744–10766 RSC.
- M. Yang, K. Wang and Z. Jin, Pyramidal CdS Polyhedron Modified with NiAl LDH to Form S-scheme Heterojunction for Efficient Photocatalytic Hydrogen Evolution, ChemCatChem, 2021, 13(15), 3525–3535 CrossRef CAS.
- A. Kumaresan, S. Yang, K. Zhao, N. Ahmad, J. Zhou, Z. Zheng, Y. Zhang, Y. Gao, H. Zhou and Z. Tang, Facile development of CoAl-LDHs/RGO nanocomposites as photocatalysts for efficient hydrogen generation from water splitting under visible-light irradiation, Inorg. Chem. Front., 2019, 6(7), 1753–1760 RSC.
- J. Zhang, Q. Zhu, L. Wang, M. Nasir, S.-H. Cho and J. Zhang, g-C3N4/CoAl-LDH 2D/2D hybrid heterojunction for boosting photocatalytic hydrogen evolution, Int. J. Hydrogen Energy, 2020, 45(41), 21331–21340 CrossRef CAS.
- K. Zhuge, Z. Chen, Y. Yang, J. Wang, Y. Shi and Z. Li,
In situ photodeposition of MoS2 onto CdS quantum dots for efficient photocatalytic H-2 evolution, Appl. Surf. Sci., 2021, 539 DOI:10.1016/j.apsusc.2020.148234.
- Y. Lei, J. Hou, F. Wang, X. Ma, Z. Jin, J. Xu and S. Min, Boosting the catalytic performance of MoSx cocatalysts over CdS nanoparticles for photocatalytic H-2 evolution by Co doping via a facile photochemical route, Appl. Surf. Sci., 2017, 420, 456–464 CrossRef CAS.
- H. Peng, J. Lu, C. Wu, Z. Yang, H. Chen, W. Song, P. Li and H. Yin, Co-doped MoS2 NPs with matched energy band and low overpotential high efficiently convert CO2 to methanol, Appl. Surf. Sci., 2015, 353, 1003–1012 CrossRef CAS.
- C. Feng, Z. Chen, J. Hou, J. Li, X. Li, L. Xu, M. Sun and R. Zeng, Effectively enhanced photocatalytic hydrogen production performance of one-pot synthesized MoS2 clusters/CdS nanorod heterojunction material under visible light, Chem. Eng. J., 2018, 345, 404–413 CrossRef CAS.
- W.-J. Xie, X. Li and F.-J. Zhang, Mo-vacancy induced high performance for photocatalytic hydrogen production over MoS2 nanosheets cocatalyst, Chem. Phys. Lett., 2020, 746 DOI:10.1016/j.cplett.2020.137276.
- G. Ye, Y. Gong, J. Lin, B. Li, Y. He, S. T. Pantelides, W. Zhou, R. Vajtai and P. M. Ajayan, Defects Engineered Monolayer MoS2 for Improved Hydrogen Evolution Reaction, Nano Lett., 2016, 16(2), 1097–1103 CrossRef CAS PubMed.
- X. Liu, X. Chen, L. Xu, B. Wu, X. Tu, X. Luo, F. Yang and J. Lin, Non-noble metal ultrathin MoS2 nanosheets modified Mn0.2Cd0.8S heterostructures for efficient photocatalytic H-2 evolution with visible light irradiation, Int. J. Hydrogen Energy, 2020, 45(51), 26770–26784 CrossRef CAS.
- X. Zhang, Y. Guo, J. Tian, B. Sun, Z. Liang, X. Xu and H. Cui, Controllable growth of MoS2 nanosheets on novel Cu2S snowflakes with high photocatalytic activity, Appl. Catal., B, 2018, 232, 355–364 CrossRef CAS.
- X. Liu, B. Wang, M. Liu, S. Liu, W. Chen, L. Gao and X. Li,
In situ growth of vertically aligned ultrathin MoS2 on porous g-C3N4 for efficient photocatalytic hydrogen production, Appl. Surf. Sci., 2021, 554 DOI:10.1016/j.apsusc.2021.149617.
- J. Wang, G. Wang, B. Cheng, J. Yu and J. Fan, Sulfur-doped g-C3N4/TiO2 S-scheme heterojunction photocatalyst for Congo Red photodegradation, Chin. J. Catal., 2021, 42(1), 56–68 CrossRef CAS.
- X. Li, J. Liu, J. Huang, C. He, Z. Feng, Z. Chen, L. Wan and F. Deng, All Organic S-Scheme Heterojunction PDI-Ala/S-C3N4 Photocatalyst with Enhanced Photocatalytic Performance, Acta Phys.-Chim. Sin., 2021, 37(6) DOI:10.3866/PKU.WHXB202010030.
- F. Mukhtar, T. Munawar, M. S. Nadeem, M. N. U. Rehman, M. Riaz and F. Iqbal, Dual S-scheme heterojunction ZnO–V2O5–WO3 nanocomposite with enhanced photocatalytic and antimicrobial activity, Mater. Chem. Phys., 2021, 263 DOI:10.1016/j.matchemphys.2021.124372.
- W.-K. Jo, Y.-G. Kim and S. Tonda, Hierarchical flower-like NiAl-layered double hydroxide microspheres encapsulated with black Cu-doped TiO2 nanoparticles: highly efficient visible-light-driven composite photocatalysts for environmental remediation, J. Hazard. Mater., 2018, 357, 19–29 CrossRef CAS PubMed.
- Y. Qin, H. Li, J. Lu, Y. Yan, Z. Lu and X. Liu, Enhanced photocatalytic performance of MoS2 modified by AgVO3 from improved generation of reactive oxygen species, Chin. J. Catal., 2018, 39(9), 1470–1483 CrossRef CAS.
- H. Li, J. Li, C. Xu, P. Yang, D. H. L. Ng, P. Song and M. Zuo, Hierarchically porous MoS2/CoAl-LDH/HCF with synergistic adsorption-photocatalytic performance under visible light irradiation, J. Alloys Compd., 2017, 698, 852–862 CrossRef CAS.
- T. Wang, Y. Zhang, Y. Wang, J. Zhou, L. Wu, Y. Sun, X. Xu, W. Hou, X. Zhou, Y. Du and W. Zhong, Alumina-Supported CoPS Nanostructures Derived from LDH as Highly Active Bifunctional Catalysts for Overall Water Splitting, ACS Sustainable Chem. Eng., 2018, 6(8), 10087–10096 CrossRef CAS.
- J.-Y. He, D. Zhang, X.-J. Wang, J. Zhao, Y.-P. Li, Y. Liu and F.-T. Li, Phosphorylation of NiAl-layered double hydroxide nanosheets as a novel cocatalyst for photocatalytic hydrogen evolution, Int. J. Hydrogen Energy, 2021, 46(36), 18977–18987 CrossRef CAS.
- Y. Fu, Z. Ren, J. Wu, Y. Li, W. Liu, P. Li, L. Xing, J. Ma, H. Wang and X. Xue, Direct Z-scheme heterojunction of ZnO/MoS2 nanoarrays realized by flowing-induced piezoelectric field for enhanced sunlight photocatalytic performances, Appl. Catal., B, 2021, 285 DOI:10.1016/j.apcatb.2020.119785.
- S. Zang, G. Zhang, Z.-A. Lan, D. Zheng and X. Wang, Enhancement of photocatalytic H-2 evolution on pyrene-based polymer promoted by MoS2 and visible light, Appl. Catal., B, 2019, 251, 102–111 CrossRef CAS.
- X. Xin, Y. Song, S. Guo, Y. Zhang, B. Wang, Y. Wang and X. Li, One-step synthesis of P-doped MoS2 for efficient photocatalytic hydrogen production, J. Alloys Compd., 2020, 829 DOI:10.1016/j.jallcom.2020.154635.
- B. Lv, X. Feng, X. Xi, X. Feng, Z. Yuan, Y. Yang and F. Zhang, Noble-metal-free Cd0.3Zn0.7S–Ni(OH)(2) for high efficiency visible light photocatalytic hydrogen production, J. Colloid Interface Sci., 2021, 601, 177–185 CrossRef CAS.
- B. Debnath, S. Dhingra, V. Sharma, V. Krishnan and C. M. Nagaraja, Efficient photocatalytic generation of hydrogen by twin Zn0.5Cd0.5S nanorods decorated with noble metal-free co-catalyst and reduction of 4-nitrophenol in water, Appl. Surf. Sci., 2021, 550 DOI:10.1016/j.apsusc.2021.149367.
- S. Zhao, J. Xu, Z. Li, Z. Liu and Y. Li, Molybdenum disulfide coated nickel–cobalt sulfide with nickel phosphide loading to build hollow core-shell structure for highly efficient photocatalytic hydrogen evolution, J. Colloid Interface Sci., 2019, 555, 689–701 CrossRef CAS PubMed.
- Y. Xiao, Z. Ji, C. Zou, Y. Xu, R. Wang, J. Wu, G. Liu, P. He, Q. Wang and T. Jia, Construction of CeO2/BiOI S-scheme heterojunction for photocatalytic removal of elemental mercury, Appl. Surf. Sci., 2021, 556 DOI:10.1016/j.apsusc.2021.149767.
- X. Yan and Z. Jin, Interface engineering: NiAl-LDH in situ derived NiP2 quantum dots and Cu3P nanoparticles ingeniously constructed p–n heterojunction for photocatalytic hydrogen evolution, Chem. Eng. J., 2020, 420 DOI:10.1016/j.cej.2020.127682.
- L. Li, J. Xu, J. Ma, Z. Liu and Y. Li, A bimetallic sulfide CuCo2S4 with good synergistic effect was constructed to drive high performance photocatalytic hydrogen evolution, J. Colloid Interface Sci., 2019, 552, 17–26 CrossRef CAS PubMed.
- L. Li and J. Xu, Rare earth perovskite modified cobalt disulfide catalysts controlled by reaction solvent synthesis to form a p–n heterojunction, Appl. Surf. Sci., 2019, 505 DOI:10.1016/j.apsusc.2019.143937.
- L. Zhang, G. Wang, X. Hao, Z. Jin and Y. Wang, MOFs-derived Cu3P@CoP p–n heterojunction for enhanced photocatalytic hydrogen evolution, Chem. Eng. J., 2020, 395 DOI:10.1016/j.cej.2020.125113.
- T. Liu, K. Yang, H. Gong and Z. Jin, Visible-light driven S-scheme Mn0.2Cd0.8S/CoTiO3 heterojunction for photocatalytic hydrogen evolution, Renewable Energy, 2021, 173, 389–400 CrossRef CAS.
- J. Tao, X. Yu, Q. Liu, G. Liu and H. Tang, Internal electric field induced S-scheme heterojunction MoS2/CoAl LDH for enhanced photocatalytic hydrogen evolution, J. Colloid Interface Sci., 2021, 585, 470–479 CrossRef CAS.
Footnote |
† Electronic supplementary information (ESI) available. See DOI: 10.1039/d1nj04728k |
|
This journal is © The Royal Society of Chemistry and the Centre National de la Recherche Scientifique 2022 |