DOI:
10.1039/D1NJ02770K
(Paper)
New J. Chem., 2022,
46, 199-211
One-pot synthesis of a highly disperse core–shell CuO–alginate nanocomposite and the investigation of its antibacterial and catalytic properties
Received
6th June 2021
, Accepted 1st November 2021
First published on 19th November 2021
Abstract
In this study, sodium alginate was extracted from Sargassum algae, collected from coastal waters of Bushehr, Persian Gulf, Iran and used as a stabilizing and wrapping agent for CuO nanoparticles. The synthesized nanocomposite was characterized by some spectroscopic and microscopic techniques, such as IR, XRD, Uv–vis, BET, BJH, zeta potential, SEM, TEM, HR-TEM, and XPS. The antibacterial effects of the CuO–alginate nanocomposite against some bacteria, isolated from a burn wound, were evaluated. The results showed that this nanocomposite had better antibacterial effects than its components on Pseudomonas aeruginosa ATCC 27853, Staphylococcus aureus ATCC 12600, Streptococcus pyogenes ATCC 19615, and Staphylococcus epidermidis ATCC 49461. Among these, Staphylococcus aureus ATCC 12600 was the most sensitive one to this nanocomposite, with the lowest minimum inhibitory concentration (2.08 mg mL−1) observed. Moreover, the synthesized nanocomposite showed good catalytic activity in the oxidative coupling of carboxylic acids with N,N-dialkylformamides toward the synthesis of amides.
1. Introduction
Copper is one of the metals that has many applications in various fields of science. One of its salts is CuO, also known as cupric oxide or tenorite, a black solid that has received much attention in recent years because of its wide range of applications, such as catalytic,1 optical,2 sensors,3 electrical,4 and antibacterial and antifungal properties,5 and for the preparation of organic–inorganic nanocomposites.6 Due to their large surface area and high reactivity, nanosized structures show excellent properties compared to bulk samples.7 Consequently, the synthesis of nanoscale structures is preferred and considered, however, one problem with nanoscale structures is that they tend to accumulate, which in turn can affect their physicochemical properties. Although, there are various ways to stabilize nanoparticles in the environment,8 a popular and efficient strategy is to wrap the nanoparticles with a polymer, especially biopolymers that are non-toxic, biodegradable, and environmentally friendly. One of these biopolymers is sodium alginate, which is structurally a polysaccharide consisting of two monosaccharides called mannuronic acid and guluronic acid. This eco-friendly biopolymer has a strong tendency to adhere to metals and has many applications in drug delivery, bone tissue engineering, and dental impression materials.9 Therefore, sodium alginate can be a good candidate for stabilizing and dispersing nanoparticles in the environment and would have positive effects on their properties and applications in various fields, especially catalytic, antibacterial, and microbial. Diaz-Visurraga et al. (2012) reported the antibacterial effect of alginate-stabilized copper nanoparticles against Staphylococcus aureus ATCC 6538.10 In 2018, Safaei et al. prepared an alginate–CuO bionanocomposite and examined its antibacterial activity against S. aureus and E. coli.11 In 2018, Siddiqui et al. reported the synthesis of CuO–Alg nanocomposites using the sol–gel method and investigated its dielectric behavior as a function of increasing frequency by an LCR meter.12 Safaei et al. also reported in 2019 the preparation of an alginate–CuO bionanocomposite and examined its thermal stability and found that the thermal stability of the alginate biopolymer and CuO nanoparticles were improved by the formation of the nanocomposite. They also showed that the synthesized nanocomposite had desirable antifungal activity against Aspergillus niger.13 Recently, Kudzin et al. described the synthesis of antimicrobial polylactide-copper alginate composite fibers and evaluated their antimicrobial activity against colonies of Gram-negative (E. coli) and Gram-positive (S. aureus) bacteria and in antifungal susceptibility tests against Aspergillus niger and Chaetomium globosum fungal mold species.14 In this line of research, here, we report the synthesis of a highly disperse core–shell CuO–alginate nanocomposite with good antibacterial activity against some bacteria isolated from burn wounds. The nanocomposite was also found to play a good role as a catalyst in the formation of amide bonds between carboxylic acids and formamides.
2. Materials and methods
2.1. General information
The chemicals used in this study were purchased commercially from Merck or Aldrich and used directly without further purification. IR spectra were recorded on a PerkinElmer FT/IR 1760 as KBr pellets. Powder X-ray diffraction spectra were recorded at room temperature on a Philips X’pert 1710 diffractometer using Cu-Kα (α = 1.54056 Å) in the Bragg–Brentano geometry (θ–2θ). The BET specific surface area, pore volume, and average pore size were determined by the physical adsorption of N2 at −196 °C in an automatic volumetric system using a Belsorp instrument. Merck silica gel 60 (63–200 mesh) was used for the column chromatography. Analytical TLC was performed with Merck silica gel 60 F254 plates, and the products were identified by 1H-NMR and 13C-NMR (300.1 MHz and 75.4 MHz, respectively). All the NMR spectra were determined in CDCl3 at ambient temperature. UV–vis absorption spectra were obtained at 200 to 800 nm by an Agilent UV–vis detector (model 8453). The zeta potential of the samples was measured by a zeta potential meter (ZetaCAD) in distilled water (conductivity = 20 lS cm−1, pH 5.5–6) and in the original dispersion medium (distillate water, pH 6.8) at 25 °C. High-resolution transmission electron microscopy (HR-TEM) experiments were performed on an FEI Model Tecnai G2, F30, with the resolution point: 2.0 Å; line: 1.0 a; magnification: 58× to 1
000
000 ×; potential: 300 kV. The X-ray photoelectron spectroscopy (XPS) analysis was done on an ESCALAB 250Xi instrument with Mg X-ray resource. In this experimental study, Pseudomonas aeruginosa ATCC 27853, Klebsiella pneumoniae ATCC 13883, Staphylococcus epidermidis ATCC 49461, Streptococcus pyogenes ATCC 19615, and Staphylococcus aureus ATCC 12600 were obtained from the Medical School of Shiraz University. The test isolate was grown with Antibacterial Susceptibility Assay Muller-Hinton Broth (MHB, Merck) at 37 °C for 24 h. The prepared suspension was inoculated on Mueller Hinton agar medium (Merck, Darmstadt, Germany). The surface culture was performed by sterile L shape pipette Pasteur on the appropriate media and incubated at 37 °C for 24 h.
2.2. Collection of seaweed and sodium alginate extraction
Sargassum latifolium brown macroalgae was collected from the coastal waters of Bushehr, Persian Gulf, Iran at low tide or by snorkeling and was washed repeatedly with double distilled water to remove debris and mud. Algae powder was obtained by shade-drying at room temperature, followed by grinding to yield dried algal powder. Sodium alginate was extracted from the as-obtained algal powder based on the previously reported method with slight adjustments.15 The extract was prepared by adding algae powder (5 g) to 200 mL distilled water and 20 mL of 0.1 N HCl aqueous solution with mixing with a magnetic stirrer at pH 4. The mixture was then added to 125 mL Na2CO3 solution (0.1 N, pH 12) and then stirred for 2 h at 60 °C. The mixture was diluted to 400 mL using water and then stirred for 15 min. Then, by centrifugation of the mixture, insoluble materials precipitated to obtain a solution containing sodium alginate. Ethanol was added to the solution (1
:
1 v/v) under stirring to precipitate sodium alginate. The purification of the extracted sodium alginate was performed by ethanol washing by Soxhlet for 100 h. Finally, the washed biopolymer was vacuum dried at room temperature.
2.3. Synthesis of the CuO–alginate nanocomposite
In order to synthesize the CuO–alginate nanocomposite, into a 100 mL round-bottom flask was added a solution of sodium alginate (1.5% w/w, 20 mL), CuSO4·5H2O (0.05 M, 20 mL), and NaOH (1 M, 10 mL), respectively. The pH of the solution in this case was 12.7. The resulting mixture was stirred for 2 h on a magnetic stirrer at 60 °C. The discoloration of the solution from blue to brown showed the formation of copper oxide nanoparticles. Finally, the resulting solution was centrifuged and the supernatant, with a pH of 12.3, poured out. The resulting nanocomposite was washed twice with distilled water and ethanol, respectively, centrifuged each time, and finally placed in an oven at 60 °C for 10 h and dried. For comparison, pure copper oxide nanoparticles (CuO NPs) were also synthesized according to the same procedure, except that sodium alginate solution was not used.
2.4. Cytotoxicity assay
2.4.1. Disk diffusion antibiotic sensitivity testing.
The antibacterial properties of the CuO–alginate nanocomposite and CuO nanoparticles were assessed on Pseudomonas aeruginosa ATCC 27853, Klebsiella pneumoniae ATCC 13883, Staphylococcus epidermidis ATCC 49461, Streptococcus pyogenes ATCC 19615, and Staphylococcus aureus ATCC 12600 by the disc diffusion method. The bacterial suspension was grown on an agar medium, and then the prepared disks were placed on a bacterial culture medium. To determine the sensitivity of each bacterial species tested, lincomycin was used as a positive control standard. The plates were then incubated at 37 °C for 24 h. The diameter of the inhibition zone was measured and reported in millimeters (Four replications for each treatment).
2.4.2. Minimum inhibitory concentration and minimum bactericidal concentration.
To determine the minimum inhibitory concentration (MIC) and minimum bactericidal concentration (MBC), a set of 9 sterile test tubes was used for each treatment. The stock solutions (500 mg ml−1) were further diluted in a 2-fold serial dilution to obtain the following concentrations: 250, 125, 62.5, 31.25, 15.625, 7.8125, 3.91, 1.95, and 0.98 mg mL−1. One test tube as a negative control and lincomycin as a positive control were used. An aliquot of 1 mL of the bacterial suspension was inoculated in each tube. All the tubes were incubated at 37 °C for 24 h. The lowest concentration that did not permit any visible growth when compared with the control was considered as the MIC. To determine the MBC, the solution of the transparent tubes of the previous step was cultured in TSA medium. A plate with no bacterial growth was considered as the MBC (Four replications for each treatment).
2.5. General procedure for the synthesis of amides 3a–3o
A mixture of carboxylic acid 1 (1.0 mmol) and CuO–alginate nanocomposite (20 mg) in N,N-dialkylformamide 2 (2.0 mL) was placed in a two-necked reactor with a condenser at room temperature. Then, TBHP (70 wt% in H2O, 1.5 equiv.) was added to the reaction mixture slowly over a 5 min period, and the mixture was placed in an oil bath with magnetic stirring at 70 °C in an open atmosphere. Completion of the reaction was monitored by TLC. Finally, after cooling to room temperature, the reaction mixture was filtered off and the catalyst removed. Water (2 mL) was added and the product extracted with ethyl acetate (3 × 2 mL), and washed with saturated NaHCO3 solution, water, and brine solution, respectively. The organic layer was collected and dried with Na2SO4. The solvent was removed under reduced pressure. The residue was purified by column chromatography on silica gel (petroleum ether/ethyl acetate) to afford the pure products.
3. Results and discussion
In this study, sodium alginate, extracted from the native algae of the Persian Gulf, Sargassum, was used as a stabilizing and complexing agent to synthesize a CuO–alginate nanocomposite. Visual observation as a change in color in the synthesized CuO–alginate nanocomposite is shown in Fig. 1. As seen in this figure, the sodium alginate solution (1.5% w/w) (sample A) was pale yellow, and the CuSO4·5H2O (0.05 M) solution (sample B) was sky blue. When the two solutions were mixed, the resulting mixture (sample C) turned a sea green. When adding NaOH solution to the resulting mixture, the color changed to blue (sample D), then, after stirring at 60 °C for 2 h, its color changed to black, which was a sign of the synthesis of copper oxide nanoparticles (sample E). After the purification process, the solid black CuO–alginate nanocomposite (sample F) was obtained.
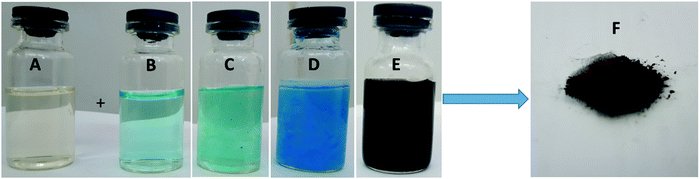 |
| Fig. 1 Visual observation as a change in color in the synthesis of CuO–alginate nanocomposite. | |
The prepared CuO–alginate nanocomposite was then characterized using some microscopic and spectroscopic techniques, such as FT-IR, XRD, UV–vis, SEM, HR-TEM, zeta potential, BET, BJH, and XPS. The FT-IR spectra of pure CuO nanoparticles, sodium alginate, and CuO–alginate nanocomposite are been shown in Fig. 2. In the FT-IR spectra of pure CuO nanoparticles, the peaks positioned at 410, 492, and 614 cm−1 were the characteristic stretching vibrations of Cu–O bonds in the monoclinic CuO phase.16 Absorption at 1618 and 3414 cm−1 was ascribed to H–O–H bending and O–H stretching, respectively.17
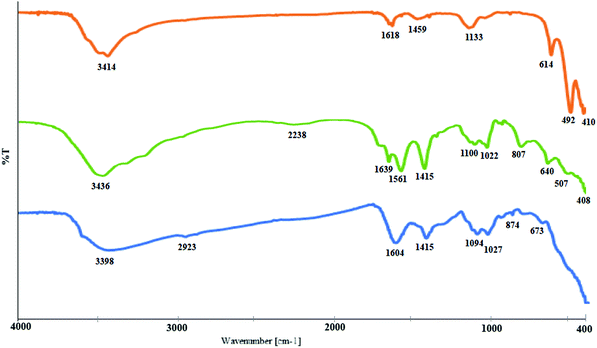 |
| Fig. 2 IR spectra of the pure CuO nanoparticles, sodium alginate, and CuO–alginate nanocomposite (top to bottom, respectively). | |
In the IR spectrum of sodium alginate, the broad band centered at 3436 cm−1 could be assigned to the hydrogen bonded O–H stretching vibrations, while the weak signal at 2942 cm−1 corresponded to the C–H stretching vibrations. The appearance of a strong peak at 1639 cm−1 was ascribed to the asymmetric stretching of carboxylate O–C–O vibration. Symmetric stretching vibration of the carboxylate group appeared as an intense band at 1415 cm−1. The absorption peaks in the range 1000–1100 were assigned to C–C–H and O–C–H deformation, C–O stretching, C–O and C–C stretching vibrations of pyranose rings, respectively. The bands appearing in the 700–950 range were also related to the carbohydrate skeleton.18 These common peaks of sodium alginate were also seen in the CuO–alginate nanocomposite, except with a red-shift for the absorption peaks of pure sodium alginate at 1639, and 1100 cm−1, which could confirm the interaction between the polymer carboxylate groups and the copper oxide.19
The XRD patterns of the pure CuO NPs and CuO–alginate nanocomposite are shown in Fig. 3. The patterns could be readily referred to the crystalline structures of tenorite (CuO), which are monoclinic (JCPDS no. 45-0937). In the pattern assigned to CuO NPs, the diffraction peaks at around 38.03, 41.50, 45.30, 54.20, 57.30, 63.06, 68.53, 72.87, 78.56, and 80.84 corresponded to the (110), (−111), (111), (−202), (020), (202), (−113), (−311), (220), and (311) reflections, respectively. No other characteristic peaks due to the impurities of other oxides of copper were detected. The slight changes observed in the XRD patterns of CuO NPs and CuO–alginate nanocomposite showed that the crystalline structure of the nanoparticles was also preserved after interaction with sodium alginate. The large peak widths were ascribed to the formation of nanosized particles of CuO. The sizes of the particles were calculated by the Debye–Scherrer equation20 using the (111) peak (2θ = 45.30), which were about 40 nm. Based on the XRD pattern, the crystal system was monoclinic and the number of both copper and oxygen atoms in the unit cell was 4 (Cu4.00O4.00), with the lattice parameters a = 4.67 Å, b = 3.43 Å, and c = 5.12 Å and the volume of the unit cell was 82.01 Å3 which were in agreement with the standard card (96-101-1195).
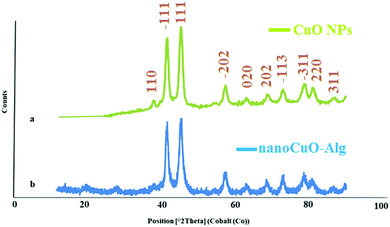 |
| Fig. 3 XRD patterns of pure CuO nanoparticles (a) and CuO–alginate nanocomposite (b). | |
The formation of the CuO–alginate nanocomposite was confirmed by the UV–visible spectral analysis recorded in the range of 190 to 790 nm (Fig. 4). A narrow absorption peak at about 203 nm was observed for the extracted alginate biopolymer and three broad peaks at about 216, 294, and 280 nm were obtained from the pure CuO nanoparticles.21 The absorption peaks observed in the CuO–alginate nanocomposite spectrum indicated the presence of CuO nanoparticles and the alginate biopolymer in this structure and confirmed the formation of this nanocomposite.
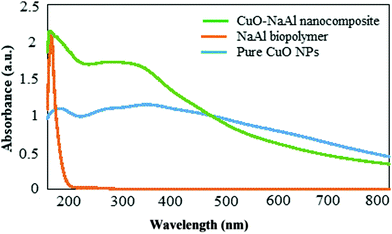 |
| Fig. 4 UV–vis spectral analysis of the alginate biopolymer, CuO NPs, and CuO–alginate nanocomposite. | |
The bandgap energy of the CuO NPs was obtained by Tauc plot equation,22 as follows:
Based on the above equation, the band gap for CuO nanoparticles was 1.8 eV. (Fig. 5).
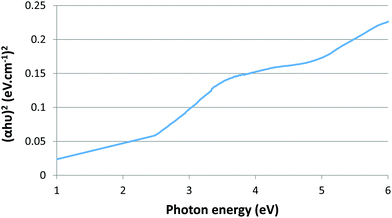 |
| Fig. 5 Plot of (αhυ)2versus photon energy for the determination of the band gap of CuO. | |
The morphology of the synthesized nanocomposite was investigated by SEM and TEM. Fig. 6(a and b) show the SEM images of CuO–alginate nanocomposite, which tended to be spherical, and Fig. 4c and d depict the morphologies of the pure copper nanoparticles, which have a completely different appearance from the nanocomposite and appeared to be laminated.
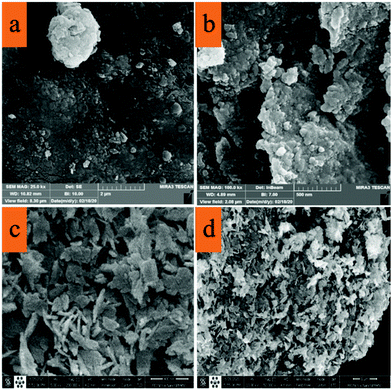 |
| Fig. 6 SEM images of CuO–alginate nanocomposite (a and b) and pure CuO nanoparticles (c and d). | |
The TEM images show that sodium alginate surrounded the nanoparticles and caused them to be highly dispersed in the environment due to the negative charge that surrounded the surface of the nanoparticles (Fig. 7a and b). To ensure that the dark regions in the TEM were tenorite, SAED patterns were recorded. From the SAED patterns, the d-spacing values were calculated as described in Fig. 7d. The d-spacing values calculated from the SAED patterns corresponded to the standard atomic spacing for tenorite from the standard card (96-101-1195).
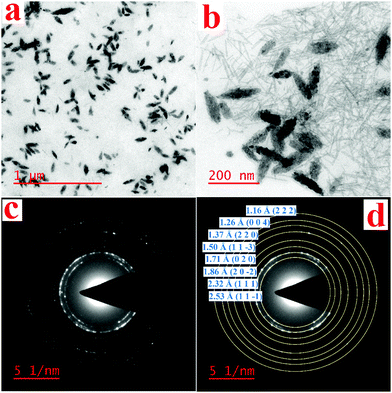 |
| Fig. 7 TEM images of CuO–alginate nanocomposite (a and b) and SAED pattern (c and d). | |
Fig. 8 shows the high dispersion of the nanocomposite in the aqueous medium. As can be seen in the image, the copper nanoparticles precipitated completely after 30 min, while a significant portion of the nanocomposite was still dispersed in the environment after 3 months.
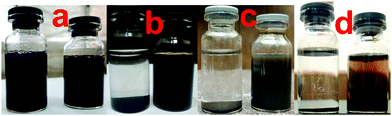 |
| Fig. 8 Dispersity of the bare CuO NPs (left) and CuO–alginate nanocomposite (right) immediately after turbulence (a); after 30 min (b); after 1 month (c); and after 3 months (d). | |
The surface charge of the nanocomposite was assessed by zeta potential analysis, which was −33.30 mV, and this could be the main cause for the high dispersion of the nanoparticles in the environment. Fig. 9 shows the zeta potential distribution graph for the CuO–alginate nanocomposite.
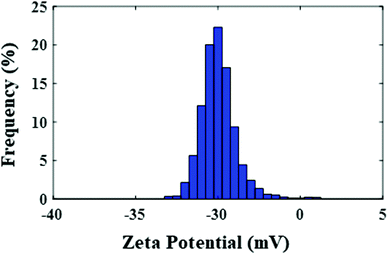 |
| Fig. 9 Zeta potential distribution graph for CuO–alginate nanocomposite. | |
As it is clear from Fig. 10 that both materials showed nearly type IV isotherms with H3 hysteresis loops. The jumps can be seen between in the range of 0.7–1.0 relative pressure. No capillary condensation appeared. From the H3 hysteresis loop, it could be found that the synthesized materials were the mesoporous compounds having slit-like pores or were non-rigid aggregates of plate-like particles with a wide range of pore sizes and volumes.23 This was confirmed by using the Barrett–Joyner–Halenda (BJH) equation, which showed a wide range of pores in both compounds with sizes from about 10 to 100 nm and average pore sizes of 28.2 nm and 33.4 nm for pure CuO and CuO–alginate, respectively. The specific surface areas of the materials were investigated by BET, which showed that the chelation with alginate reduced the specific surface area from 24.6 to 12.4 m2 g−1. The differences in the surface areas and pore sizes may be related to the difference in the surface morphologies.
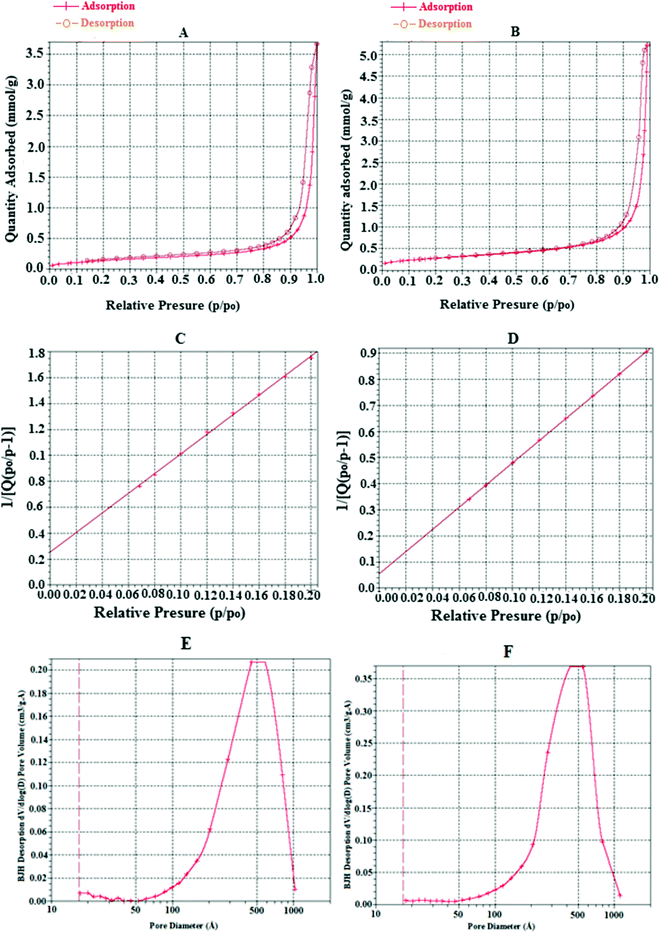 |
| Fig. 10 N2 adsorption–desorption isotherm of the synthesized CuO–alginate nanocomposite (A) and pure CuO nanoparticles (B); BET surface area plot of CuO–alginate nanocomposite (C) and BET surface area plot of pure CuO nanoparticles (D); BJH desorption of the synthesized CuO–alginate nanocomposite (E) and pure CuO nanoparticles (F). | |
The oxidation state of the Cu was examined by X-ray photoelectron spectroscopy analysis. Fig. 11 shows the XPS patterns of the Cu2p and O1s of the CuO–alginate nanocomposite. The Cu2p spectrum of the nanocomposite was characterized by two spin orbit doublets with the presence of strong satellite peaks. The shape of the spectrum and the two peaks at 934.3 and 954.5 eV corresponded to the Cu 2p3/2 and 2p1/2 peaks of Cu2+ in CuO, respectively. The peaks at 944.1 and 963.6 eV were considered to be shakeup satellite peaks. The difference in binding energies between the Cu 2p3/2 and Cu 2p1/2 was found to be 20 eV, which was also in close agreement with the literature-cited values.24 The O1s oxygen spectrum showed a main peak at 531.5 eV, which was assigned to lattice oxygen or oxide O2−.
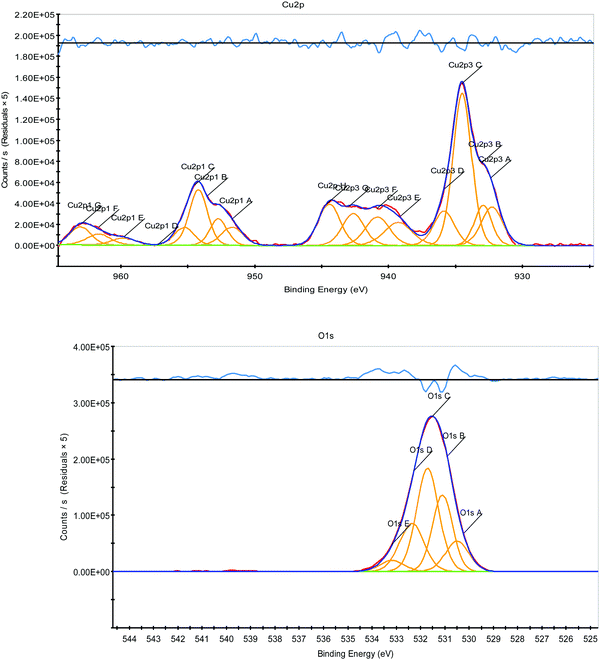 |
| Fig. 11 X-ray photoelectron spectra of Cu2p and O1s for CuO nanoparticles surrounded by sodium alginate. | |
After identifying the structure of the nanocomposite, its antibacterial and catalytic activity were investigated. There are several reports about the antibacterial properties of CuO NPs.5 However, the antibacterial activity of CuO–alginate nanocomposite has been less studied. Safaei et al. (2018) investigated the effect of the alginate–CuO nanocomposite against Gram-positive (S. aureus) and Gram-negative (E. coli) bacteria.11 In this study, the antibacterial activities of the CuO–alginate nanocomposite and pure CuO nanoparticles (for comparison) were evaluated against Pseudomonas aeruginosa ATCC 27853, Klebsiella pneumoniae ATCC 13883, Streptococcus pyogenes ATCC 19615, Staphylococcus epidermidis ATCC 49461, and Staphylococcus aureus ATCC 12600 bacteria, isolated from a burn wound, by a disc diffusion assay. Generally speaking, Staphylococcus aureus ATCC 12600 was highly affected by the CuO nanoparticles (CuO–NPs) and CuO–alginate nanocomposite (Table 1 and Fig. 12). The MIC and MBC values of the CuO–NPs and CuO–alginate nanocomposite, at different concentrations ranging from 0.98 mg mL−1 to 250 mg mL−1, were compared with the activity of lincomycin, and the results are presented in Tables 2 and 3. Between the CuO–NPs and CuO–alginate nanocomposite, the latter showed the highest antibacterial activity against these bacteria. The lowest MIC (2.08 mg mL−1) was for Staphylococcus aureus ATCC 12600, while the highest MIC (16.66 mg mL−1) was for Pseudomonas aeruginosa ATCC 27853. In terms of the antibacterial effect mechanisms, physical disruption and oxidative stress were the main causes of the NPs’ toxicity. Reactive oxygen species (ROS), including superoxide anion, hydrogen peroxide, hydroxyl radicals, and organic hydroperoxides, NPs deposition on the surface of bacteria, and NPs accumulation in the cytoplasm/periplasmic region can result in bacterial death. ROS are an important factor for several antibacterial mechanisms, including damage to the cell wall by interaction with CuO, increased membrane permeability, and the internalization of NPs due to the loss of proton motility. These lead to mitochondrial weakness, intracellular outflow, and the release of oxidative stress genes that inhibit cell growth and cell death.25 ROS can result in damage to cellular constituents, like lipids, peptidoglycan, proteins, and DNA through their release from NPs and subsequent penetration into bacteria.26 Copper (Cu) dissolves on the surface of the bacteria and penetrates the bacteria cells, thereby breaking the cell membrane.27 Due to the stresses caused by Cu atoms and other phenomena leading to the loss of membrane potential and cytoplasmic contents, Cu ions stimulate the development of reactive oxygen species, causing further damage to the cells, and finally, the degradation of genomic and plasmid DNA.28
Table 1 Antibacterial properties of the CuO–alginate nanocomposite against bacteria isolated from burn wounds
Treatment |
Inhibition zone (cm) |
PA. ATCC 27853 |
SE. ATCC49461 |
SA. ATCC 12600 |
KP. ATCC 13883 |
SP. ATCC 19615 |
SA, sodium alginate; CuO–NPs, copper oxide nanoparticles; CuO–SA–NPs, CuO–alginate nanocomposite; Lin, lincomycin; DW, deionized water; PA. ATCC 27853, pseudomonas aeruginosa ATCC 27853; SE. ATCC49461, staphylococcus epidermidis ATCC 49461; SA. ATCC 12600, staphylococcus aureus ATCC 12600; KP. ATCC 13883, klebsiella pneumoniae ATCC 13883; SP. ATCC 19615, streptococcus pyogenes ATCC 19615. a–d means ±sd within columns with no common superscript differ significantly (p ≤ 0.05). |
SA |
0.00 ± 0.00c |
0.53 ± 0.46c |
0.90 ± 0.17c |
0.00 ± 0.00b |
0.26 ± 0.46 |
CuO–NPs |
0.00 ± 0.00c |
0.96 ± 0.28bc |
1.16 ± 0.32c |
0.00 ± 0.00b |
0.93 ± 0.23 |
CuO–SA–NPs |
0.90 ± 0.1b |
1.23 ± 0.05b |
1.76 ± 0.05b |
0.00 ± 0.00b |
1.06 ± 0.05 |
Lin |
2.70 ± 0.26a |
2.93 ± 0.35a |
3.16 ± 0.15a |
2.96 ± 0.15a |
3.13 ± 0.15 |
DW |
0.00 ± 0.00c |
0.00 ± 0.00d |
0.00 ± 0.00d |
0.00 ± 0.00b |
0.00 ± 0.00 |
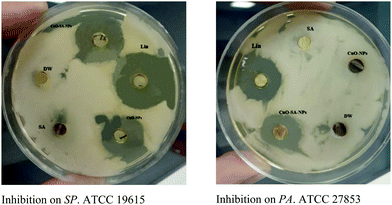 |
| Fig. 12
In vitro antibacterial activity of CuO–alginate nanocomposite. SP. ATCC 19615: Streptococcus pyogenes ATCC 19615, PA. ATCC 27853: Pseudomonas aeruginosa ATCC 27853. | |
Table 2 MIC (mg ml−1) results of CuO–alginate nanocomposite against bacteria isolated from burn wounds
Treatment |
PA. ATCC 27853 |
SE. ATCC49461 |
SA. ATCC 12600 |
KP. ATCC 13883 |
SP. ATCC 19615 |
NO, no effect; SA, sodium alginate; CuO–NPs, copper oxide nanoparticles; CuO–SA–NPs, CuO–alginate nanocomposite; Lin, Lincomycin; DW, deionized water; PA. ATCC 27853, pseudomonas aeruginosa ATCC 27853; SE. ATCC49461, staphylococcus epidermidis ATCC 49461; SA. ATCC 12600: staphylococcus aureus ATCC 12600; KP. ATCC 13883, klebsiella pneumoniae ATCC 13883; SP. ATCC 19615, streptococcus pyogenes ATCC 19615. |
SA |
NO |
NO |
41.66 |
NO |
NO |
CUO–NPS |
50.0 |
20.83 |
16.66 |
NO |
41.16 |
CUO–SA–NPS |
16.66 |
8.33 |
2.08 |
NO |
4.16 |
Lin |
1.30 |
0.65 |
1.04 |
1.30 |
1.04 |
DW |
NO |
NO |
NO |
NO |
NO |
Table 3 MBC (mg ml−1) results of CuO–alginate nanocomposite against bacteria isolated from burn wounds
Treatment |
PA. ATCC 27853 |
SE. ATCC49461 |
SA. ATCC 12600 |
KP. ATCC 13883 |
SP. ATCC 19615 |
NO, no effect; SA, sodium alginate; CuO–NPs, copper oxide nanoparticles; CuO–SA–NPs, CuO–alginate nanocomposite; Lin, lincomycin; DW, deionized water; PA. ATCC 27853, pseudomonas aeruginosa ATCC 27853; SE. ATCC49461, staphylococcus epidermidis ATCC 49461; SA. ATCC 12600, staphylococcus aureus ATCC 12600; KP. ATCC 13883, klebsiella pneumoniae ATCC 13883; SP. ATCC 19615, streptococcus pyogenes ATCC 19615. |
SA |
NO |
NO |
NO |
NO |
NO |
CUO–NPS |
NO |
NO |
50.0 |
NO |
NO |
CUO–SA–NPS |
33.3 |
16.66 |
5.20 |
NO |
10.41 |
Lin |
2.60 |
2.60 |
2.08 |
2.08 |
3.12 |
DW |
NO |
NO |
0.00 |
NO |
NO |
In the following, the catalytic role of the synthesized nanocomposite in the formation of one of the most important organic bonds, the amide one, between carboxylic acids and formamides, via the oxidative-coupling pathway, was investigated. In the twentieth century, and especially since the 1970s, coupling reactions have found a special place in the formation of carbon–carbon and carbon–heteroatom bonds.29 In terms of the importance of such reactions, it is enough to note that the 2010 Nobel Prize in Chemistry was awarded to scientists who worked in this field. The irreplaceable role of transition metals as catalysts in the development of these reactions is not hidden from anyone. Among these, copper metal has a special place because it has shown a high ability to perform this type of reaction and is cheap and available compared to other intermediate metals.30 Amides are one of the important functional groups in organic chemistry and therefore the formation of amide bonds is of special importance, because of their presence in several natural products and in many fine chemicals, pharmaceuticals, and agrochemicals.31 A variety of methods have been developed to form amide bonds, employing different raw materials. Oxidative-coupling reactions have also played an important role in the synthesis of amides, and by using a variety of inexpensive and available reactants, interesting routes have been reported to access this family of compounds.32 One of the simplest reported pathways is the oxidative coupling of carboxylic acids and formamides, catalyzed by copper. In 2013, Reddy et al. reported the oxidative-coupling reaction of carboxylic acids with formamides toward the synthesis of amides.33 They succeeded in synthesizing various derivatives of amides from this pathway by employing copper(II) perchlorate hexahydrate, Cu(ClO4)2·6H2O as a catalyst and TBHP as an oxidant at 100 °C for 15 h of reaction. In the same year, Lee and co-authors synthesized amides with the same precursors.34 They optimized the reaction conditions by using copper chloride as a catalyst, DABCO, 1,4-diazabicyclo[2.2.2]octane, as an auxiliary ligand, in a reaction at 80 °C under argon gas overnight. Of course, 1,1,2-trichloroethane was used as a solvent in these reactions. A metal–organic framework (MOF) based on copper and 1,3-bis(carboxymethyl)imidazole (bcmim) was reported in 2016 by Pastor et al. for the preparation of amides through an oxidative coupling between carboxylic acids and formamides in the presence of an oxidant, such as tert-butyl hydroperoxide (TBHP).35 Moreover, Jayaram et al. prepared copper oxide nanoparticles stabilized on the surface of hexagonal mesoporous silica (HMS) and investigated its catalytic activity in the oxidative amidation of carboxylic acids with formamides.36
Here, we were encouraged to investigate the catalytic activity of the synthesized CuO–Alg nanocomposite in the oxidative amidation reaction of carboxylic acids and dimethyl formamide. Fortunately, in the first reaction between benzoic acid and DMF, the corresponding amide was obtained with high efficiency. In this reaction, based on similar reactions reported previously, TBHP (70% aqueous solution) was used as an oxidant, and the reaction was conducted at 70 °C, and after 5 h, the product 3a was extracted with a 75% yield (Scheme 1).
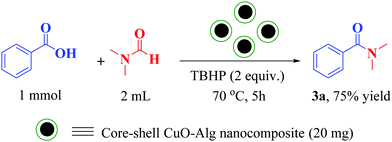 |
| Scheme 1 CuO–alginate nanocomposite-catalyzed amide bond formation. | |
By changing the parameters affecting the reaction, such as the temperature, the amount of catalyst used, and the reaction time, no significant change in the efficiency of the extracted product was observed and therefore the same conditions were used to synthesize more N,N-dialkyl benzamide derivatives (Table 4). As can be seen in Table 4, the catalyst was able to couple well the various derivatives of benzoic acid, including the functional groups of methyl, methoxy, halogens, and nitro, located at different positions of the benzene ring, with DMF and give the corresponding amide with good efficiency (products 3a–j). Moreover, cinnamic acid and phenylacetic acid were well coupled with DMF toward the synthesis of the corresponding amides in acceptable yields (products 3k and 3l). Subsequently, some derivatives of benzoic acids were subjected to the reaction conditions with diethyl formamide and the corresponding amides were formed in good yields (products 3m–o).
Table 4 Structures of the synthesized amidesa
Reaction conditions: carboxylic acid (1 mmol), dialkylformamide (2 mL), catalyst (20 mg), 70 °C, open air, 5 h. Yields refer to the isolated pure products.
Values in parentheses are related to the yields in the presence of the recycled catalyst in three consecutive runs.
|
|
All the synthesized products were known and characterized by IR, and 1H- and 13C-NMR analysis. For example, the 1H-NMR spectrum of the product 3b in CDCl3 exhibited three sharp singlets, at δ = 2.29, 2.91, and 3.02, attributed to the methyl groups. It also exhibited two doublets at 7.12 ppm (3J = 7.86 Hz) and at 7.25 ppm (3J = 8.04 Hz) related to the protons of the aromatic region. In addition, in the carbon spectrum, 8 peaks could be distinguished at δ 21.3, 35.4, 39.7, 127.1, 128.9, 133.3, 139.5, and 171.8 related to the carbons of this compound (Fig. 13).
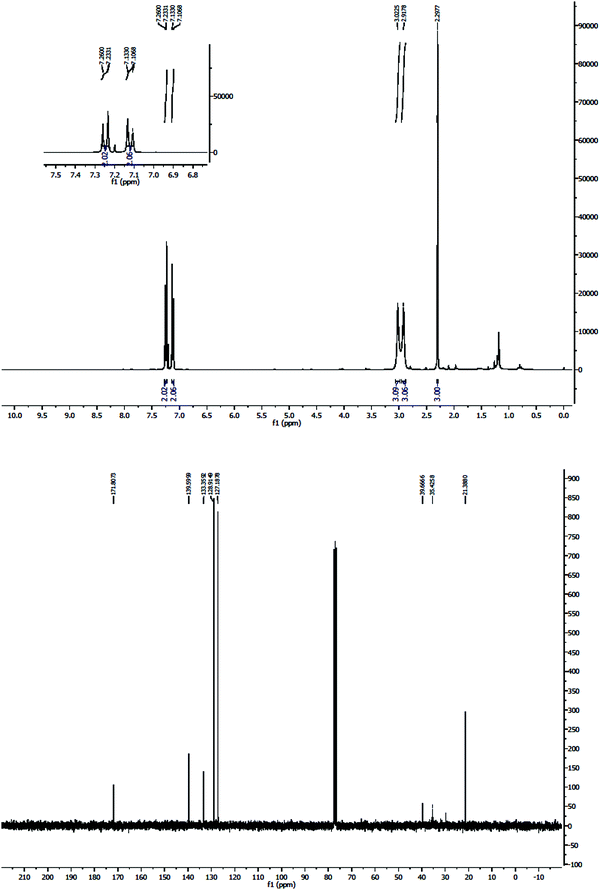 |
| Fig. 13
1H- and 13C-NMR spectra of the product 3b. | |
Then, the reaction efficiency in the presence of the recycled catalyst was investigated in the preparation of compound 3a. For this purpose and after completion of the reaction in the first run, the catalyst was separated by filtration, washed with distilled water (2 × 5 mL), and ethyl acetate (2 × 5 mL), respectively, dried at 60 °C for 1 h, and then used in the next step. The reaction was repeated for three consecutive runs without significant change in the efficiency of the reaction (Table 5). From the data in Table 5, it can be concluded that the decrease in efficiency was due to the decrease in the amount of catalyst in successive runs.
Table 5 Product efficiency and amount of catalyst recycled in the catalyst reuse processa
Run |
Amount of recycled catalyst (mg) |
Yield (%) |
Results are related to the synthesis of the product 3a under optimal conditions.
|
Initial reaction |
20 |
75 |
1 |
19 |
75 |
2 |
17 |
73 |
3 |
15 |
70 |
The mechanism for the oxidative amidation of carboxylic acids and formamides in the presence of the Cu/TBHP system has been investigated in several previously reported papers37 and has been shown to proceed in a radical pathway. In the present study, when 2,2,6,6-tetramethylpiperidin-1-yloxyl (TEMPO), a well-known radical scavenger, was added to the model reaction under the standard conditions, no product 3a was formed, which proved that the reaction might be go through a radical intermediate. As shown in Scheme 2, it can be expected at first that the radical 1 is produced in the presence of Cu(II)/TBHP. Copper(II) is converted to copper(I) in this step. Radical 1 then reacts immediately with DMF to form radical 2. Copper(I) returns to copper(II) by converting the TBHP to radical 3, which then enters the catalytic cycle. Then, radical 4 is achieved via the abstraction of H from the acid by radical 3. Coupling radical 2 with radical 3 followed by the extrusion of CO2 forms the final product (Scheme 2).
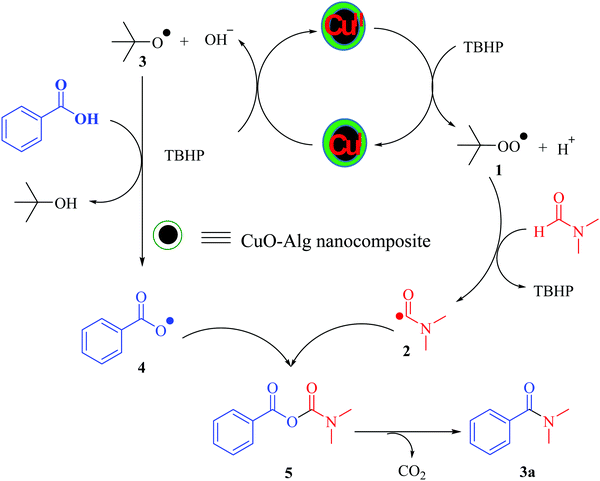 |
| Scheme 2 Plausible mechanism for the formation of the product 3a. | |
4. Conclusion
CuO–alginate nanocomposite was synthesized and its antibacterial and catalytic activity were studied. Complexation of the nanoparticles with sodium alginate showed a high degree of dispersion such that after 3 months, most of the nanoparticles were still scattered in the aqueous environment. The CuO–alginate nanocomposite presented better antibacterial effects than its components on Pseudomonas aeruginosa ATCC 27853, Staphylococcus epidermidis ATCC 49461, Staphylococcus aureus ATCC 12600, and Streptococcus pyogenes ATCC 19615 bacteria. This study demonstrates that the mechanisms behind the antimicrobial response of CuO–alginate nanocomposite in different species of bacterial should be further investigated by assay. Also, the synthesized nanocomposite showed good catalytic activity in the oxidative amidation reaction of carboxylic acids with formamides toward the synthesis of benzamides in good yields.
Conflicts of interest
There are no conflicts to declare.
Acknowledgements
We are thankful to Persian Gulf University Research Council for partial support of this work.
References
-
(a) W. Zhang, F. Guo, F. Wang, N. Zhao, L. Liu, J. Li and Z. Wang, Org. Biomol. Chem., 2014, 12, 5752–5756 RSC;
(b) C. D. S. Dias, T. D. M. Lima, C. G. S. Lima, J. Z. Schpector and R. S. Schwab, ChemistrySelect, 2018, 3, 6195–6202 CrossRef;
(c) N. K. M. Subodh, K. Chaudhary, G. Kumar and D. T. Masram, ACS Omega, 2018, 3, 16377–16385 CrossRef CAS PubMed;
(d) N. Mittapelly, B. R. Regury and K. Mukkanty, Der. Pharm. Chem., 2011, 3, 180–189 CAS.
- T. Yu, F. C. Cheong and C. H. Sow, Nanotechnology, 2004, 15, 1732–1736 CrossRef CAS.
-
(a) A. Umar, M. M. Rahman, A. Al-Hajry and Y. B. Hahn, Electrochem. Commun., 2009, 11, 278–281 CrossRef CAS;
(b) J. Tamaki, K. Shimanoe, Y. Yamada, Y. Yamamoto, N. Miura and N. Yamazoe, Sens. Actuators, B, 1998, 49, 121–125 CrossRef CAS;
(c) T. Ishihara, M. Higuchi, T. Takagi, M. Ito, H. Nishiguchi and T. Takita, J. Mater. Chem., 1998, 8, 2037–2042 RSC.
-
(a) P. Podhajecky, B. Klapste, P. Novak, J. Mrha, R. Moshtev, V. Manev and A. Nassalevska, J. Power Sources, 1985, 14, 269–275 CrossRef CAS;
(b) Y. Zhao, S. Mu, W. Sun, Q. Liu, Y. Li, Z. Yan, Z. Huo and W. Liang, Nanoscale, 2016, 8, 19994–20000 RSC.
-
(a) P. Kumar, A. G. Nene, S. Punia, M. Kumar, Z. Abbas, F. Thakral and H. S. Tuli, Int. J. Appl. Pharm., 2020, 12, 17–20 CAS;
(b) A. Radhakrishnan, P. Rejani and B. Beena, Int. J. Nano Dimens., 2014, 5, 519–524 Search PubMed;
(c) H. Palza, Int. J. Mol. Sci., 2015, 16, 2099–2116 CrossRef CAS PubMed;
(d) S.-S. Gabriela, F.-R. Daniela and B.-T. Helia, Int. J. Odontostomat., 2016, 10, 547–554 CrossRef;
(e) H. Qamar, S. Rehman, D. Kumar, C. Ashok, K. Tiwari and V. Upmanyu, Int. J. Nanomed., 2020, 15, 2541–2553 CrossRef CAS;
(f) W. M. Mohammed, T. H. Mubark and R. M. S. Al-Haddad, Int. J. Appl. Eng. Res., 2018, 13, 10559–10562 Search PubMed;
(g) M. S. Usman, M. E. E. Zowalaty, K. Shameli, N. Zainuddin, M. Salama and N. A. Ibrahim, Int. J. Nanomed., 2013, 8, 4467–4479 Search PubMed;
(h) S. Jadhav, S. Gaikwad, M. Nimse and A. Rajbhoj, J. Cluster Sci., 2011, 22, 121–129 CrossRef CAS;
(i) P. G. Bhavyasree and T. S. Xavier, Heliyon, 2020, 6, e03323 CrossRef CAS;
(j) M. Ahamed, H. A. Alhadlaq, M. A. Majeed Khan, P. Karuppiah and N. A. Al-Dhabi, J. Nanomater, 2014, 17, 1–5 Search PubMed;
(k) M. Taran, M. Rad and M. Alavi, Pharm. Sci., 2017, 23, 198–206 CrossRef;
(l) H. L. Karlsson, P. Cronholm, J. Gustafsson and L. Moller, Chem. Res. Toxicol., 2008, 21, 1726–1732 Search PubMed;
(m) J. Zhao, Z. Wang, Y. Dai and B. Xing, Water Res., 2013, 47, 4169–4178 CrossRef CAS PubMed.
- R. V. Kumar, R. Elgamiel, Y. Diamant, A. Gedanken and J. Norwig, Langmuir, 2001, 17, 1406–1410 CrossRef.
-
(a) I. Safarik and M. Safarikova, Mater. Sci. Eng., 2002, 133, 737–759 CAS;
(b) M. Moradi, M. Abdolhosseini, A. Zarrabi and B. Johari, Nano-Struct. Nano-Objects, 2019, 19, 100348 CrossRef CAS.
-
(a) C. Lourenco, M. Teixeira, S. Simões and R. Gaspar, Int. J. Pharm., 1996, 138, 1–12 CrossRef CAS;
(b) A. R. Studart, E. Amstad and L. J. Gauckler, Langmuir, 2007, 23, 1081–1090 CrossRef CAS PubMed;
(c) A. A. Thorat and S. V. Dalvi, Chem. Eng. J., 2012, 181–182, 1–34 CrossRef CAS.
-
(a) J. Sun and H. Tan, Materials, 2013, 6, 1285–1309 CrossRef CAS PubMed;
(b) A. Serrano-Aroca, J. F. Ruiz-Pividal and M. Llorens-Gamez, Sci. Rep., 2017, 7, 11684 CrossRef PubMed;
(c) N. Ninan, M. Muthiah, I. K. Park, T. W. Wong, S. Thomas and Y. Grohens, Polym. Rev., 2015, 55, 453–490 CrossRef CAS;
(d) D. Leal, B. Matsuhiro, M. Rossi and F. Caruso, Carbohydr. Res., 2008, 343, 308–316 CrossRef CAS;
(e) N. T. Anh, D. V. Phu, N. N. Duy, B. D. Du and N. Q. Hien, Radiat. Phys. Chem., 2010, 79, 405–408 CrossRef.
- J. Diaz-Visurraga, C. Daza, C. Pozo, A. Becerra, C. Plessing and A. Garcia, Int. J. Nanomed., 2012, 7, 3597–3612 CrossRef CAS PubMed.
- M. Safaei and M. Taran, J. Appl. Polym. Sci., 2018, 135, 45682 CrossRef.
-
V. U. Siddique, I. Khan, A. Ansari, W. A. Siddique and M. K. Akram, In: Gupta B., Ghosh A., Suzuki A., Rattan S. ed. Advances in Polymer Sciences and Technology. Materials Horizons: From Nature to Nanomaterials. Springer, Singapore, 2018 Search PubMed.
- M. Safaei, M. Taran and M. M. Imani, Mater. Sci. Eng., C, 2019, 101, 323–329 CrossRef CAS.
- M. H. Kudzin, M. Bogoń, Z. Mrozińska and A. Kaszmarek, Mar. Drugs, 2020, 18, 660 CrossRef CAS.
- C. G. Gomez, M. V. P. Lambrecht, J. E. Lozano, M. Rinaudo and M. A. Villar, Int. J. Biol. Macromol., 2009, 44, 365–371 CrossRef CAS PubMed.
- R. M. Mohamed, F. A. Harraz and A. Shawky, Ceram. Int., 2014, 40, 2127–2133 CrossRef CAS.
- M. Ohanishi, A. Kusachi, S. Kobayashi and J. Yamakawa, J. Mineral. Petrol. Sci., 2007, 102, 233–239 CrossRef.
- D. Leal, B. Matsuhiro, M. Rossi and F. Caruso, Carbohydr. Res., 2008, 343, 308–316 CrossRef CAS.
- W. Kang, C. Zhao and Q. Shen, Int. J. Electrochem. Sci., 2012, 7, 8194–8204 CAS.
- N. A. Smith, N. Sekido, J. H. Perepezko, A. B. Ellis and W. C. Crone, Scr. Mater., 2004, 51, 423–426 CrossRef CAS.
-
(a) B. Pal, S. S. Mallick and B. Pal, Colloids Surf., A, 2014, 459, 282–289 CrossRef CAS;
(b) J. Zhu, D. Li, H. Chen, X. Yang, L. Lu and X. Wang, Mater. Lett., 2004, 58, 3324–3327 CrossRef CAS.
- A. Sirelkhatim, S. Mahmud, A. Seeni, N. H. Mohamad Kaus, L. I. Chuo Ann, S. K. H. Mohd Bakhori, H. Hasan and D. Mohamad, Nano-Micro Lett., 2015, 7, 219–242 CrossRef CAS PubMed.
-
(a) D. P. Dubal, G. S. Gund, R. Holze, H. S. Jadhav, C. D. Lokhande and C.-J. Park, Dalton Trans., 2013, 42, 6459–6467 RSC;
(b) M. Thommes, Chem. Ing. Tech., 2010, 82, 1059–1073 CrossRef CAS;
(c) S.-S. Chang, B. Clair, J. Ruelle, J. Beauchêne, F. D. Renzo, F. Quignard, G.-J. Zhao, H. Yamamoto and J. Gril, J. Exp. Bot., 2009, 60, 3023–3030 CrossRef CAS.
-
(a) R. G. Kadam, M. Petr, R. Zbořil, M. B. Gawande and R. V. Jayaran, ACS Sustainable Chem. Eng., 2018, 6, 12935–12945 CrossRef CAS;
(b) C. Tamuly, I. Saikia, M. Hazarika and M. R. Das, RSC Adv., 2014, 4, 53229–53236 RSC.
- A. S. Bhosale, K. K. Abitkar, P. S. Sadalage, K. D. Pawar and K. M. Garadkar, J. Mater. Sci.: Mater. Electron., 2021, 32, 20510–20524 CrossRef CAS.
- H. Palza, Int. J. Mol. Sci., 2015, 16, 2099–2116 CrossRef CAS.
- C. L. Dupont, G. Grass and C. Rensing, Metallomics, 2011, 3, 1109–1118 CrossRef CAS.
- P. S. Harikumar and A. Aravind, Int. J. Sci., 2016, 2, 83–90 Search PubMed.
-
(a) Y. Yang, J. Lan and J. You, Chem. Rev., 2017, 117, 8787–8863 CrossRef CAS PubMed;
(b) P. Ruiz-Castillo and S. T. Buchwald, Chem. Rev., 2016, 116, 12564–12649 CrossRef CAS;
(c) A. Biffis, P. Centomo, A. D. Zotto and M. Zecca, Chem. Rev., 2018, 118, 2249–2295 CrossRef CAS;
(d) F.-S. Han, Chem. Soc. Rev., 2013, 42, 5270–5298 RSC.
-
(a) S. V. Ley and A. W. Thomas, Angew. Chem., Int. Ed., 2003, 42, 5400–5449 CrossRef CAS;
(b) R. Gujadhur, D. Venkataraman and J. T. Kintigh, Tetrahedron Lett., 2001, 42, 4791–4793 CrossRef CAS;
(c) J. F. Marcoux, S. Doye and S. L. Buchwald, J. Am. Chem. Soc., 1997, 119, 10539–10540 CrossRef CAS.
-
(a) G. W. Wang, T. T. Yuan and D. D. Li, Angew. Chem., Int. Ed., 2011, 50, 1380–1383 CrossRef CAS PubMed;
(b) X. X. Zhang, W. T. Teo and P. W. H. Chan, J. Organomet. Chem., 2011, 696, 331–337 CrossRef CAS.
-
(a) C. L. Allen and J. M. J. Williams, Chem. Soc. Rev., 2011, 40, 3405–3415 RSC;
(b) E. Valeur and M. Bradely, Chem. Soc. Rev., 2009, 38, 606–631 RSC;
(c) J. M. Li, F. Xu, Y. Zhang and Q. Shen, J. Org. Chem., 2009, 74, 2575–2577 CrossRef CAS PubMed;
(d) R. Vanjari, T. Guntreddi and K. N. Singh, Org. Lett., 2013, 15, 4908–4911 CrossRef CAS PubMed.
- P. S. Kumar, G. S. Kumar, R. A. Kumar and K. R. Reddy, Eur, J. Org. Chem., 2013, 1218–1222 CAS.
- Y.-X. Xie, R.-J. Song, X.-H. Yang, J.-N. Xiang and J.-H. Li, Eur, J. Org. Chem., 2013, 5737–5742 CAS.
- M. Albert-Soriano and I. M. Pastor, Eur. J. Org. Chem., 2016, 5180–5188 CrossRef CAS.
- R. G. Kadam, M. Petr, R. Zbořil, M. B. Gawande and R. V. Jayaram, ACS Sustainable Chem. Eng., 2018, 6, 12935–12945 CrossRef CAS.
-
(a) J. Rothenberg, L. Feldberg, H. Wiener and Y. Sasson, J. Chem. Soc., Perkin Trans. 2, 1998, 2429–2443 RSC;
(b) D. J. Rawlinson and B. M. Humke, Tetrahedron Lett., 1972, 13, 4395–4398 CrossRef;
(c) Y. Zhu and Y. Wei, Eur. J. Org. Chem., 2013, 4503–4508 CrossRef CAS;
(d) J. Wang, C. Liu, J. Yuan and A. Lei, Angew. Chem., Int. Ed., 2013, 52, 2256–2259 CrossRef CAS.
|
This journal is © The Royal Society of Chemistry and the Centre National de la Recherche Scientifique 2022 |
Click here to see how this site uses Cookies. View our privacy policy here.