DOI:
10.1039/D1NJ04676D
(Paper)
New J. Chem., 2022,
46, 212-220
Photo- and electro-luminescence properties of two coumarin-triarylimidazole hybrid derivatives
Received
30th September 2021
, Accepted 19th November 2021
First published on 20th November 2021
Abstract
Two coumarin-triarylimidazole hybrid derivatives with an N,N-diethylamine at the 7-position and a triaryl-substituted imidazole at the 3-position on the coumarin core, 3-(1-(4-(tert-butyl)phenyl)-4,5-diphenyl-1H-imidazol-2-yl)-7-(diethylamino)-2H-chromen-2-one (C-IM-Bu) and 3-(1-(4-cyanophenyl)-4,5-diphenyl-1H-imidazol-2-yl)-7-(diethylamino)-2H-chromen-2-one (C-IM-CN), were successfully synthesized for application in organic-light emitting devices. Their photophysical and electrochemical properties, thermal stability and electroluminescence properties were investigated. The results showed that these compounds have excellent thermal stability, and exhibit strong blue-green emission in dichloromethane solution and green emission in thin solid films. Further, the solution-processed doped devices based on the compounds were fabricated, in which the devices fabricated from C-IM-CN exhibited the best device performance with a turn-on voltage of 3.6 V, a maximum brightness of 6896 cd m−2, a maximum current efficiency of 6.39 cd A−1 and a maximum external quantum efficiency of 2.92%.
1. Introduction
Many coumarin derivatives are well-known as highly efficient luminescent materials and have been extensively used in different application areas such as fluorescent chemosensors,1 bio-imaging,2 organic light-emitting devices (OLEDs),3–10 dye-sensitized solar cells11–15 and optical brighteners.16,17 The photophysical properties of coumarin derivatives strongly depend on their molecular structures.18–20 Substitutions of the electron donating and electron accepting groups on the coumarin moiety can reduce the energy band gaps of the coumarins, leading to redshifted absorption and emission.21,22 For example, coumarin itself showed a very weak blue emission with a peak at 430 nm,23 but the coumarin derivatives with electron-donor groups in the 6- or 7-position or electron-withdrawing groups in the 3- or 4-position of the coumarin skeleton exhibited a bathochromic and intensive emission due to the enhanced intramolecular charge transfer (ICT).24,25 In particular, grafting one electron-donating group and one electron-withdrawing group on the 7- and 3-positions of the coumarin ring, respectively, to form a push–pull electron effect, could greatly enhance the fluorescence properties of coumarins. However, coumarins often suffer from the concentration quenching effect or the aggregation-caused quenching (ACQ) effect, and usually exhibit weak fluorescence emission, even becoming nearly nonemissive in the aggregate or solid state.26,27 In order to reduce the self-aggregation quenching of coumarin derivatives at high concentration or in the solid state, grafting sterically hindered substituents onto the coumarin skeleton is an effective method for preparing highly efficient OLEDs.3,7,28
In recent years, imidazole compounds have been often used in the construction of organic optoelectronic functional materials for OLEDs due to their bipolar characteristics, which have high photoluminescence quantum yields, high stability, high triplet energy and pure blue emission.29–31 The imidazole unit is an electron-deficient heterocyclic five-membered ring, in which two different nitrogen atoms (N1 and N3) exhibit pyrrole-like electron-rich properties and pyridine-like electron-deficient properties, respectively.32,33 The photophysical properties of imidazole derivatives are easily optimized using chemical modification for suitable OLED materials, such as electron transporters, emitters and host materials. In this paper, two new coumarin-triarylimidazole hybrid derivatives (C-IM-Bu and C-IM-CN) were synthesized by grafting different triaryl-substituted imidazole groups at the 3-position on the coumarin core for application in OLEDs. In these molecules, the N,N-diethylamine at the 7-position of the coumarin core is chosen as an electron-donating group, while different triaryl-substituted imidazole units at the 3-position on the coumarin core serve as the electron-withdrawing segments. By attaching the triaryl-substituted imidazole units with push or pull electronic abilities to the coumarin core, we investigated the differences in their photophysical properties and EL performance.
2. Experimental
2.1 Materials and methods
4-(Diethylamino)-2-hydroxybenzaldehyde, 4-aminobenzonitrile, benzyl and 4-tert-butylaniline were obtained from Energy Chemical (China). All the other reactants and solvents were obtained from commercial sources. The preparation of the intermediate, 7-(diethylamino)coumarin-3-carbaldehyde, was described in our previous paper.9
The NMR spectra (1H and 13C) were measured on a Bruker 500. The mass spectra were recorded using a Bruker Esquire 3000 mass spectrometer. Thermogravimetric analyses (TGA) were performed on a Shimadzu thermal analyzer. The UV-vis absorption spectra were recorded on a Shimadzu UV-2550 spectrometer. The cyclic voltammograms were performed on an electrochemical analyzer (CHI Instruments 760 B). At room temperature, using an Edinburgh instrument FLS920 integrating sphere and a Xe lamp, the photoluminescence quantum yields of the compounds were measured using an absolute method. The photoluminescence decay lifetime was measured using a time-correlated single photon counting spectrometer (Edinburgh Instruments FLS920) with a microsecond flashlamp as the excitation source (repetition rate 90 Hz) at room temperature.
2.2 Synthesis and characterization of the compounds
C-IM-Bu
.
Under a N2 atmosphere, a mixture of benzil (0.86 g, 4.09 mmol), 4-tert-butylaniline (0.76 g, 5.09 mmol), 7-(diethylamino)coumarin-3-carbaldehyde (1.00 g, 4.08 mmol) and NH4OAc (3.80 g, 49.30 mmol) was dissolved in AcOH (120 mL) and stirred at 120 °C for 16 h. After cooling to room temperature, the reaction mixture was poured into water (150 mL) and extracted with dichloromethane (3 × 100 mL). The organic phase was washed with water (2 × 100 mL) and dried over anhydrous Na2SO4. After removal of the solvent, the residue was purified using silica gel column chromatography using dichloromethane/ethyl acetate (10
:
1, v/v) as the eluent to obtain a yellow solid (1.21 g, 52%). M.p. > 260 °C. 1H NMR (500 MHz, CDCl3, δ, ppm): 7.98 (s, 1H), 7.53 (d, J = 7.6 Hz, 2H), 7.27 (d, J = 8.9 Hz, 2H), 7.25–7.17 (m, 7H), 7.10 (d, J = 8.2 Hz, 2H), 7.02 (m, J = 8.7 Hz, 2H), 6.57 (d, J = 8.8 Hz, 1H), 6.42 (s, 1H), 3.41 (q, J = 7.2 Hz, 4H), 1.23 (s, 9H), 1.20 (t, J = 7.1 Hz, 6H). 13C NMR (126 MHz, CDCl3, δ, ppm): 160.0, 157.1, 151.1, 150.9, 145.6, 143.2, 138.2, 134.5, 134.0, 131.0, 130.8, 130.6, 129.5, 128.2, 128.1, 127.8, 127.6, 127.3, 126.5, 125.5, 108.9, 108.3, 97.1, 44.7, 34.5, 31.2, 29.7, 26.9, 12.4. HRMS: calcd for C38H37N3O2, 568.2959 [M + H]+; found: 568.2957. Error = 0.35 ppm. Anal. calcd for C38H37N3O2: C, 80.39; H, 6.57; N, 7.40; found: C, 80.45; H, 6.53; N, 7.42.
C-IM-CN
.
7-(Diethylamino)coumarin-3-carbaldehyde (1.00 g, 4.08 mmol), benzil (0.86 g, 4.09 mmol), 4-aminobenzonitrile (1.94 g, 16.42 mmol) and NH4OAc (1.58 g, 20.50 mmol) were mixed with 60 mL of acetic acid in a three-neck round bottom flask (250 mL), and the mixture was stirred at 120 °C for 4 h. After cooling, the reaction mixture was poured into water (150 mL) and extracted with dichloromethane (3 × 100 mL). The organic phase was washed with water (2 × 100 mL) and dried over anhydrous Na2SO4. After the solvent was removed under vacuum, the residue was purified using silica gel column chromatography using dichloromethane/ethyl acetate (14
:
1, v/v) as the eluent to a obtain yellow solid (1.20 g, 55%). M.p. > 260 °C. 1H NMR (500 MHz, DMSO-d6, δ, ppm): 8.19 (s, 1H), 7.66 (d, J = 8.6 Hz, 3H), 7.45 (d, J = 8.8 Hz, 1H), 7.35 (d, J = 7.5 Hz, 2H), 7.27–7.22 (m, 5H), 7.17–7.07 (m, 4H), 6.65 (d, J = 8.8 Hz, 1H), 6.38 (s, 1H), 3.34 (q, J = 6.8 Hz, 4H), 1.01 (t, J = 7.2 Hz, 6H). 13C NMR (126 MHz, DMSO-d6, δ, ppm): 159.4, 157.1, 151.8, 147.2, 143.3, 141.0, 137.7, 134.4, 133.7, 133.3, 131.4, 130.8, 130.4, 130.2, 129.3, 129.2, 129.0, 128.7, 127.2, 126.9, 119.4, 118.5, 111.2, 110.6, 110.5, 110.1, 110.0, 107.9, 96.8, 44.7, 12.7. HRMS: Calcd for C35H28N4O2, 537.2285 [M + H]+; Found: 537.2288. Error = 0.56 ppm. Anal. calcd for C35H28N4O2: C, 78.34; H, 5.26; N, 10.44; found: C, 78.27; H, 5.29; N, 10.41.
3. Results and discussion
3.1 Synthesis
The synthetic routes and procedures for C-IM-Bu and C-IM-CN are shown in Scheme 1 and the experimental section. The key intermediate, 7-(diethylamino)coumarin-3-carbaldehyde, was prepared according to the method described in our previous paper.9 The compounds C-IM-Bu and C-IM-CN were synthesized via the one-pot multi-component reaction of the corresponding aromatic amine (4-tert-butylaniline or 4-aminobenzonitrile) with 7-(diethylamino)coumarin-3-carbaldehyde and benzil in ammonium acetate/acetic acid as the medium in moderate yield (>50%) after purification using column chromatography. The structures of the compounds were confirmed using 1H and 13C NMR and high-resolution mass spectrometry. All compounds can be dissolved in common organic solvents, such as tetrahydrofuran, dichloromethane, chloroform, toluene and DMF.
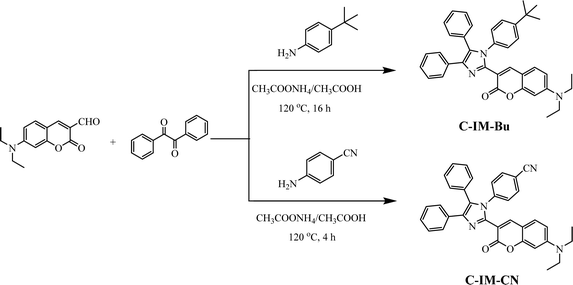 |
| Scheme 1 Synthetic routes and molecular structures of C-IM-Bu and C-IM-CN. | |
3.2 Photophysical, electrochemical properties and thermal stabilities of the compounds
The UV-vis absorption and photoluminescence (PL) spectra of C-IM-Bu and C-IM-CN in a CH2Cl2 solution and thin films are shown in Fig. 1. The compounds C-IM-Bu and C-IM-CN in a CH2Cl2 solution exhibited similar absorption spectra in the range of 250–500 nm. The maximum absorption bands of C-IM-Bu and C-IM-CN were located at 405 and 415 nm, respectively, which were attributed to the π → π* transition of the characteristic vibrational structure of the 7-diethylamino-coumarin skeleton,5,6,10 indicating that the absorption band of C-IM-CN was red-shifted with the increasing electron-withdrawing ability of the aromatic imidazole group. The UV-vis absorption edges of C-IM-Bu and C-IM-CN in the CH2Cl2 solutions were estimated to be at 503 and 510 nm, and the corresponding optical band gaps (Eopt) of C-IM-Bu and C-IM-CN were calculated to be 2.47 eV and 2.43 eV, respectively, from the equation: Eopt = 1241/λonset.
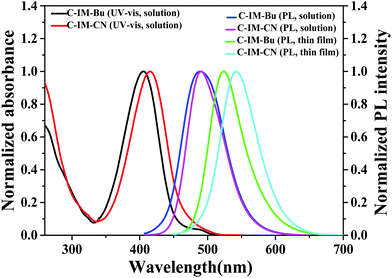 |
| Fig. 1 UV-vis absorption in dichloromethane solutions and the photoluminescence spectra of C-IM-Bu and C-IM-CN in CH2Cl2 solutions and thin solid films. | |
Under excitation at 405 nm and 415 nm, C-IM-Bu and C-IM-CN in a CH2Cl2 solution exhibited blue-green emission and both had maximum peaks at 490 nm. Compared with their PL spectra in CH2Cl2 solution, the PL spectra of C-IM-Bu and C-IM-CN in the thin solid films exhibited significant bathochromic shifts due to interaction and aggregation between the molecules, and these compounds in the thin solid films exhibited green emission with maximum peaks at 524 and 542 nm, respectively. Additionally, the fluorescence quantum yields (Φf) of C-IM-Bu and C-IM-CN were measured to be 81.0 and 81.4% in CH2Cl2 solutions at room temperature, respectively, and their fluorescence decay lifetimes (τ) were measured to be 6.05 and 6.04 ns.
The compounds C-IM-Bu and C-IM-CN possess the D–π–A system consisting of a diethylamino group as the donor at the 7-position and different N-phenyl imidazole units as the acceptor at the 3-position of the coumarin skeleton, which should have certain intramolecular charge transfer (ICT) characteristics. In order to further investigate the intramolecular charge transfer (ICT) properties of C-IM-Bu and C-IM-CN, the effect of solvent polarity on the photoluminescence of the compounds was investigated in four different solvents from non-polar toluene to highly polar DMF (Fig. 2). With the increase of solvent polarity, the maximum emission peak of C-IM-Bu in toluene, acetone and DMF was shifted from 473 nm to 482 nm, and the maximum emission peak of C-IM-CN in toluene, acetone and DMF was shifted from 475 nm to 486 nm. Compared with other solvents, the compounds C-IM-Bu and C-IM-CN exhibited a maximum emission peak at 490 nm, with significant red-shifts of 17 nm and 15 nm, respectively, compared to their maximum emission peaks in toluene. All the compounds C-IM-Bu and C-IM-CN exhibit red-shifted emission from the non-polar (toluene) to the highly polar (DMF) solvents, which may be mainly due to the fact that the excited state of the compounds are controlled by the dipole–dipole interaction and solute–solvent interaction.34 The corresponding CIE chromaticity diagrams of the compounds in different solvents are shown in Fig. 2 (insets).
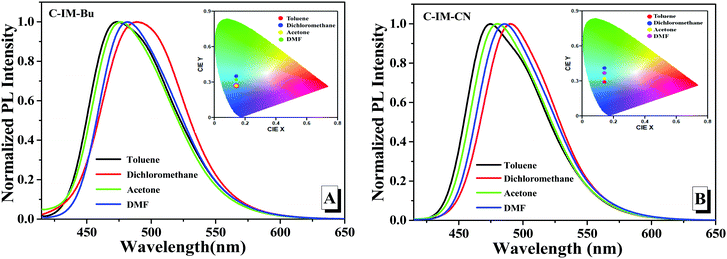 |
| Fig. 2 Photoluminescence spectra and the chromaticity coordinates (insets) of C-IM-Bu (A) and C-IM-CN (B) in different solvents. | |
The PL spectra of C-IM-Bu and C-IM-CN at different concentrations (10−7–10−3 mol L−1) were investigated in a CH2Cl2 solution (Fig. 3). As shown in Fig. 3, the PL spectra of the compounds exhibited the same pattern of change within the whole process of concentration changes. At lower than 1.0 × 10−7 mol L−1, the emission bands of the compounds were very weak. With the concentration increasing, the relative emission intensity of the compounds gradually increased, and the emission intensity of the compounds reached the maximum when the concentration was 1.0 × 10−5 mol L−1. At over 1.0 × 10−5 mol L−1, the emission intensity of the compounds decreased due to the intermolecular aggregation. Furthermore, with the increase of the concentration from 10−7 mol L−1 to 10−3 mol L−1, the emission peak of C-IM-Bu was shifted from 480 nm to 517 nm, and the emission peak of C-IM-CN was shifted from 528 nm to 540 nm (insets of Fig. 3), respectively, which could be attributed to the intermolecular aggregation at high concentrations.
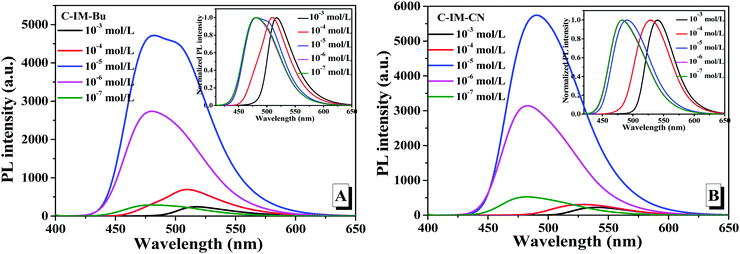 |
| Fig. 3 Fluorescence spectra of C-IM-Bu (A) and C-IM-CN (B) in different concentrations of dichloromethane solution. | |
The electrochemical properties of the compounds were measured in a CH2Cl2 solution using cyclic voltammetry (Fig. 4), in which a 0.10 mol L−1 solution of Bu4NPF6 in CH2Cl2 was used as the electrolyte, the glassy carbon electrode was used as the working electrode, and the Pt wire and Ag/Ag+ electrode were used as the counter electrode and reference electrode, respectively. As shown in Fig. 4, the first oxidation potentials of C-IM-Bu and C-IM-CN were about 1.11 and 1.15 V, respectively. Under the same conditions, the measured Fc/Fc+ potential was 0.52 V. Thus, the highest occupied molecular orbital (HOMO) energy levels of C-IM-Bu and C-IM-CN were calculated to be −5.39 and −5.43.10 According to the equation: ELUMO = (EHOMO + Eoptg) (eV), the lowest unoccupied molecular orbital (LUMO) energy levels of C-IM-Bu and C-IM-CN were calculated to be −2.92 and −3.00 eV, respectively.
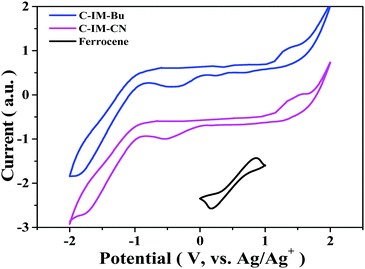 |
| Fig. 4 Cyclic voltammograms of ferrocene and the compounds C-IM-Bu and C-IM-CN (scan rate: 10 mV s−1; solvent: dichloromethane). | |
Fig. 5 shows the thermogravimetric analysis (TGA) and differential thermal analysis (DTA) curves of C-IM-Bu and C-IM-CN, which were measured under a N2 atmosphere at a heating rate of 10 °C min−1. From their TGA curves, C-IM-Bu and C-IM-CN exhibited good thermal stability, and their decomposition temperatures (Td, corresponding to a 3% weight loss) were about 382 and 401 °C, respectively. From their DTA curves, the melting temperatures (Tm) of C-IM-Bu and C-IM-CN were about 247 and 303 °C.
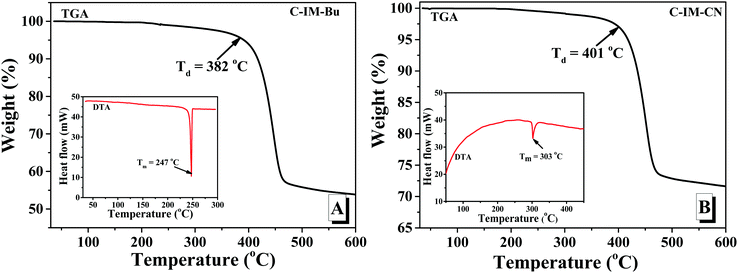 |
| Fig. 5 Thermogravimetric and differential thermal (inset) analyses of C-IM-Bu (A) and C-IM-CN (B). | |
The key parameters of the photophysical, electrochemical and thermal properties of C-IM-Bu and C-IM-CN are summarized in Table 1.
Table 1 Photophysical, thermal and electrochemical properties of the compounds
Compound |
UV-visa (nm) |
PL/PLa,b (nm) |
T
d (°C) |
T
m (°C) |
Φ
f
(%) |
τ (ns) |
E
OX (V) |
E
opt (eV) |
HOMO/LUMO(eV) |
In a dichloromethane solution.
In thin solid films.
|
C-IM-Bu
|
405 |
490/524 |
382 |
247 |
81.0 |
6.05 |
1.11 |
2.47 |
−5.39/−2.92 |
C-IM-CN
|
415 |
490/542 |
401 |
303 |
81.4 |
6.04 |
1.15 |
2.43 |
−5.43/−3.00 |
3.3 Theoretical calculations of the compounds
In order to understand the electronic structure of the compounds in depth, density functional theory (DFT) calculations were carried out using the B3LYP/6-31G(d) basis set. The optimized structures and the electronic distributions of the HOMO and LUMO orbitals for C-IM-Bu and C-IM-CN are depicted in Fig. 6. In the HOMO orbitals of C-IM-Bu and C-IM-CN, besides the absence of electron-density distributions on the (tert-butyl)benzene and (cyano)phenyl groups connected to the N1-position of the imidazole rings, the electron density is completely distributed throughout the molecular skeletons. However, the electron-density distributions in the LUMO orbitals vary for the two compounds. Most of the electron density in the LUMO orbital of C-IM-Bu is distributed in the 7-diethylamino-coumarin skeleton, and only a very small fraction of the electron density is located in the imidazole ring. There are no electron-density distributions in the three phenyl groups attached to the imidazole ring. For C-IM-CN, most of the electron density in the LUMO orbital is completely located on the 7-diethylamino-coumarin skeleton and the (cyano)phenyl group connected to the N1-position of the imidazole ring, and a very small part of the electron density is located on the imidazole ring. There are no electron-density distributions in the two phenyl groups attached to the imidazole ring.
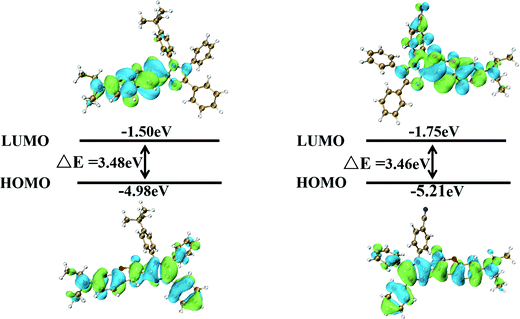 |
| Fig. 6 The spatial electron distributions of the HOMO and LUMO energy densities of C-IM-Bu and C-IM-CN. | |
3.4 Electroluminescence performance of the compounds
The electroluminescence (EL) performance of the compounds were investigated by fabricating the doped devices with a device structure of ITO/PEDOT:PSS (30 nm)/TBADN:Coumarin derivative (x wt%) (25 nm)/TPBi (35 nm)/Liq (2 nm)/Al (150 nm). Poly(3,4-ethylenedioxythiophene) doped with poly(styrenesulfonate) (PEDOT:PSS) was spin-coated onto precleaned and O2-plasma-treated indium tin oxide (ITO) substrates, yielding a layer ca. 30 nm thick. The PEDOT:PSS layer was heated at 140 °C for 15 min to remove the residual water, to obtain a hole injection/transport layer. Blends of the coumarin derivative and TBADN (9,10-bis(2-naphthyl)-2-t-butylanthracene) in a chlorobenzene solution were spin-coated on top of the PEDOT:PSS layer, yielding films ca. 25 nm thick for use as the emitting layer, and then the sample was dried at 50 °C for 30 min. A TPBi electron transport/hole blocking layer (35 nm) was deposited via thermal evaporation at a rate of ∼2 Å s−1. A cathode composed of an ultra-thin Liq interface layer with a thickness of 2 nm and an Al layer with a thickness of about 150 nm was deposited using thermal evaporation. The deposition rates for Liq and Al were ∼0.5 and 4 Å s−1, respectively.
The device configuration and the corresponding HOMO and LUMO energy levels of the materials are given in Fig. 7. The EL spectra of the devices fabricated from the compounds at different doping concentrations are shown in Fig. 8. The current density–voltage–luminance (I–V–L) characteristics and current/power efficiency versus luminance curves of the devices based on the compounds are shown in Fig. 9 and 10, respectively, and the corresponding numerical parameters are listed in Table 2.
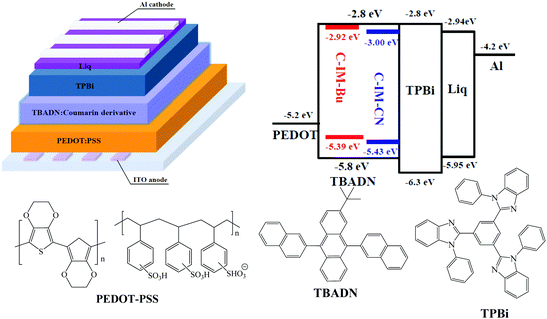 |
| Fig. 7 Schematic diagram of the doped device (upper left), the relative HOMO/LUMO energy levels of the materials (upper right) and the structures of PEDOT: PSS, TBADN and TPBi (bottom). | |
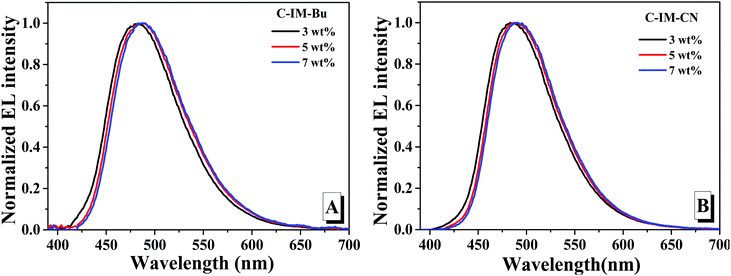 |
| Fig. 8 The EL spectra of the devices based on C-IM-Bu (A) and C-IM-CN (B) with different dopant concentrations. | |
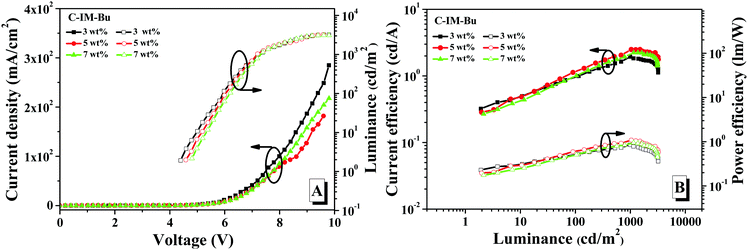 |
| Fig. 9 The current density–voltage-luminance (I–V–L) characteristics (A) and current/power efficiency versus luminance curves (B) of the devices based on C-IM-Bu with different dopant concentrations. | |
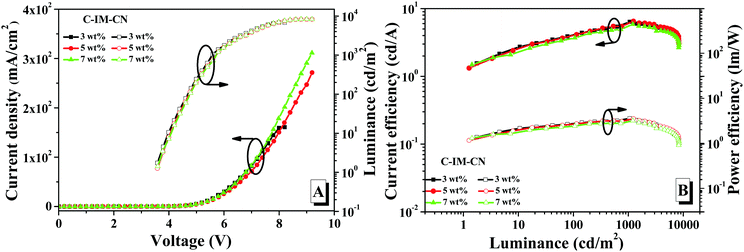 |
| Fig. 10 The current density–voltage–luminance (I–V–L) characteristics (A) and current/power efficiency versus luminance curves (B) of the devices based on C-IM-CN with different dopant concentrations. | |
Table 2 EL performance of the compounds
Compound |
Concentration (wt%) |
V
on (V) |
ELmax (nm) |
L
max (cd m−2) |
CEmax (cd A−1) |
PEmax (lm W−1) |
EQEmax (%) |
C-IM-CBu
|
3 |
4.2 |
482 |
3203 |
1.95 |
0.85 |
0.97 |
5 |
4.6 |
484 |
3222 |
2.50 |
1.09 |
1.16 |
7 |
4.6 |
486 |
3096 |
2.24 |
0.95 |
1.00 |
C-IM-CCN
|
3 |
3.6 |
485 |
6896 |
6.39 |
3.59 |
2.92 |
5 |
3.6 |
487 |
8296 |
6.51 |
3.52 |
2.82 |
7 |
3.6 |
490 |
8345 |
5.87 |
3.19 |
2.47 |
As shown in Fig. 8, the doped devices fabricated from the compounds exhibited blue-green emission, and the EL spectra of the doped devices of the compounds exhibited a slight red-shift when the doping concentrations of the compounds was gradually increased from 3 wt% to 7 wt%. With gradual increases of the doping concentration, the maximum EL emission peak was red-shifted from 482 nm to 486 nm for the compound C-IM-Bu and from 485 nm to 490 nm for the compound C-IM-CN.
As shown in Fig. 9, Fig. 10 and Table 2, it was found that the EL performance of C-IM-CN was better than that of C-IM-CBu. With doping concentration from 3 wt% to 7 wt%, the doped devices of C-IM-CN exhibited maximum luminance (Lmax) values of 6896, 8296 and 8345 cd m−2, and maximum current efficiency (CEmax) values of 6.39, 6.51 and 5.87 cd A−1, and maximum power efficiency (PEmax) values of 3.59, 3.52 and 3.19 lm W−1, and maximum external quantum efficiency (EQEmax) values of 2.92%, 2.82% and 2.47%, respectively. Additionally, the doped devices of C-IM-CN displayed low turn-on voltages (Von) of 3.6 V within the doped concentration range from 3 wt% to 7 wt%. Compared with the doped devices of C-IM-CN, the doped devices of C-IM-Bu not only exhibited very poor EL performance, but also showed higher turn-on voltages (4.2–4.6 V). The doped device with a 5 wt% doping concentration exhibited the best performance: Lmax of 3222 cd m−2, CEmax of 2.50 cd A−1, PEmax of 1.09 lm W−1 and EQEmax of 1.16%, and Von of 4.6 V.
Although the difference between the photoluminescence quantum yields of C-IM-Bu (81.0%) and C-IM-CN (81.4%) was very small, there were significant differences in the electroluminescence performance of the compounds. Compared with C-IM-Bu, the intramolecular charge transfer (ICT) characteristics of C-IM-CN are enhanced due to the strong pull-down electron ability of the benzocyanyl group attached on the N1-position of the imidazole ring. In addition, the C-IM-CN molecules could be linked together end-to-end by C–H⋯N intermolecular hydrogen bonds in the emitting layer due to the presence of the –C
N group in C-IM-CN, forming a relatively disordered network,35–37 which may cause the film forming performance of the emitting layer to be good, so that the EL performance of the devices fabricated from C-IM-CN were better than that of C-IM-Bu.
4. Conclusions
Two coumarin-triarylimidazole hybrid derivatives (C-IM-Bu and C-IM-CN) were designed and prepared for OLEDs, in which a N,N-diethylamine at the 7-position of the coumarin skeleton was the electron-donor and a triaryl-substituted imidazole at the 3-position of the coumarin skeleton was the electron-acceptor. These compounds exhibited strong blue-green emission in a CH2Cl2 solution and green emission in thin solid films as well as good thermal stability (Td > 350 °C). Among these compounds, the compound C-IM-CN showed better PL and EL properties. The EL devices fabricated from C-IM-CN at a 3 wt% doping concentration exhibited the best EL performance with the maximum luminance of 6896 cd m−2, the maximum current efficiency of 6.39 cd A−1 and the maximum external quantum efficiency of 2.92%.
Conflicts of interest
There are no conflicts to declare.
Acknowledgements
This work was supported by the National Natural Science Foundation of China (Grant 51563014, 61864005) and the Innovation Star Project for Excellent Postgraduates in Gansu Province (2021CXZX-550).
References
- D. X. Cao, Z. Q. Liu, P. Verwilst, S. Koo, P. Jangjili, J. S. Kim and W. Y. Lin, Coumarin-based small-molecule fluorescent chemosensors, Chem. Rev., 2019, 119, 10403–10519 CrossRef CAS.
- J. H. Chen, W. M. Liu, B. J. Zhou, G. L. Niu, H. Y. Zhang, J. S. Wu, Y. Wang, W. G. Ju and P. F. Wang, Coumarin- and rhodamine-fused deep red fluorescent dyes: Synthesis, photophysical properties, and bioimaging in vitro, J. Org. Chem., 2013, 78, 6121–6130 CrossRef CAS PubMed.
- N. Prachumrak, S. Potjanasopa, R. Rattanawan, S. Namuangruk, S. Jungsuttiwong, T. Keawin, T. Sudyoadsuk and V. Promarak, Coumarin-cored carbazole dendrimers as solution-processed non-doped green emitters for electroluminescent devices, Tetrahedron, 2014, 70, 6249–6257 CrossRef CAS.
- S. S. E. Puthumana and B. Damodaran, Multicomponent-reaction- (MCR-) assisted synthesis of a coumarin-based deep blue emitter for OLEDs and related applications, ChemistrySelect, 2018, 3, 2951–2957 CrossRef CAS.
- C. T. Chen, C. L. Chiang, Y. C. Lin, L. H. Chan, C. H. Huang, Z. W. Tsai and C. T. Chen, Ortho-substituent effect on fluorescence and electroluminescence of arylamino-substituted coumarin and stilbene, Org. Lett., 2003, 5, 1261–1264 CrossRef CAS.
- S. Kumar, B. Puttaraju and S. Patil, A deep-blue electroluminescent device based on a coumarin derivative, ChemPlusChem, 2016, 81, 384–390 CrossRef CAS.
- P. Kotchapradist, N. Prachumrak, T. Sunonnam, R. Tarsang, S. Namuangruk, T. Sudyoadsuk, T. Keawin, S. Jungsuttiwong and V. Promarak, N-Coumarin derivatives as hole-transporting emitters for high efficiency solution-processed pure green electroluminescent devices, Dyes Pigm., 2015, 112, 227–235 CrossRef CAS.
- H. Zhang, X. C. Liu, Y. X. Gong, T. Z. Yu and Y. L. Zhao, Synthesis and characterization of SFX-based coumarin derivatives for OLEDs, Dyes Pigm., 2021, 185, 108969 CrossRef CAS.
- Y. J. Wang, Y. M. Li, T. Z. Yu, W. M. Su, H. L. Ma, Y. L. Zhao, X. Y. Li and H. Zhang, Functionalized coumarin derivatives containing aromatic-imidazole unit as organic luminescent materials, Dyes Pigm., 2020, 173, 107958 CrossRef CAS.
- T. Z. Yu, Z. Y. Zhu, Y. J. Bao, Y. L. Zhao, X. X. Liu and H. Zhang, Investigation of novel carbazole-functionalized coumarin derivatives as organic luminescent materials, Dyes Pigm., 2017, 147, 260–269 CrossRef CAS.
- Z. S. Wang, Y. Cui, K. Hara, Y. Dan-oh, C. Kasada and A. Shinpo, A high-light-harvesting-efficiency coumarin dye for stable dye-sensitized solar cells, Adv. Mater., 2007, 19, 1138–1141 CrossRef CAS.
- C. J. Zhong, J. R. Gao, Y. H. Cui, T. Li and L. Han, Coumarin-bearing triarylamine sensitizers with high molar extinction coefficient for dye-sensitized solar cells, J. Power Sources, 2015, 273, 831–838 CrossRef CAS.
- X. G. Liu, J. M. Cole, P. G. Waddell, T. C. Lin, J. Radia and A. Zeidler, Molecular origins of optoelectronic properties in coumarin dyes: toward designer solar cell and laser applications, J. Phys. Chem. A, 2012, 116, 727–737 CrossRef CAS.
- H. Feng, R. Li, Y. Song, X. Li and B. Liu, Novel D–π–A–π–A coumarin dyes for highly efficient dye-sensitized solar cells: Effect of π-bridge on optical, electrochemical, and photovoltaic performance, J. Power Sources, 2017, 345, 59–66 CrossRef CAS.
- B. Liu, R. Wang, W. Mi, X. Li and H. Yu, Novel branched coumarin dyes for dye-sensitized solar cells: significant improvement in photovoltaic performance by simple
structure modification, J. Mater. Chem., 2012, 22, 15379–15387 RSC.
- S. S. Keskin, N. Aslan and F. Bayrakçeken, Optical properties and chemical behavior of laser-dye Coumarin-500 and the influence of atmospheric corona discharges, Spectrochim. Acta, Part A, 2009, 72, 254–259 CrossRef.
- A. Dorlars, C.-W. Schellhammer and J. Schroeder, Heterocycles as structural units in new optical brighteners, Angew. Chem., Int. Ed. Engl., 1975, 14, 665–679 CrossRef.
- M. Tasior, D. Kim, S. Singha, M. Krzeszewski, K. H. Ahn and D. T. Gryko, π-Expanded coumarins: synthesis, optical properties and applications, J. Mater. Chem. C, 2015, 3, 1421–1446 RSC.
- C. Y. Li, D. Wang, W. H. Xue, J. F. Peng, T. Wang and Z. T. Zhang, Synthesis and photophysical properties of vertically π-expanded coumarins, Dyes Pigm., 2021, 186, 108956 CrossRef CAS.
- Z. R. Grabowski, K. Rotkiewicz and W. Rettig, Structural changes accompanying intramolecular electron transfer: focus on twisted intramolecular charge-transfer states and structures, Chem. Rev., 2003, 103, 3899–4031 CrossRef.
- A. B. Tathe, V. D. Gupta and N. Sekar, Synthesis and combined experimental and computational investigations on spectroscopic and photophysical properties of red emitting 3-styryl coumarins, Dyes Pigm., 2015, 119, 49–55 CrossRef CAS.
- V. D. Gupta, A. B. Tathe, V. S. Padalkar, P. G. Umape and N. Sekar, Red emitting solid state fluorescent triphenylamine dyes: Synthesis, photo-physical property and DFT study, Dyes Pigm., 2013, 97, 429–439 CrossRef CAS.
- T. Z. Yu, Y. L. Zhao and D. W. Fan, Synthesis, crystal structure and photoluminescence of 3-(1-benzotriazole)-4-methyl-coumarin, J. Mol. Struct., 2006, 791, 18–22 CrossRef CAS.
-
H. Q. Li, L. Cai and Z. Chen, Coumarin-derived fluorescent chemosensors, in Advanced Chemical Sensors, ed. W. Wang, 2012, pp. 121–150 Search PubMed.
-
K. H. Drexhage, Structure and properties of laser dyes, in Dye Lasers, ed. F. P. Schäfer, Springer-Verlag, Berlin, NY, 1990, pp. 144–193 Search PubMed.
- T. Z. Yu, P. Zhang, Y. L. Zhao, H. Zhang, J. Meng and D. W. Fan, Synthesis, characterization and high-efficiency blue electroluminescence based on coumarin derivatives of 7-diethylamino-coumarin-3-carboxamide, Org. Electron., 2009, 10, 653–660 CrossRef CAS.
- M. Mitsuya, T. Suzuki, T. Koyama, H. Shirai and Y. Taniguchi, Bright red organic lightemitting diodes doped with a fluorescent dye, Appl. Phys. Lett., 2000, 77, 3272–3274 CrossRef CAS.
- N. Prachumrak, S. Pojanasopa, R. Tarsang, S. Namuangruk, S. Jungsuttiwong, T. Keawin, T. Sudyoadsuk and V. Promarak, Synthesis and characterization of carbazole dendronized coumarin derivatives as solution-processed non-doped emitters and hole-transporters for electroluminescent devices, New J. Chem., 2014, 38, 3282–3294 RSC.
- W. C. Chen, Z. L. Zhu and C. S. Lee, Organic Light-emitting diodes based on imidazole semiconductors, Adv. Opt. Mater., 2018, 1800258 CrossRef.
- W. C. Chen, Q. X. Tong and C. S. Lee, The development of phenanthroimidazole derivatives in blue-emitting organic electroluminescence, Sci. Adv. Mater., 2015, 7, 2193–2205 CrossRef CAS.
- J. Tagare and S. Vaidyanathan, Recent development of phenanthroimidazolebased fluorophores for blue organic light-emitting diodes (OLEDs): an overview, J. Mater. Chem. C, 2018, 6, 10138–10173 RSC.
-
C. Hofmann, Imidazole and Its Derivatives, Interscience Publishers, Inc., New York, 1953 Search PubMed.
- A. Richaud, N. Barba-Behrens and F. Méndez, Chemical reactivity of the imidazole: a semblance of pyridine and pyrrole?, Org. Lett., 2011, 13, 972–975 CrossRef CAS PubMed.
- S. B. Yadav, S. Kothavale and N. Sekar, Triphenylamine and N-phenyl carbazole-based coumarin derivatives: Synthesis, solvatochromism,
acidochromism, linear and nonlinear optical properties, J. Photochem. Photobiol., A, 2019, 382, 111937 CrossRef CAS.
- B. J. Zhao, G. H. Xie, H. Q. Wang, C. M. Han and H. Xu, Simply structured near-infrared emitters with a multicyano linear acceptor for solution-processed organic light-emitting diodes, Chem. – Eur. J., 2019, 25, 1010–1017 CrossRef CAS.
- B. J. Zhao, H. Q. Wang, M. J. Xie, C. M. Han, H. R. Yang, W. L. Zhao, Q. Zhao and H. Xu, Phosphine oxides manipulate aggregation-induced delayed fluorescence for time-resolved bioimaging, Adv. Photonics Res., 2021, 2, 2000096 CrossRef.
- Z. Li, D. Z. Yang, C. M. Han, B. J. Zhao, H. Q. Wang, Y. Man, P. Ma, P. Chang, D. G. Ma and H. Xu, Optimizing charge transfer and out-coupling of a quasi-planar deep-red TADF emitter: towards Rec.2020 gamut and external quantum efficiency beyond 30%, Angew. Chem., Int. Ed., 2021, 60, 14846–14851 CrossRef CAS PubMed.
|
This journal is © The Royal Society of Chemistry and the Centre National de la Recherche Scientifique 2022 |