DOI:
10.1039/D1NJ04251C
(Paper)
New J. Chem., 2022,
46, 407-418
Transition metal phosphide of nickel and cobalt-modified Zn0.5Cd0.5S for efficient photocatalytic hydrogen evolution with visible light irradiation†
Received
5th September 2021
, Accepted 14th November 2021
First published on 16th November 2021
Abstract
Transition metal phosphides (TMPs), as an environmentally friendly non-noble metal cocatalyst, play a key role in promoting photocatalytic hydrogen evolution. In this work, three metal phosphating compounds (NiCoP, Ni2P, and Co2P) were synthesized by solvothermal method using red phosphorus as a phosphorus source. The three kinds of co-catalysts were individually loaded onto Zn0.5Cd0.5S by physical agitation method to form binary nanocomposites (ZnCdS/TMPs). The results showed that the three composite photocatalysts all showed excellent HER performance in the Na2S/Na2SO3 sacrifice solution. Among them, the hydrogen production rate of ZnCdS/NiCoP-5% reached 64 μmol at 5 h, which was significantly higher than that of pure Zn0.5Cd0.5S, ZnCdS/Ni2P-5%, and ZnCdS/Co2P-5%. According to the results of various characterization techniques, NiCoP nanoparticles provide more active sites for Zn0.5Cd0.5S and the composite photocatalytic materials exhibit excellent hydrogen evolution performance. Moreover, the Schottky barrier between Zn0.5Cd0.5S and NiCoP nanoparticles effectively enhances the charge separation rate, thus promoting the improvement of photocatalytic hydrogen evolution. Therefore, low-cost transition metal phosphide as a cocatalyst has broad prospects in the field of photocatalysis.
1. Introduction
As a clean energy source, hydrogen energy has the advantages of being environmentally friendly and having high energy density, which has attracted extensive attention and research of scientists.1–3 Among them, semiconductor photocatalytic hydrogen production technology is the most commonly used method to solve environmental and energy problems.4,5 At present, semiconductors that have been discovered include metal sulfides (ZnIn2S46,7) and metal oxides (CaTiO3,8 ZnO9,10). Therefore, the exploration of a low cost and environmental protection semiconductor photocatalyst can effectively improve the photocatalytic hydrogen evolution rate.
Metal sulfide semiconductors have attracted much attention due to their suitable band gap and excellent photocatalytic hydrogen evolution performance.11,12 As a kind of metal sulfide, Zn0.5Cd0.5S has outstanding hydrogen production performance.13,14 Zn0.5Cd0.5S easily causes photocorrosion. This is because the valence band of Zn0.5Cd0.5S is less electronegative, which leads to the autooxidation of the holes generated by light excitation.15,16 In addition, in the study of ZnCdS, the problem of a photoexcited electron–hole pair rapid recombination needs to be further improved, so as to effectively improve the charge separation efficiency and hydrogen evolution activity. Therefore, the supported cocatalyst can effectively inhibit the recombination of electron holes in the Zn0.5Cd0.5S solid solution, thus promoting the improvement of its hydrogen evolution performance. Therefore, the exploration of a cocatalyst without precious metals has become the main method to solve this problem.17
However, the most effective cocatalysts currently rely on noble metals, but the high cost of precious metals limits their large-scale application. Therefore, it has been reported that transition metal phosphide is widely used in electrocatalysis due to its excellent electrical conductivity and stability, such as Ni2P,18 Co2P19 and FeP.20 Compared with transition metal oxides, transition metal phosphide has the characteristics of low cost, easy access and high catalytic performance. According to previous studies, Ni2P as a co-catalyst of one-dimensional semiconductor nanorods can effectively improve the photocatalytic hydrogen evolution efficiency of composite photocatalysts.21 Yu et al. reported the performance comparison of Ni0.1Co0.9P with Ni2P and CoP, and the results showed that Ni0.1Co0.9P exhibited the most significant catalytic performance in neutral water.22 In addition, MoP was used as a co-catalyst to support the light-absorbent CdS, and an efficient system of photocatalytic hydrogen evolution without noble metals was constructed, which was similar to the rate of hydrogen evolution when Pt was used as a co-catalyst.23
In this work, a series of ZnCdS/TMPs composite photocatalysts were successfully prepared by solvothermal method and physical agitation method. The effects of Ni2P, Co2P and NiCoP as co-catalysts on the hydrogen evolution performance were studied. Under simulated solar irradiation, the ZCS/NCP-5% composite photocatalyst with Na2S/Na2SO3 as a sacrificial agent had the highest hydrogen production rate, and its 5 h hydrogen production activity reached 64 μmol, which was significantly higher than that of pure Zn0.5Cd0.5S, ZCS/NP-5% and ZCS/CP-5%. A series of characterization studies showed that TMPs nanoparticles, as a cocatalyst, not only effectively improved the active hydrogen evolution site of Zn0.5Cd0.5S, but also improved the charge separation efficiency of Zn0.5Cd0.5S, thus effectively enhancing the hydrogen evolution performance of ZnCdS/TMPs composite photocatalyst.
2. Experimental section
2.1 Materials
The chemicals used in this experiment are nickel chloride hydrate (NiCl2·6H2O), sodium dodecyl benzene sulfonate (SDBS), cobaltous nitrate hexahydrate (Co(NO3)2·6H2O), red phosphorus, hexadecyl trimethyl ammonium bromide (CTAB), thiourea (CH4N2S), zinc nitrate hexahydrate (Zn(NO3)2·6H2O), sodium sulfide nonahydrate (Na2S·9H2O), cadmium nitrate tetrahydrate (Cd(NO3)2·4H2O), and sodium sulfite (Na2SO3).
2.2 Sample preparation
2.2.1 The preparation of Zn0.5Cd0.5S.
Zn0.5Cd0.5S was prepared by hydrothermal method. 0.5950 g Zn(NO3)2·6H2O, 0.6169 g Cd(NO3)2·4H2O and 0.3806 g thiourea were dissolved in 60 mL distilled water. After stirring for 0.5 h, the mixed solution was placed in a 100 mL Teflon lined reactor at 180 °C for 15 h. The solution was cooled and filtered to obtain Zn0.5Cd0.5S.
2.2.2 Preparation of TMPs (NiCoP, Ni2P and Co2P) nanoparticles.
1.5 g red phosphorus was added to a 100 mL Teflon lined reactor. A volume of 60 mL deionized water was added and the mixture was stirred for 30 min. Then, the reactor was placed in an oven at 200 °C for 12 h. The solution was cooled and filtered to obtain pure RP.
1.1188 g NiCl2·6H2O, 0.4365 g Co(NO3)2·6H2O, 0.7743 g pure RP, 0.1 g CTAB and 0.1 g SDBS were dissolved in a 50 mL mixture of deionized water and ethanol (volume ratio is 1
:
1). After stirring for 1 h, the mixed solution was placed in a 100 mL Teflon lined reactor in 200 °C for 24 h. The solution was cooled and filtered to obtain the NiCoP nanoparticles. Ni2P and Co2P nanoparticles were synthesized by the same method, except for hydrothermal temperature and time. Ni2P can be synthesized by reacting for 12 h at 140 °C, while Co2P can be synthesized by reacting for 10 h at 200 °C.
2.2.3 The preparation of ZCS/TMP-X.
0.1 g of the above prepared Zn0.5Cd0.5S was taken and NiCoP nanoparticles with percentages of 1%, 5%, 10%, 15% and 20% were separately added. The mixed solution was ultrasonicated for 5 min and stirred for 3 h. Finally, the composite catalysts ZCS/NCP-X were obtained after washing and filtration with deionized water, and they were named as ZCS/NCP-1%, ZCS/NCP-5%, ZCS/NCP-10%, and ZCS/NCP-15%. Similarly, ZCS/NP-5% and ZCS/CP-5% of Zn0.5Cd0.5S with 5% Ni2P and 5% Co2P were also prepared.
2.3 Characterization
The crystal diffraction (XRD) data of the photocatalyst were determined by an X-ray diffractometer (Rigaku SmartLab). The morphology, structure and elemental composition of the samples were analyzed by scanning electron microscopy (SEM, Zeiss EVO 18), transmission electron microscopy (TEM, JEM-2100) and energy dispersive X-ray spectroscopy (EDX), respectively. X-Ray photoelectron spectroscopy (XPS) measurements were obtained with a Thermo Escalab 250XI instrument. The UV-Vis spectra (UV-Vis) of the sample was recorded by an UV-Vis (UV2550, Shimadu) apparatus. Nitrogen adsorption–desorption tests were performed on the samples using a Micromeritics ASAP 2020 instrument. Stable state photoluminescence (PL) and time-resolved fluorescence (TRF) of the catalyst were measured using a Fluoromax-4 instrument.
2.4 Photocatalytic H2 evolution experiments
The experiment of photocatalytic hydrogen production was conducted in a multichannel illuminated photocatalytic reaction system with a 5 W LED light source. A mixture of 10 mg catalyst powder and 30 mL Na2S·9H2O and Na2SO3 was placed in a quartz glass reactor. After agitation and ultrasound for 10 min, the mixed solution was degassed with nitrogen for 5 min. The amount of H2 produced was measured with a gas chromatograph (Ruili SP-2100) every hour after irradiation. The gas chromatograph was a 13 X Zeolite packed column, and N2 was used as the carrier gas. Under the same condition, the cycle stability experiment was also carried out in order to compare the hydrogen production effect between ZnCdS/TMPs and the composite photocatalysis supported by the Pt cocatalyst. H2PtCl6 solution and Na2S/Na2SO4 as electron sacrificial agents were used to test the hydrogen production performance of ZnCdS/Pt.
The apparent quantum yield (AQY) of the catalyst was measured under a 300 W xenon lamp. The experiment was conducted in a closed quartz glass reaction flask, in which 0.05 g catalyst was added into a quartz reaction flask containing 100 mL Na2S/Na2SO4 solution for ultrasonic agitation. The reaction flask was degasified with nitrogen for 10 min and the light source was a 300 W xenon lamp, with a fixed wavelength monochromatic light every 1 h for the hydrogen production test. A total wavelength range of 450–550 nm was used for the hydrogen production rate with the AQY calculation formula below:37
2.5 Photoelectrochemical experiments
The electrochemical properties of different catalysts were measured in 0.2 M sodium sulfate electrolyte solution using a three-electrode electrochemical workstation (CHI660E). A saturated silver chloride electrode (Ag/AgCl) and platinum wire were used as the counter electrode and reference electrode, respectively. The catalyst powder was dispersed in a mixture of 5% Nafion solution and ethanol, and then the suspension was coated onto ITO glass with an effective area of 1.0 cm2 as the working electrode. The photocurrent response curves (I–T) of different catalysts were measured during the switching cycle of a 300 W xenon lamp. In addition, the electrochemical impedance (EIS) and scanning linear voltammetry (LSV) curves of different catalysts were measured.
3. Results and discussion
3.1 Crystal structure analysis (XRD)
In Fig. 1(a), the crystal structures of the NiCoP, Zn0.5Cd0.5S and ZCS/NCP-5% photocatalysts were studied by XRD patterns. The diffraction peaks of NiCoP at 40.99°, 44.90°, 47.58° and 54.44° correspond to the (111), (201), (210) and (300) crystal faces of the NiCoP standard card (PDF#71-2336), respectively,24 indicating the successful synthesis of NiCoP. Similarly, in Fig. 1(b), the diffraction peaks of Ni2P and Co2P correspond to the standard cards (PDF#74-1385) and (PDF#32-0306), respectively.25 Meanwhile, the Zn0.5Cd0.5S in the figure corresponds to the characteristic peak of hexagonal phase CdS. Among them, 24.81°, 26.51°, 28.18°, 43.68° and 51.82° correspond to the crystal faces of (100), (002), (101), (110) and (112) of the CdS standard card (PDF#41-1049), respectively. Since the radius of Cd2+ was larger than that of Zn2+, the introduction of Zn2+ into the lattice of CdS reduces the lattice fringe distance of CdS, resulting in a large angular displacement of the peak position of Zn0.5Cd0.5S. These characteristics indicate the formation of the ZnXCd1-XS solid solution. The result conforms to Vegard's law.26,27 Because the content of NiCoP in the ZCS/NCP-5% composite catalyst was very small, the (111) crystal plane belonging to NiCoP shows relatively weak characteristic peaks. In addition, no diffraction peaks with other characteristics were observed in the composite photocatalysts ZCS/NCP-5%, ZCS/NP-5% and ZCS/CP-5%, indicating that no other impurities were generated.
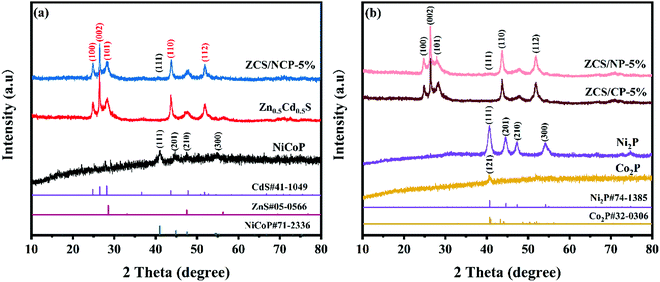 |
| Fig. 1 The XRD patterns of (a) NiCoP, Zn0.5Cd0.5S, ZCS/NCP-5% and (b) Ni2P, Co2P, ZCS/NP-5%, ZCS/CP-5%. | |
3.2 SEM and TEM analysis
In Fig. 2, SEM images were used to analyze the microscopic morphology of the sample. As shown in Fig. 2(a), Zn0.5Cd0.5S is a leaf-like structure with a length of 4–6 μm.27 It can be observed from Fig. 2(b–d) that the Ni2P, Co2P and NiCoP nanoparticles have similar structures with an average diameter of about 0.1–1 μm. In Fig. 2(e), it is obvious that the Ni2P nanoparticles are distributed on the Zn0.5Cd0.5S surface. In particular, it can be found from Fig. 2(f and g) that both Co2P and NiCoP are distributed on Zn0.5Cd0.5S. The microstructure of ZCS/NCP-5 was analyzed by TEM and HRTEM, as shown in Fig. 3. In Fig. 3(a and b), Zn0.5Cd0.5S has good interface contact with the NiCoP nanoparticles. In particular, lattice fringes with lattice spacing of 0.34 nm and 0.21 nm correspond to the (002) and (110) crystal faces of Zn0.5Cd0.5S, respectively. The lattice fringes with lattice spacing of 0.22 nm belong to the (110) plane of NiCoP. The results are consistent with the XRD diffraction peaks of Zn0.5Cd0.5S and NiCoP. According to the EDX diagram in Fig. 3(c), it is proved that Zn, Cd, S, P, Ni and Co elements are present in the composite catalyst. In addition, the distribution of each element is observed from the elemental mapping in Fig. 3(d–j). Due to the small content of NiCoP in the composite catalyst, the strength of elements Ni, Co and P is lower than that of elements Zn, Cd and S.
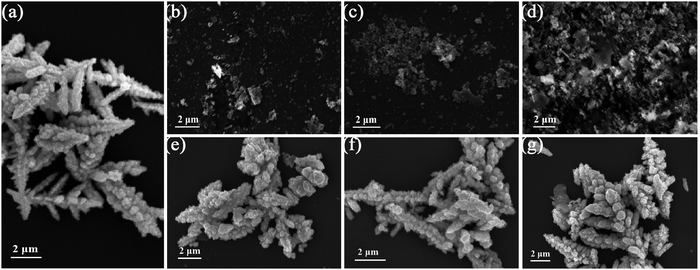 |
| Fig. 2 SEM image of (a) Zn0.5Cd0.5S, (b) Ni2P, (c) Co2P, (d) NiCoP, (e) ZCS/NP-5%, (f) ZCS/CP-5%, and (g) ZCS/NCP-5%. | |
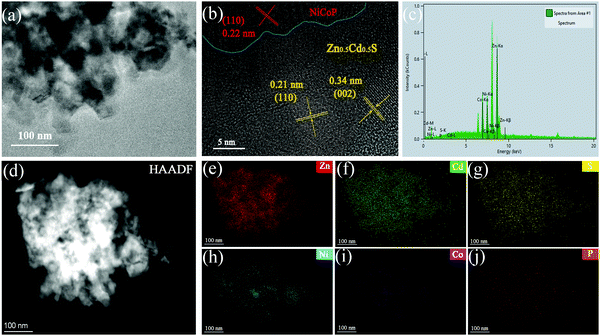 |
| Fig. 3 TEM images of (a) ZCS/NCP-5%, (b) HRTEM image of ZCS/NCP-5%, (c) EDX of ZCS/NCP-5%, (d) HAADF of ZCS/NCP-5%. (e–j) Elemental-mapping of Zn, Cd, S, Ni, Co and P elements. | |
3.3 XPS analysis
In order to further analyze the elemental composition and valence state of the sample, we carried out XPS tests on the sample. Fig. 4(a) shows the XPS spectrum calibrated by C 1s (282.8 eV). The full spectrum chart shows that the samples are mainly composed of Zn, Cd, S, Ni, P and Co elements. In Fig. 4(b), the spin–orbit splitting peaks of 1044.3 eV and 1021.4 eV corresponded to Zn 2p1/2 and Zn 2p3/2, respectively. This effectively indicates the presence of Zn2+.17,28Fig. 4(c) shows the map of Cd 3d, which was similar to that of Zn 2p. There were two strong peaks at 411.2 eV and 403.9 eV, corresponding to Cd 3d3/2 and Cd 3d5/2, respectively. This proves the presence of Cd2+.29Fig. 4(d) shows the fine spectrum of S 2p for ZCS/NCP-5%. The spin–orbit splitting peaks at 161.4 eV and 160.1 eV correspond to S 2p1/2 and S 2p3/2, respectively, proving the existence of S2−. The smaller twin peaks of 166.9 eV and 167.8 eV represent S22−.30 Compared with Zn0.5Cd0.5S, the XPS peaks of Zn 2p, Cd 3d and S 2p spectra move towards the direction of lower binding energy. These results indicate that Zn0.5Cd0.5S has strong electron interaction with NiCoP nanoparticles, which greatly improves the charge transfer rate and hydrogen evolution performance of the ZCS/NCP-5% composite photocatalyst.27,34
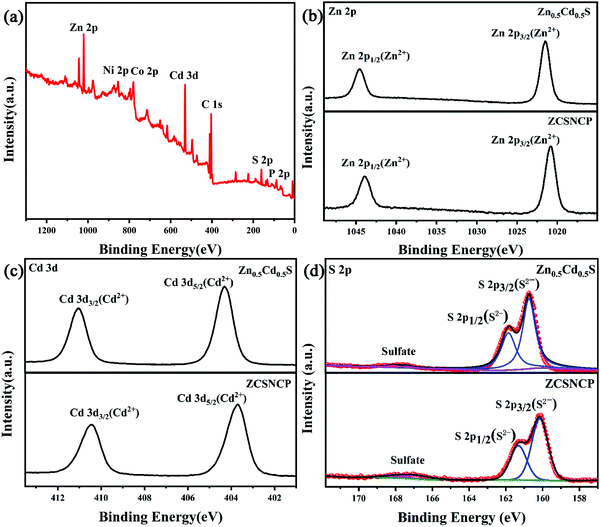 |
| Fig. 4 (a) XPS survey spectrum of ZCS/NCP-5%; (b–d) XPS spectra of Zn 2p, Cd 3d, and S 2p for different photocatalysts. | |
In the diagram of Ni in Fig. 5(a), Ni 2P3/2 has two pairs of spin splitting peaks of 852.5 eV and 854.9 eV. The spin splitting peaks at 879.1 eV and 872.6 eV correspond to Ni 2p1/2. Among them, the subpeaks at 852.5 eV and 879.1 eV effectively prove the existence of Ni2+, while the peaks at 854.9 eV and 872.6 eV effectively prove the existence of Ni3+.31 In Fig. 5(b), the Co element has an optional split slit similar to that of the Ni element. The spin splitting peaks at 777.9 eV and 780.7 eV correspond to Co 2p3/2. The spin-splitting peaks at 792.9 eV and 796.6 eV correspond to Co 2p1/2. Among them, the subpeaks of 777.9 eV and 792.9 eV represent the existence of Co3+.32 In Fig. 5(c), the peaks at 128.8 eV, 129.2 eV and 131.8 eV correspond to P 2p3/2, P 2p1/2 and P oxide on the sample surface, respectively.33
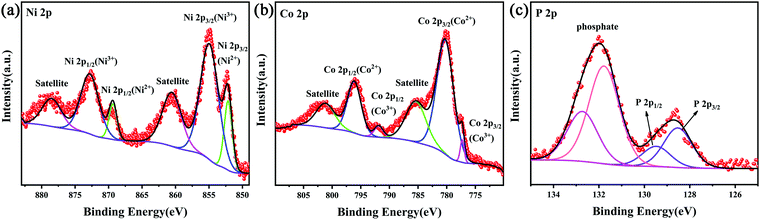 |
| Fig. 5 (a–c) XPS spectra of Ni 2p, Co 2p and P 2p for ZCS/NCP-5%. | |
3.4 BET analysis
As shown in Fig. 6, the N2 adsorption–desorption isothermal curve of the sample provides a basis for studying the specific surface area of the photocatalyst. In Fig. 6(a–c), all catalysts exhibit typical type IV isotherms. In addition, they all have H3 type hysteresis loops, indicating that Zn0.5Cd0.5S, NiCoP and the composite photocatalyst ZCS/NCP-5% are mesoporous structures. As shown in Table 1, the specific surface areas of Zn0.5Cd0.5S, NiCoP and the composite photocatalyst ZCS/NCP-5% were 11.902 m2 g−1, 41.088 m2 g−1 and 16.416 m2 g−1, respectively. After loading NiCoP on Zn0.5Cd0.5S, the specific surface area of Zn0.5Cd0.5S was effectively increased. Similarly, the average aperture of ZCS/NCP-5% was also improved compared to Zn0.5Cd0.5S. Therefore, the addition of NiCoP can effectively improve the specific surface area of Zn0.5Cd0.5S, thus contributing to the improvement of the hydrogen evolution activity of the ZCS/NCP-5% composite photocatalyst.
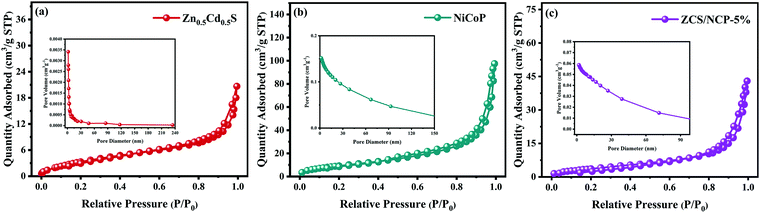 |
| Fig. 6 (a–c) N2 adsorption–desorption isotherms and BJH adsorption mean pore sizes of Zn0.5Cd0.5S, NiCoP and ZCS/NCP-5% samples. | |
Table 1 The SBET, pore volume and average pore size of different samples
Samples |
S
BET
(m2 g−1) |
Pore volumeb (cm3 g−1) |
Average pore sizec (nm) |
BET specific surface area.
BJH adsorption cumulative volume of pores.
BJH adsorption average pore diameter.
|
Zn0.5Cd0.5S |
11.902 |
0.030 |
10.020 |
NiCoP |
41.088 |
0.152 |
14.786 |
ZCS/NCP-5% |
16.416 |
0.059 |
14.315 |
3.5 UV-Vis DRS analysis
UV-Vis spectra provide a basis for studying the light absorption properties of different photocatalysts. Generally speaking, the stronger the light absorption intensity of photocatalyst, the more easily electrons are excited by light, which is favorable for the photoexcited carrier transfer and hydrogen evolution of the photocatalyst.34 As shown in Fig. 7(a), NiCoP, Ni2P and Co2P show strong light absorption in the whole band because they were black powders. The absorbance edge of pure Zn0.5Cd0.5S was about 600 nm. After loading TMPs nanoparticles on the photocatalyst Zn0.5Cd0.5S, the absorption of visible light by Zn0.5Cd0.5S was significantly improved.25,35 The band gaps of different samples were obtained by converting the UV-Vis DRS data into Tauc plots using eqn (1).36Fig. 7(b) clearly shows that the band gaps of Zn0.5Cd0.5S is 2.21 eV.where a is the absorption coefficient; A is a constant; hν is the photon energy; and Eg is the band-gap energy.
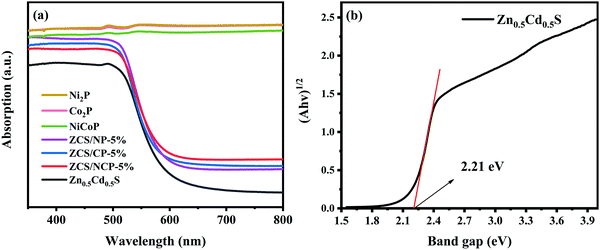 |
| Fig. 7 (a) UV-Vis DRS of different catalysts. (b) Tauc plots of different catalysts. | |
3.6 Photocatalytic hydrogen evolution
The hydrogen evolution performance of different photocatalysts was measured with Na2S/Na2SO3 as a sacrificial agent. As shown in Fig. 8(a), Zn0.5Cd0.5S showed weak photocatalytic hydrogen evolution performance, and its hydrogen evolution rate reached 3.54 μmol h−1. This corresponds to its weak light capture ability in the ultraviolet spectrum and low electron–hole pair separation ability in the fluorescence spectrum. The hydrogen evolution activity of the ZCS/NP-5%, ZCS/CP-5% and ZCS/NCP-5% composite photocatalysts was significantly increased by loading Ni2P, Co2P and NiCoP nanoparticles onto the Zn0.5Cd0.5S surface, respectively. The hydrogen evolution rate in ZCS/NCP-5% was significantly higher than that in ZCS/NP-5% and ZCS/CP-5%, reaching 12.8 μmol h−1. In Fig. 8(b), the hydrogen evolution performance of the composite photocatalyst ZCS/TMPs was further optimized by adjusting the molar ratio of Zn0.5Cd0.5S to NiCoP. It is obvious in the figure that when NiCoP load reaches 5%, the hydrogen yield of the ZCS/NCP-5% composite photocatalyst reaches the highest. As the NiCoP loading continued to increase, the H2 evolution rate of the composite catalyst decreased significantly. This is because the excess NiCoP load can obscure the active site of Zn0.5Cd0.5S. It is clear from Fig. 8(c) that the stability of the ZCS/NCP-5% photocatalytic hydrogen evolution performance has not significantly decreased in the five cycle experiments, proving that the composite catalyst has good stability. In particular, Fig. 8(d) shows the XRD patterns of ZCS/NCP-5% before and after hydrogen production, which were compared to further prove that ZCS/NCP-5% has good stability.
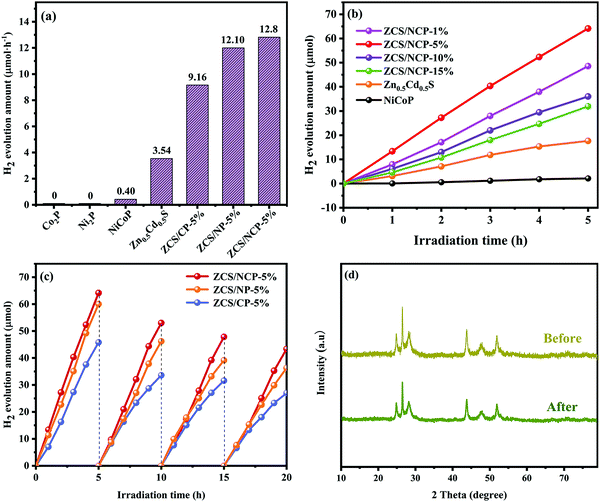 |
| Fig. 8 (a and b) H2 evolution of the different photocatalyst; (c) cycle test of the ZCS/TMPs-5%; (d) XRD patterns of the ZCS/NCP-5% before and after H2 production. | |
As shown in Fig. 9, we measured the apparent quantum efficiency (AQY) at 450 nm, 475 nm, 500 nm, 520 nm and 550 nm, among which the AQY at 500 nm was the highest, reaching 1.32%. It can also be seen from the figure that the variation trend of the AQY value is consistent with the light absorption characteristics of the photocatalyst.37–39
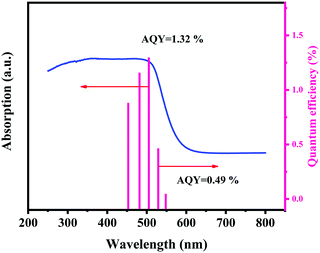 |
| Fig. 9 AQY of ZCS/NCP-5%. | |
3.7 PL analysis
The charge separation ability of the photocatalyst is an important factor to determine its photocatalytic performance. The PL spectra results show the charge separation ability of the composite photocatalyst. As the sample is irradiated by light of a certain energy, the ground state electrons of the sample will transition from the low energy level to high energy level, resulting in electron hole pairs.40 However, the electrons in the excited state will go back to the ground state because of their instability, and that will produce a fluorescence spectrum. Therefore, the lower PL intensity indicates that the excited electrons return to the ground state with fewer electrons, indicating that the charge separation efficiency of the photocatalyst is higher.41 As shown in Fig. 10(a), the composite photocatalyst ZCS/NP-5%, ZCS/CP-5% and ZCS/NCP-5% generated after loading Ni2P, Co2P and NiCoP on Zn0.5Cd0.5S were all lower than that of pure Zn0.5Cd0.5S. This indicates that the introduction of TMPs nanoparticles in Zn0.5Cd0.5S effectively promotes the charge separation. Among them, the peak intensity of ZCS/NCP-5% was the lowest, which further proves that NiCoP can accelerate the separation efficiency of photoexcited carriers of the ZCS/NCP-5% composite photocatalyst, thus promoting the improvement of the hydrogen evolution performance of the composite photocatalyst.
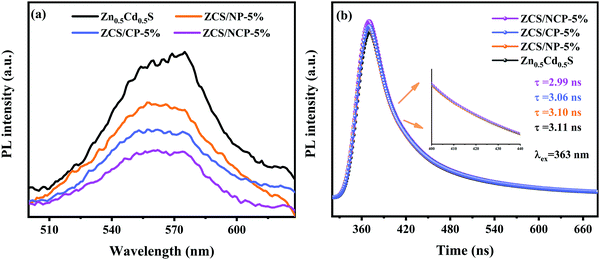 |
| Fig. 10 (a) PL spectra of the Zn0.5Cd0.5S, ZCS/NP-5%, ZCS/CP-5% and ZCS/NCP-5%; (b) TRPL spectra of the Zn0.5Cd0.5S, ZCS/NP-5%, ZCS/CP-5% and ZCS/NCP-5%. | |
The lifetime of the photocatalyst was further determined by the time-resolved transient decay curve of the sample. According to the formula, the decay curve of the sample can be well fitted by using the three-exponential equation, as shown in Fig. 10(b):27
τi: Decay life;
Ai: pre-exponential factor; 〈
τ〉: average life.
The decay curve of TRPL shows that the average life of ZCS/NP-5%, ZCS/CP-5% and ZCS/NCP-5% decrease successively, which were 3.10 ns, 3.06 ns and 2.99 ns, respectively. The main reason for the decrease in the mean lifetime of ZCS/NCP-5% was the effective electron transfer between Zn0.5Cd0.5S and NiCoP, which promotes the separation of the electron hole pairs.40,42,43 Therefore, the ZCS/NCP-5% photocarrier separation rate was the fastest, which was conducive to its hydrogen evolution performance.
3.8 Photoelectrochemical test analysis
The charge separation ability of the photocatalyst was further studied by photochemical experiment. As shown in Fig. 11(a), intermittent irradiation was carried out with a 300 W Xenon lamp light source, and the I–T curves of Zn0.5Cd0.5S, ZCS/CP-5%, ZCS/NP-5% and ZCS/NCP-5% were measured. All photocatalysts show different photocurrent responses under light, but with the continuous accumulation of holes, the photocurrent response intensity decreases slightly.44 In Fig. 11(a), the photocurrent response intensity of the composite photocatalyst ZCSNCP-5% was the highest, indicating that its electron transfer rate was the fastest, which can promote hydrogen evolution performance.
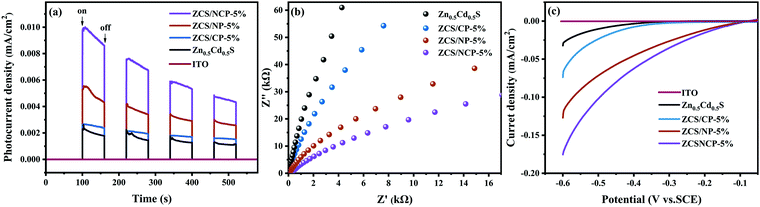 |
| Fig. 11 (a) I–T curves of Zn0.5Cd0.5S, ZCS/CP-5%, ZCS/NP-5%, and ZCS/NCP-5%; (b) Corresponding EIS curves, (c) LSV plots of Zn0.5Cd0.5S, ZCS/CP-5%, ZCS/NP-5%, and ZCS/NCP-5%. | |
In Fig. 11(b), the EIS of different photocatalysts reflect the resistance of the photocatalysts during charge transfer. The smaller the arc radius of the photocatalyst, the smaller the charge transfer resistance in the photocatalyst.45 The composite photocatalyst supported by 5% NiCoP nanoparticles has the smallest radius of arc, indicating that the conductivity of the composite photocatalyst ZCS/NCP-5% was greater than that of ZCS/NP-5% and ZCS/CP-5%. The introduction of NiCoP, a cocatalyst, provides more catalytic active sites for Zn0.5Cd0.5S by rapidly capturing photoelectrons. Therefore, Zn0.5Cd0.5S and NiCoP have excellent electrical conductivity and low resistance, which effectively promote the photocatalytic hydrogen evolution performance.
Fig. 11(c) shows that ZCS/NCP-5% has the highest photocurrent density, indicating that the overpotential of ZCS/NCP-5% was lower than that of Zn0.5Cd0.5S, ZCS/CP-5% and ZCS/NP-5%. The decrease of the overpotential of the hydrogen production was mainly caused by the rapid transfer of electrons between the Zn0.5Cd0.5S and NiCoP interface.44,46 This further indicates that the ZCS/NCP-5% composite photocatalyst has strong activity of hydrogen evolution. In conclusion, the ZCS/NCP-5% composite photocatalyst had a faster charge separation efficiency and a lower recombination efficiency, thus effectively improving the activity of the hydrogen evolution of the photocatalyst.
Fig. 12 shows the Mott–Schottky results for Zn0.5Cd0.5S, NiCoP, Co2P, and Ni2P, and all line plots have positive slopes, indicating that they are typical n-type semiconductors.47 It is obvious from the figure that the flat band potentials of the Zn0.5Cd0.5S, NiCoP, Co2P, and Ni2P are −1.03 eV, −0.64 eV, −0.62 eV and −0.60 eV (relative to SCE), respectively. In general, the conduction band potential (ECB) of the n-type semiconductors is 0.1 V or 0.2 V more negative than the flat band potential.36,48,49 Therefore, the ECB of the Zn0.5Cd0.5S, NiCoP, Co2P, and Ni2P are estimated to be −1.13 eV, −0.74 eV, −0.72 eV and −0.70 eV (relative to SCE), respectively. According to formula,50 the ECB of the Zn0.5Cd0.5S, NiCoP, Co2P, and Ni2P are −0.89 eV, −0.50 eV, −0.48 eV and −0.46 eV (relative to NHE), respectively. According to the band gap value of Zn0.5Cd0.5S at 2.21 V, the valence band potential (EVB) of Zn0.5Cd0.5S is 1.32 eV (relative to NHE).
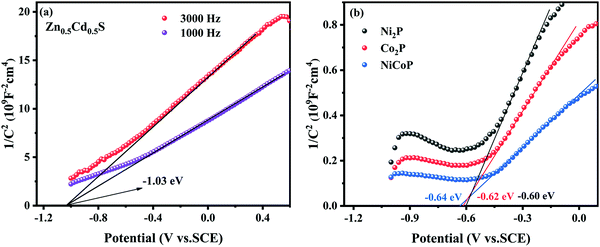 |
| Fig. 12 (a and b) Mott–Schottky plots of Zn0.5Cd0.5S, Ni2P, Co2P, and NiCoP. | |
3.10 Photocatalytic mechanism
Combined with the photoelectric chemistry experiment and fluorescence spectrum, a possible interface charge transfer direction between Zn0.5Cd0.5S and TMPs nanoparticles was proposed, as shown in Fig. 13. In particular, it had been reported that the work function results of Zn0.5Cd0.5S have been characterized by the Kelvin probe method,51 which provides an important basis for studying the direction of the charge transfer. Under visible light irradiation, the photoexcited electrons in the Zn0.5Cd0.5S transfer from the valence band (VB) to the conduction band (CB), leaving holes in the valence band. However, due to the rapid recombination between electrons and holes, the photocatalytic hydrogen evolution performance of Zn0.5Cd0.5S has not been well developed. Compared with Zn0.5Cd0.5S, the XPS peaks of ZCS/NCP-5% Zn 2p, Cd 3d and S 2p move towards the direction of lower binding energy. It is possible that the work function of the TMPs nanoparticles is smaller than that of ZnCdS. Thus, the free electrons are easily transferred from the TMPs nanoparticles to Zn0.5Cd0.5S until their Fermi energy levels reach equilibrium,52 thus forming a Schottky barrier. After simulated visible light irradiation, photoexcited electrons are transferred from Zn0.5Cd0.5S to TMPs nanoparticles, and the electrons on the surface of TMPs reduce H+ to H2, while the VB holes of Zn0.5Cd0.5S are consumed by the sacrifice reagent Na2S/Na2SO3.31,53 On the other hand, TMPs nanoparticles, as a cocatalyst, not only effectively improve the visible light absorption capacity of Zn0.5Cd0.5S, but also increase the active site of the hydrogen evolution and charge transfer rate of Zn0.5Cd0.5S, thus effectively enhancing its photocatalytic hydrogen evolution performance. Compared with Ni2P, Co2P and NiCoP, the composite photocatalytic hydrogen evolution with 5% NiCoP was the best. This is because in most TMPs, the active sites for hydrogen production are in the metal center and phosphating center. When 5% NiCoP nanoparticles were loaded on Zn0.5Cd0.5S, the hydrogen evolution rate of ZCS/NCP-5% was the highest, indicating that NiCoP provided more H+ receptor centers. This also means that NiCoP has more active sites.54,55 Therefore, TMPs nanoparticles loaded on Zn0.5Cd0.5S effectively improve the charge separation efficiency and increase more active sites for photocatalytic hydrogen evolution, thus improving the activity of hydrogen evolution.
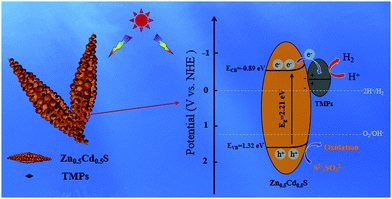 |
| Fig. 13 Schematic diagram of the electron transfer of the ZnCdS/TMPs composite photocatalyst. | |
4. Conclusion
In this work, a series of ZnCdS/TMPs composite photocatalysts were successfully prepared by solvothermal method and physical agitation method. The effects of Ni2P, Co2P and NiCoP as co-catalysts on the hydrogen evolution performance were studied. After loading TMPs nanoparticles on the Zn0.5Cd0.5S nanomaterial, all photocatalysts show good photocatalytic H2 produce performance. Under visible light irradiation, the hydrogen evolution rate of ZCS/NCP-5% reached 12.80 μmol h−1, which was significantly higher than that of pure Zn0.5Cd0.5S (3.54 μmol h−1), ZCS/NP-5% (12.10 μmol h−1) and ZCS/CP-5% (9.16 μmol h−1). According to the results of fluorescence and photoelectrochemical tests, TMPs nanoparticles, as a co-catalyst, not only effectively improve the visible light absorption capacity of Zn0.5Cd0.5S, but also increase the active site of hydrogen evolution and charge transfer rate of Zn0.5Cd0.5S, thus effectively enhancing its photocatalytic hydrogen evolution performance. In particular, compared with Ni2P and Co2P, NiCoP has more active sites, which effectively promotes the charge transfer rate of ZCS/NCP-5%. It provides a new idea for the study of high efficiency and low cost photocatalyst.
Conflicts of interest
There are no conflicts to declare.
Acknowledgements
This work was supported by the Fundamental Research Funds for the Natural Science Foundation of Ningxia Province (2021AAC03180) and the Central Universities of North University for Nationalities (2021KJCX02).
References
- P. Kuang, M. Sayed, J. Fan, B. Cheng and J. Yu, 3D graphene-based H2-Production photocatalyst and electrocatalyst, Adv. Energy Mater., 2020, 10(14), 1903802 CrossRef CAS
.
- Q. Xu, L. Zhang, B. Cheng, J. Fan and J. Yu, S-scheme heterojunction photocatalyst, Chem, 2020, 6(7), 1543–1559 CAS
.
- J. Ge, Y. Liu, D. Jiang, L. Zhang and P. Du, Integrating non-precious-metal cocatalyst Ni3N with g-C3N4 for enhanced photocatalytic H2 production in water under visible-light irradiation, Chin. J. Catal., 2019, 40(2), 160–167 CrossRef CAS
.
- J. Jia, X. Bai and Q. Zhang,
et al., Porous honeycomb-like NiSe2/red phosphorus heteroarchitectures for photocatalytic hydrogen production, Nanoscale, 2020, 12(9), 5636–5651 RSC
.
- B. He, C. Bie, X. Fei, B. Cheng and J. Yu,
et al., Enhancement in the photocatalytic H2 production activity of CdS NRs by Ag2S and NiS dual cocatalysts, Appl. Catal., B, 2021, 288, 119994 CrossRef CAS
.
- P. Jin, L. Wang and X. Ma,
et al., Construction of hierarchical ZnIn2S4@PCN-224 heterojunction for boosting photocatalytic performance in hydrogen production and degradation of tetracycline hydrochloride, Appl. Catal., B, 2021, 284, 119762 CrossRef CAS
.
- Z. Gao, K. Chen and L. Wang,
et al., Aminated flower-like ZnIn2S4 coupled with benzoic acid modified g-C3N4 nanosheets via covalent bonds for ameliorated photocatalytic hydrogen generation, Appl. Catal., B, 2020, 268, 118462 CrossRef CAS
.
- A. Kumar, M. Kumar and V. Rao, Unraveling the structural and morphological stability of oxygen vacancy engineered leaf-templated CaTiO3 towards photocatalytic H2 evolution and N2 fixation reactions, J. Mater. Chem. A, 2021, 9, 17006–17018 RSC
.
- S. Kumar, N. Reddy and H. Kushwaha,
et al., Efficient Electron Transfer across a ZnO-MoS2-Reduced Graphene Oxide Heterojunction for Enhanced Sunlight-Driven Photocatalytic Hydrogen Evolution, ChemSusChem, 2017, 10, 3588–3603 CrossRef CAS PubMed
.
- S. Kumar, A. Kumar and V. Rao,
et al., Defect-Rich MoS2 Ultrathin Nanosheets-Coated Nitrogen-Doped ZnO Nanorod Heterostructures: An Insight into in-Situ-Generated ZnS for Enhanced PhotocatalyticHydrogen Evolution, ACS Appl. Energy Mater., 2019, 2, 5622–5634 CrossRef CAS
.
- J. Gao, F. Zhang, H. Xue and L. Zhang,
et al., In-situ synthesis of novel ternary CdS/PdAg/g-C3N4 hybrid photocatalyst with significantly enhanced hydrogen production activity and catalytic mechanism exploration, Appl. Catal., B, 2021, 281, 119509 CrossRef CAS
.
- L. Li and J. Xu, Rare earth perovskite modified cobalt disulfide catalysts controlled by reaction solvent synthesis to form a p-n heterojunction, Appl. Surf. Sci., 2019, 505, 143937 CrossRef
.
- S. Dhingra, T. Chhabra and V. Krishnan,
et al., Visible-Light-Driven Selective Oxidation of Biomass-Derived HMF to DFF Coupled with H2 Generation by Noble Metal-Free Zn0.5Cd0.5S/MnO2 Heterostructures, ACS Appl. Energy Mater., 2020, 3, 7138–7148 CrossRef CAS
.
- T. Liu, Q. Li, S. Qiu and Q. Wang,
et al., Construction of Zn0.5Cd0.5S nanosheets and the hybridization with onion-like carbon for enhanced photocatalytic hydrogen production, Appl. Surf. Sci., 2020, 525, 146586 CrossRef CAS
.
- C. Zeng, A. Hu and A. Zhang,
et al., A core-satellite structured Z-scheme catalyst Cd0.5Zn0.5S/BiVO4 for highly efficient and stable photocatalytic water splitting, J. Mater. Chem. A, 2018, 6(35), 16932–16942 RSC
.
- H. She, Y. Sun and S. Li,
et al., Synthesis of non-noble metal nickel doped sulfide solid solution for improved photocatalytic performance, Appl. Catal., B, 2019, 245, 439–447 CrossRef CAS
.
- B. Zwa, B. Mxa and B. Jla,
et al., Simultaneous visible-light-induced hydrogen production enhancement and antibiotic wastewater degradation using MoS2@ZnxCd1-xS: Solid - solution - assisted photocatalysis, Chin. J. Catal., 2020, 41(1), 103–113 CrossRef
.
- D. Zeng, W. Xu and W. Ong,
et al., Toward noble-metal-free visible-light-driven
photocatalytic hydrogen evolution: monodisperse sub-15 nm Ni2P nanoparticles anchored on porous g-C3N4 nanosheets to engineer 0D-2D heterojunction interfaces, Appl. Catal., B, 2018, 221, 47–55 CrossRef CAS
.
- S. Meng, P. An, L. Chen, S. Sun, Z. Xie, M. Chen and D. Jiang, Integrating Ru-modulated CoP nanosheets binary co-catalyst with 2D g-C3N4 nanosheets for enhanced photocatalytic hydrogen evolution activity, J. Colloid Interface Sci., 2021, 585, 108–117 CrossRef CAS PubMed
.
- C. Ksa, B. Js and C. Yy,
et al., Chunsheng Lei. Highly efficient photocatalytic hydrogen evolution from 0D/2D heterojunction of FeP nanoparticles/CdS nanosheets, Appl. Surf. Sci., 2020, 505, 144042 CrossRef
.
- Z. Sun, H. Zheng, J. Li and P. Du, Extraordinarily efficient photocatalytic hydrogen evolution in water using semiconductor nanorods integrated with crystalline Ni2P cocatalysts, Energy Environ. Sci., 2015, 8(9), 2668–2676 RSC
.
- R. Wu, B. Xiao and Q. Gao,
et al., A Janus nickel cobalt phosphide catalyst for high - efficiency neutral - pH water splitting, Angew. Chem., 2018, 130(47), 15833 CrossRef
.
- S. Yin, J. Han and Z. Yinjun,
et al., A highly efficient noble metal free photocatalytic hydrogen evolution system containing MoP and CdS quantum dots, Nanoscale, 2016, 8(30), 14438–14447 RSC
.
- L. Xie, Q. Zong, Q. Zhang, J. Sun, Z. Zhou, B. He, Z. Zhu and S. Yao, Hierarchical NiCoP nanosheet arrays with enhanced electrochemical properties for high - performance wearable hybrid capacitors, J. Alloys Compd., 2019, 781, 783–789 CrossRef CAS
.
- C. Jin, C. Xu, W. Chang and X. Ma,
et al., Bimetallic phosphide NiCoP anchored g-C3N4 nanosheets for efficient photocatalytic H2 evolution, J. Alloys Compd., 2019, 803, 205–215 CrossRef CAS
.
- Q. Huang, Y. Xiong, Q. Zhang, H. Yao and Z. Li, Noble metal-free MoS2 modified Mn0.25Cd0.75S for highly efficient visible-light driven photocatalytic H2 evolution, Appl. Catal., B, 2017, 209, 514–522 CrossRef CAS
.
- S. Zhao, J. Xu and M. Mao,
et al., NiCo2S4@Zn0.5Cd0.5S with direct Z-scheme heterojunction constructed by band structure adjustment of ZnxCd1-xS for efficient photocatalytic H2 evolution ScienceDirect, Appl. Surf. Sci., 2020, 528, 147016 CrossRef CAS
.
- X. Gao, J. Yang and D. Zeng,
et al., Two-dimensional nickel nanosheets coupled with Zn0.5Cd0.5S nanocrystals for highly improved visible-light photocatalytic H-2 production, J. Alloys Compd., 2021, 871, 159460 CrossRef CAS
.
- Y. Li, D. Zhang and X. Xi,
et al., Noble metal-free bimetallic NiCo decorated Zn0.5Cd0.5S solid solution for enhanced photocatalytic H2 evolution under visible light, Int. J. Hydrogen Energy, 2020, 45(15), 8300–8309 CrossRef CAS
.
- G. M. Bremmer, L. Haandel, E. Hensen and J. Frenken,
et al., The effffect of oxidation and resulfifidation on (Ni/Co)MoS2 hydrodesulfurisation catalysts, Appl. Catal., B, 2019, 243, 145–150 CrossRef CAS
.
- Z. Sun, M. Zhu and M. Fujitsuka,
et al., Phase Effect of NixPy Hybridized with g-C3N4 for Photocatalytic Hydrogen Generation, ACS Appl. Mater. Interfaces, 2017, 9(36), 30583–30590 CrossRef CAS PubMed
.
- R. Shen, W. Liu, D. Ren, J. Xie and X. Li, Co1.4Ni0.6P cocatalysts modifified metallic carbon black/g-C3N4 nanosheet Schottky heterojunctions for active and durable photocatalytic H2 production, Appl. Surf. Sci., 2019, 466, 393–400 CrossRef CAS
.
- S. Li, L. Wang, S. Liu, B. Xu and N. Xiao,
et al., In situ synthesis of strongly coupled Co2P-CdS nanohybrids: an effective strategy to regulate photocatalytic hydrogen evolution activity, ACS Sustainable Chem. Eng., 2018, 6(8), 9940–9950 CrossRef CAS
.
- L. Zhang and Z. Jin, Theoretically guiding the construction of a novel Cu2O@Cu97P3@Cu3P heterojunction with a 3D hierarchical structure for efficient photocatalytic hydrogen evolution, Nanoscale, 2021, 13(2), 1340–1353 RSC
.
- Y. Zhao, Y. Lu, L. Chen and X. Wei, Redox Dual-Cocatalyst-Modified CdS Double-Heterojunction Photocatalysts for Efficient Hydrogen Production, ACS Appl. Mater. Interfaces, 2020, 12(41), 46073–46083 CrossRef CAS PubMed
.
- S. Zhao, J. Xu and M. Mao,
et al., Protonated g-C3N4 cooperated with Co-MOF doped with Sm to construct 2D/2D heterojunction for integrated dye-sensitized photocatalytic H2 evolution, J. Colloid Interface Sci., 2021, 583, 435–447 CrossRef CAS PubMed
.
- A. Kumar, A. Kumar and V. Krishnan, Perovskite Oxide Based Materials for Energy and Environment-Oriented Photocatalysis, ACS Catal., 2020, 10, 10253–10315 CrossRef CAS
.
- A. Kumar, V. Rao and A. Kumar,
et al., Three-Dimensional Carbonaceous Aerogels Embedded with Rh-SrTiO3 for Enhanced Hydrogen Evolution Triggered by Efficient Charge Transfer and Light Absorption, ACS Appl. Energy Mater., 2020, 3, 12134–12147 CrossRef CAS
.
- A. Kumar and V. Krishnan, Vacancy Engineering in Semiconductor Photocatalysts: Implications in Hydrogen Evolution and Nitrogen Fixation Applications, Adv. Funct. Mater., 2021, 31, 2009807 CrossRef CAS
.
- C. Lzab, C. Xhab and C. Jlab,
et al., Unique synergistic effects of ZIF-9(Co)-derived cobalt phosphide and CeVO4 heterojunction for efficient hydrogen evolution, Chin. J. Catal., 2020, 41(1), 82–94 CrossRef
.
- X. Hao, J. Zhou and Z. Cui,
et al., Zn-vacancy mediated electron-hole separation in ZnS/g-C3N4 heterojunction for efficient visible-light photocatalytic hydrogen production, Appl. Catal., B, 2018, 229, 41–51 CrossRef CAS
.
- L. Tian, F. Wang, Z. Zhang and S. Min, Vanadium diboride as an efficient cocatalyst coupled with CdS for enhanced visible light photocatalytic H2 evolution, Int. J. Hydrogen Energy, 2020, 45(38), 19017–19026 CrossRef CAS
.
- M. Ahmad, X. Quan, S. Chen, H. Yu and Z. Zeng, Operating redox couple transport mechanism for enhancing photocatalytic H2 generation of Pt and CrOx-decorated ZnCdS nanocrystals, Appl. Catal., B, 2021, 283, 119601 CrossRef CAS
.
- Z. Jin and L. Zhang, Performance of Ni-Cu bimetallic co-catalyst g-C3N4 nanosheetsfor improving hydrogen evolution, J. Mater. Sci. Technol., 2020, 49, 144–156 CrossRef
.
- L. Zhang, X. Hao, Q. Jian and Z. Jin, Ferrous oxalate dehydrate over CdS as Z-scheme photocatalytic hydrogen evolution, J. Solid State Chem., 2019, 274, 286–294 CrossRef CAS
.
- R. Chava, N. Son and M. Kang, Surface engineering of CdS with ternary Bi/Bi2MoO6-MoS2 heterojunctions for enhanced photoexcited charge separation in solar-driven hydrogen evolution reaction, Appl. Surf. Sci., 2021, 565, 150601 CrossRef CAS
.
- L. Zhang, G. Wang and X. Hao,
et al., MOFs-derived Cu3P@CoP p-n heterojunction for enhanced photocatalytic hydrogen evolution, Chem. Eng. J., 2020, 395(4), 125113 CrossRef CAS
.
- Z. Jin, Y. Liu and X. Hao, Self-assembly of zinc cadmium sulfide nanorods into nanoflowers with enhanced photocatalytic hydrogen production activity, J. Colloid Interface Sci., 2020, 567, 357–368 CrossRef CAS PubMed
.
- Z. Luo, X. Zhao, H. Zhang and Y. Jiang, Zn0.3Cd0.7S nanorods loaded with noble-metal-free Ni3C co-catalyst enhancing photocatalytichydrogen evolution, Appl. Catal., A, 2019, 582, 117115 CrossRef CAS
.
- X. Li, R. He, Y. Dai and S. Li,
et al., Design and fabrication of Co9S8/Zn0.5Cd0.5S hollow nanocages with significantly enhanced photocatalytic hydrogen production activity, Chem. Eng. J., 2020, 400, 125474 CrossRef CAS
.
- D. Gao, X. Wu and P. Wang,
et al., Selenium-enriched amorphous NiSe1+ nanoclusters as a highly efficient cocatalyst for photocatalytic H2 evolution, Chem. Eng. J., 2020, 408(4), 127230 Search PubMed
.
- M. Xiong, Y. Qin and B. Chai,
et al., Unveiling the role of Mn-Cd-S solid solution and MnS in MnxCd1-xS photocatalysts and decorating with CoP nanoplates for enhanced photocatalytic H2 evolution, Chem. Eng. J., 2021, 428, 131069 CrossRef
.
- R. Shen, W. Liu and D. Ren,
et al., Co1.4Ni0.6P cocatalysts modified metallic carbon black/g-C3N4 nanosheet Schottky heterojunctions for active and durable photocatalytic H2 production, Appl. Surf. Sci., 2019, 466(FEB.1), 393–400 CrossRef CAS
.
- L. Zhou, Y. Kong and Y. Du,
et al., Spatial and electronic structure of the Ni3P surface, Appl. Surf. Sci., 2010, 256(24), 7692–7695 CrossRef CAS
.
- D. Ren, Z. Liang, Y. Ng, P. Zhang, Q. Xiang and X. Li, Strongly coupled 2D-2D nanojunctions between P-doped Ni2S (Ni2SP) cocatalysts and CdS nanosheets for efficient photocatalytic H2 evolution, Chem. Eng. J., 2020, 390, 124496 CrossRef CAS
.
Footnote |
† Electronic supplementary information (ESI) available. See DOI: 10.1039/d1nj04251c |
|
This journal is © The Royal Society of Chemistry and the Centre National de la Recherche Scientifique 2022 |
Click here to see how this site uses Cookies. View our privacy policy here.