DOI:
10.1039/D1NJ03424C
(Paper)
New J. Chem., 2022,
46, 185-198
New ferrocene-integrated multifunctional guanidine surfactants: synthesis, spectroscopic elucidation, DNA interaction studies, and DFT calculations†
Received
15th July 2021
, Accepted 8th November 2021
First published on 17th November 2021
Abstract
Three new ferrocene-substituted aliphatic guanidines were successfully synthesized and well characterized by means of several analytical methods such as FT-IR, 1H and 13C-NMR, Raman, atomic absorption spectroscopy (AAS), and elemental analysis. The amphiphilic nature of ferrocene-based guanidines has been evaluated by determining their critical micelle concentration (CMC) through tensiometry, and UV-visible spectroscopy. The results obtained through both the techniques are in good agreement with each other. It was observed that the alkyl chain length played a major part in determining the CMC in pure ethanol as well as in an ethanol/water mixture. The quantum mechanical parameters such as the energy of frontier molecular orbitals (EHOMO and ELUMO) and the Mulliken charge distribution on the optimized structures was determined using a DFT/B3LYP method combined with the 6-31G(d,p) basis set in the gas phase. The shift in the peak potential, current and absorption maxima of the studied ferrocenyl guanidines in the presence of DNA revealed that cyclic voltammetry coupled with UV-visible spectroscopy provided a way to elaborate the DNA interaction mechanism, a pre-requisite for the design of new anticancer agents and understanding of the molecular basis of their action. The synthesized ferrocenyl derivatives exhibited good scavenging activity against the 1,1-diphenyl-2-picrylhydrazyl radical (DPPH). These complexes were also scanned for their in vitro cytotoxicity against the human carcinoma cell line (MCF-7) and a normal cell line (MCF-10A) by means of an MTT assay, thereby rendering G-18 as the most potent chemotherapeutic drug.
1. Introduction
Guanidines have earned a huge reputation due to their biological and synthetic applications, high basicity and remarkable thermodynamic stability.1 They are categorized as organic compounds exhibiting three nitrogen atoms that are covalently bonded to the central carbon atom.2–4 The electron donation ability of these nitrogen atoms endowed an unusual basic character to the guanidine molecules. The introduction of electron withdrawing/electron donating or conjugated functionality into the molecular structure of guanidine makes it possible to broaden its biological profile by adjusting its basicity, lipophilicity, and vice versa. A number of guanidine-based compounds have been known for their pharmacodynamic applications e.g. they play an inhibitory role against urokinase, which is responsible for a large number of malignancies including lung, stomach, breast, bladder, kidney, and brain cancers.5–7 Due to the rich supramolecular chemistry of guanidine molecules, they exhibit antimicrobial,8 anti-cancer,9–13 anti-inflammatory, anti-diabetic and cytotoxic properties.12 Guanidine and its derivatives are also used as cardiovascular as well as anti-hypertensive drugs.14,15
The incorporation of a ferrocenyl fragment into an organic framework frequently augments the biological action of the resulting compound, just like the ferrocene derivatives of quinine, mefloquine and chloroquine have shown enhanced antimalarial activities.16,17 It is apparent that the presence of a ferrocene moiety can reduce the toxicity of drugs and can also increase its antioxidant candidature.18 Moreover, the introduction of an aliphatic chain into the guanidine functionality not only improved the lipophilicity, but also endowed the surface activity, and self-ordering properties in organic and aqueous-organic media. The self-ordering properties of a surfactant in a mixture of organic/aqueous media play a prime role in template synthesis of inorganic materials and micelles help to determine the pore size distribution and pore ordering of nonporous materials.17,19 Ethanol is a commonly used additive in such template synthesis due to the lower solubility of many organic reactants in pure aqueous media. A number of reports on the mechanism of aggregation in organic/aqueous mixtures have been presented.19–23
Keeping in consideration the above-mentioned points, it was thought worthwhile to couple the pharmacokinetic properties of ferrocenyl guanidines with the self-assembling nature of amphiphiles. For this purpose, ferrocenyl guanidinium compounds with variable chain lengths were synthesized and structurally investigated by various spectroscopic techniques. Critical micelle concentration was determined in different media by tensiometry and UV-visible spectroscopy to have a comparative outlook of self-aggregation and surface activity of newly prepared guanidine appended amphiphiles. In order to consider the therapeutic properties of ferrocene-functionalized guanidines, in vitro antioxidant, DNA interaction, and cytotoxic studies were accomplished.
2. Experimental
2.1 Materials and methods
The reagents used during this study including: sodium nitrite, ferrocene, 2-methyl-4-nitroaniline, hydrochloric acid, hydrazine, hexadecyltrimethylammonium bromide (PTC), myristoyl chloride, potassium thiocyanate, heptyl amine, hexadecyl amine, and octadecyl amine were obtained from Fluka, Sigma Aldrich, and Merck. Solvents such as: acetone, chloroform, ethanol, methanol, petroleum ether, diethyl ether, dimethylformamide, cyclohexane, and n-hexane were dried and distilled before use in accordance with the standard drying protocols. Melting points were determined by using Gallem Kamp (UK) electrothermal melting point apparatus. 1H and 13C-NMR spectra for all the synthesized compounds were recorded using an advanced NMR BRUKER spectrophotometer using tetramethylsilane as an internal reference. FT-IR spectral studies of the compounds were recorded using a BRUKER (Tensor-37) spectrophotometer (4000–400 cm−1). Raman spectra (±1 cm−1) were measured on an InVia Renishaw spectrometer, using argon-ion (514.5 nm) and near-infrared diode (785 nm) lasers. Renishaw WiRE 2.0 software was used for the Raman data acquisition and spectral manipulation. The elemental analyses were performed using a LECO-932 CHNS analyzer, while the Fe concentrations were determined on an atomic absorption spectrophotometer (AAS) PerkinElmer 2380. Mass spectra (ESI-MS) were recorded using LCQ Duo and double focusing MS25RFA instruments. For the assessment of critical micelle concentration an automatic surface tensiometer (BZY-202) was used. A SHIMADZU (UV-1800) spectrophotometer was also used for the CMC determination and DNA binding activities. Computational measurements (DFT) for all the synthesized compounds were performed utilizing Gaussian 9.0 software. Cyclic voltammetric analysis for DNA-binding studies was carried out by using Gamry Potentiostat/Galvanostat Interface 1000 (Version 6.03).
2.2 General procedure for the synthesis of ferrocene-based guanidines
The syntheses of ferrocenyl aliphatic guanidines (G-7, G-16, and G-18) were attained in four steps (Scheme 1). The first step involved the preparation of nitrophenylferrocene by the coupling of ferrocene with diazonium salt of nitroaniline using a phase transfer catalyst. In the second step, this nitrophenyl ferrocene was reduced to ferrocenylaniline using palladium-charcoal as the catalyst and hydrazine served as the reducing agent.24 In the subsequent reaction, thiourea (13C4N) was synthesized by the coupling of substituted aniline with thiocyanate in dry acetone.25 The last step involved the synthesis of ferrocene-based guanidine as a result of the mixing of freshly prepared ferrocenyl thioureas and different amines in dimethyl formamide in an equimolar ratio with two equivalents of triethylamine.26 The temperature was maintained below 5 °C using an ice-salt bath and one equivalent of mercuric chloride (HgCl2) was added to the reaction mixture under vigorous stirring. The ice bath was removed after 45 min while the stirring continued overnight. The progress of the reaction was monitored by thin layer chromatography (TLC). The solvent from the filtrate was evaporated under reduced pressure and the residue was re-dissolved in 30 ml dichloromethane, washed with water and the resulting product was dried.27,28
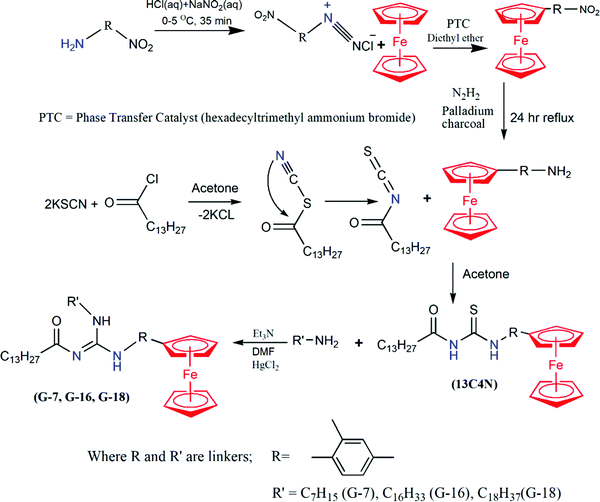 |
| Scheme 1 Synthetic scheme for ferrocene-based guanidine surfactants. | |
2.2.1 Synthesis of N-(4-ferrocenyl-3-methylphenyl)-N′-(octadecyl)-N′′-tetradecanamideguanidine (G-18).
The quantities used were 2.8 mL (20 mmol) triethylamine, 0.070 g (0.259 mmol) octadecylamine, 0.15 g (0.267 mmol) of 1-(tetradecanamide)-3-(4-ferrocenyl-3-methylphenyl) thiourea and 0.073 g (0.268 mmol) mercuric chloride. Yield: 83%; dark brown solid; m.p. 210 °C; FT-IR and Raman (powder, cm−1): 3307, 3348 (N–H), 3091, 3023 (sp2 C–H), 2920, 2886 (sp3 C–H), 1659, 1645 (C
O), 1582, 1577 (C
N), 486, 478 (Fe-Cp); 1H-NMR (300 MHz, acetone-d6, ppm): δ 10.67 (s, 1H, NH), 9.55 (s, 1H, NH), 7.71 (d, 1H, J = 8.7 Hz, Ar-H), 7.44 (dd, 1H, 1J = 8.7 Hz, 2J = 2.1 Hz, Ar-H), 7.33 (d, 1H, J = 2.1 Hz, Ar-H), 4.53 (t, 2H, J = 1.8 Hz, o-C5H4), 4.32 (t, 2H, J = 1.8 Hz, m-C5H4), 4.14 (s, 5H, C5H5), 2.55–2.50 (m, 2H, -CH2-R), 2.39 (s, 3H, Ar-CH3), 1.76–1.27 (m, 56H, R-H), 0.91–0.87 (m, 6H, R-CH3); 13C-NMR (75 MHz, Acetone-d6, ppm): δ 170.8 (s, C
O), 151.6 (s, CN3), 137.6 (s, Ar-C), 135.6 (s, Ar-C), 132.1 (s, Ar-C), 130.6 (s, Ar-C), 120.7 (s, Ar-C), 116.5 (s, Ar-C), 87.2 (s, ipso-C5H4), 69.6 (s, C5H5), 69.2 (s, o-C5H4), 67.7 (s, m-C5H4), 40.6 (s, aliphatic-C), 36.8 (s, aliphatic-C), 31.8 (s, aliphatic-C), 30.3 (s, aliphatic-C), 26.7 (s, aliphatic-C), 25.7 (s, aliphatic-C), 25.4 (s, aliphatic-C), 22.5 (s, aliphatic-C), 20.8 (s, aliphatic-C); ESI-MS (m/z): 796.51; elemental analysis calcd (%) for C50H81FeN3O: C, 75.44; H, 10.26; N, 5.28; Fe, 7.02. Found (%): C, 75.42; H, 10.29; N, 5.32; Fe, 7.06.
2.2.2 Synthesis of N-(4-ferrocenyl-3-methylphenyl)-N′-(hexadecyl)-N′′-tetradecanamideguanidine (G-16).
The quantities used were 2.8 mL (20 mmol) triethylamine, 0.065 g (0.269 mmol) hexadecylamine, 0.15 g (0.267 mmol) of 1-(tetradecanamide)-3-(4-ferrocenyl-3-methylphenyl) thiourea, and 0.073 g (0.268 mmol) mercuric chloride. Yield: 87%; orange-red solid; m.p. 204–205 °C; FT-IR and Raman (powder, cm−1): 3291, 3302 (N–H), 3094, 3011 (sp2 C–H), 2927, 2951 (sp3 C–H), 1654, 1665 (C
O), 1579, 1581 (C
N), 483, 470 (Fe-Cp); 1H-NMR (300 MHz, acetone-d6, ppm): δ 10.18 (s, 1H, NH), 9.05 (s, 1H, NH), 7.65 (d, 1H, J = 8.5 Hz, Ar-H), 7.57 (dd, 1H, J = 8.1 Hz, J = 1.5 Hz, Ar-H), 7.46 (d, 1H, J = 1.5 Hz, Ar-H), 4.52 (t, 2H, J = 1.6 Hz, o-C5H4), 4.33 (t, 2H, J = 1.7 Hz, m-C5H4), 4.14 (s, 5H, C5H5), 2.33–2.06 (m, 2H, -CH2-R), 2.18 (s, 3H, Ar-CH3), 1.63–1.30 (m, 52H, R-H), 0.91–0.87 (m, 6H, R-CH3); 13C-NMR (75 MHz, Acetone-d6, ppm): δ 171.6 (s, C
O), 150.4 (s, CN3), 135.8 (s, Ar-C), 134.3 (s, Ar-C), 132.7 (s, Ar-C), 129.4 (s, Ar-C), 120.5 (s, Ar-C), 117.2 (s, Ar-C), 87.6 (s, ipso-C5H4), 70.4 (s, C5H5), 69.7 (s, o-C5H4), 66.1 (s, m-C5H4), 41.3 (s, aliphatic-C), 37.9 (s, aliphatic-C), 31.6 (s, aliphatic-C), 30.7 (s, aliphatic-C), 27.2 (s, aliphatic-C), 24.9 (s, aliphatic-C), 24.0 (s, aliphatic-C), 21.8 (s, aliphatic-C), 20.5 (s, aliphatic-C); ESI-MS (m/z): 768.84; elemental analysis calcd (%) for C48H77FeN3O: C, 75.07; H, 10.11; N, 5.47; Fe, 7.27. Found (%): C, 74.08; H, 10.19; N, 5.52; Fe, 7.31.
2.2.3 Synthesis of N-(4-ferrocenyl-3-methylphenyl)-N′-(heptyl)-N′′-tetradecanamideguanidine (G-7).
The quantities used were 2.8 mL (20 mmol) triethylamine, 2.7 g (10 mmol) heptyl amine, 0.15 g (0.267 mmol) of 1-(tetradecanamide)-3-(4-ferrocenyl-3-methylphenyl) thiourea and 0.073 g (0.268 mmol) mercuric chloride. Yield: 75%; dark brown solid; m.p. 142–143 °C; FT-IR and Raman (powder, cm−1): 3305, 3246 (N–H), 3093, 3081 (sp2 C–H), 2939, 2912 (sp3 C–H), 1658, 1650 (C
O), 1578, 1575 (C
N), 482, 485 (Fe-Cp); 1H-NMR (300 MHz, Acetone-d6, ppm): δ 9.03 (s, 1H, NH), 7.97 (s, 1H, NH), 7.66 (d, 1H, J = 8.5 Hz, Ar-H), 7.51 (dd, 1H, 1J = 8.5 Hz, 2J = 1.3 Hz, Ar-H), 7.45 (d, 1H, J = 1.5 Hz, Ar-H), 4.53 (t, 2H, J = 1.6 Hz, o-C5H4), 4.32 (t, 2H, J = 1.9 Hz, m-C5H4), 4.14 (s, 5H, C5H5), 2.29–2.05 (m, 2H, -CH2-R), 2.37 (s, 3H, Ar-CH3), 1.63–1.30 (m, 34H, R-H), 0.91–0.87 (m, 6H, R-CH3); 13C-NMR (75 MHz, acetone-d6, ppm): δ 170.1 (s, C
O), 152.3 (s, CN3), 136.9 (s, Ar-C), 134.6 (s, Ar-C), 131.5 (s, Ar-C), 130.8 (s, Ar-C), 119.1 (s, Ar-C), 115.7 (s, Ar-C), 86.3 (s, ipso-C5H4), 70.8 (s, C5H5), 69.5 (s, o-C5H4), 66.4 (s, m-C5H4), 39.7 (s, aliphatic-C), 35.8 (s, aliphatic-C), 32.6 (s, aliphatic-C), 31.2 (s, aliphatic-C), 26.4 (s, aliphatic-C), 25.9 (s, aliphatic-C), 24.4 (s, aliphatic-C), 22.9 (s, aliphatic-C), 21.6 (s, aliphatic-C); ESI-MS (m/z): 649.24; elemental analysis calcd (%) for C39H59FeN3O: C, 72.99; H, 9.27; N, 6.55; Fe, 8.70. Found (%): C, 73.03; H, 9.29; N, 6.58; Fe, 8.78.
2.3 Critical micelle concentration (CMC) determination
2.3.1 Surface tension measurements.
Surface tensiometry was employed to determine the aggregation point of the synthesized compounds. Measurement of surface tension was carried out by following the De-Nouy ring method. Prior to the measurement, a platinum ring was washed with distilled water and dried. The surface tension of the solvent systems, ethanol and ethanol–water mixture (8
:
2), was measured as 22 mN m−1 and 47.29 mN m−1, respectively at 20 °C. Then, the solution of tested surfactant was added to the glass tray by successive increase in concentration of 0.001 mM and surface tension was observed through readings that appeared on the tensiometer screen. A graph between the surface tension (mN m−1) and log of concentrations (mM) was plotted in order to achieve the CMC value.
2.3.2 UV-visible spectroscopic measurements.
The critical micelle concentration (CMC) was determined through UV-visible spectroscopy. The stock solutions (1 mM) of all the synthesized surfactants (G-7, G-16, G-18) were prepared in ethanol and an ethanol–water mixture (8
:
2). The baseline was set each time by taking the solvent in both the reference cell and the working cell to avoid the solvent effects. Then, a small aliquot of surfactant solution (1 mM) was added continuously to the solvent, and the absorbance spectrum was recorded for varying concentrations of tested surfactant. A graph of absorbance vs. concentration (mM) was plotted, and an inflection point was located and labelled as the CMC point.29
2.4 DNA interaction studies
2.4.1 UV-visible spectroscopy.
Absorption spectra were recorded on a UV-visible spectrophotometer. Firstly, the stock solutions of ferrocene-based guanidines (25 μM), in ethanol and that of DNA (6 × 10−4 M) in distilled water were prepared. The concentration of the stock solution of DNA was calculated by using the molar extinction co-efficient (ε = 600 M−1 cm−1) giving UV-absorbance at 260 nm,30 which renders this DNA solution as protein free due to the A260/A280 ratio between 1.80–1.90. For spectroscopic measurements, absorbance of the 25 μM compound was recorded without DNA. The response was then examined for the same amount of compound on the addition of small aliquots of different concentrations of DNA (3–18 μM) at constant intervals of 3–4 min, on the basis of variation in the absorption maxima (λmax). The binding constant “K” of the compound–DNA was calculated by using the Benesi–Hildebrand equation.31 |  | (1) |
where A and Ao corresponds to the absorbance of the free compound and the compound–DNA complex, and εG and εH–G are the molar extinction coefficient of the free compound and the extinction coefficient of the compound–DNA respectively.
2.4.2 Cyclic voltammetry.
In order to electrochemically evaluate the drug–DNA binding studies, stock solutions of all synthesized compounds (1 mM) were prepared in DMSO, and that of DNA in distilled water. KCl solution was used as an electrolyte. Measurements were carried out by using a three electrode assembly in which glassy carbon was used as the working electrode, Ag/AgCl was taken as the reference electrode, while the platinum wire served as the counter electrode. Before taking each measurement, the glassy carbon electrode was polished with alumina powder and rinsed with distilled water. The experiment was performed with 1 mM free compound (without DNA) at different scan rates (100 mV s−1 to 700 mV s−1) and the respective voltammograms were recorded. In the next step, the experiment was repeated with the addition of 10 μL DNA at varying scan rates from 100 mV s−1 to 700 mV s−1. The same procedure was repeated by keeping the concentration of compound and the scan rate constant (100 mV s−1), while varying the DNA concentrations from 0.002–0.016 mM at constant intervals of 2–3 minutes. Variations in peak current and potential indicate the appreciable binding of DNA with the compound. On account of the decrease in peak current, the binding constant (K) was calculated by using this eqn (2).32 | 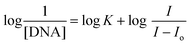 | (2) |
The Randles–Sevcik equation was used for calculating the diffusion coefficients.24 |  | (3) |
where Ipa is the anodic peak current, n is the number of electrons involved in the process, A is the geometric area of the electrode in cm2, ν is the scan rate in V s−1, α is the transfer coefficient,
is the concentration of the reductant in mol L−1, and Do is the diffusion coefficient in cm2 s−1.
2.5 Computational studies
Computational studies were executed in order to optimize the given structures, to calculate the different electrochemical parameters i.e., Mulliken charge distribution on the molecular structures, the energies of the frontier molecular orbitals (EHOMO and ELUMO)33 ionization energy (−EHOMO), electron affinity (−ELUMO), band gap, electronegativity (χ), global hardness (η), softness (σ), and dipole moment for all the tested compounds by using the 6-31G(d,p)DFT/B3LYP method. Rather than using complex and lengthy calculations, DFT (Density Functional Method) was preferred because of its simplicity and less time consumption. Gaussian 9.0 software was used for measurements.
2.6 DPPH free radical scavenging assay
The reducing ability of synthesized ferrocenyl derivatives was determined with the help of 1,1-diphenyl-2-picrylhydrazyl in DMSO to produce 1,1-diphenyl-2-picrylhydrazine. The decrease in the absorption of 1,1-diphenyl-2-picrylhydrazyl (DPPH) was monitored to calculate the percentage of scavenging according to the following formula:34,35
Scavenging activity (%) = Ao − A/Ao × 100 |
where Ao is the absorbance of free DPPH and A is the absorption of the DPPH–drug mixture with increasing concentration of drug. Increasing concentrations (20 μM) of the synthesized compounds (G-18, G-16, and G-7) were added to a solution of DPPH (3.9 mg of DPPH in 100 mL DMSO). The decrease in absorption of DPPH was monitored after 30 min at a wavelength of 517 nm. All the readings were taken in triplicate and averages of all the readings were used.
2.7 Cytotoxicity assay
The growth inhibitory effect of the synthesized complexes on the human breast cancer cell line MCF-7 (ATCC# HTB-22), and breast non-cancerous cells MCF-10A (ATCC# CRL-10317) was assessed by use of the MTT (3-(4,5-dimethylthiazol-2-yl)-2,5-diphenyl tetrazolium bromide) assay, in which the metabolically active cells reduce MTT to yield a DMSO soluble formazan product that can be analyzed calorimetrically.36,37 The cells were maintained and cultured in RPMI 1640 culture medium supplemented with 10% FBS (fetal bovine serum). Cisplatin was used as a control. In brief, 1 × 104 cells were seeded in triplicate into the wells of flat bottomed 96-well culture plates. The plates were incubated at 37 °C for 24 h in a humidified atmosphere (5% carbon dioxide in air, pH 7.4) to permit the cells to attach. The samples were then added at different concentrations (0–100 μM in ethanol) to triplicate wells. After 72 h of incubation, the cells were exposed to 20 μL of MTT (5 mg mL−1) and incubated for 4 h at 37 °C for further cultivation. The yellow formazan crystals thus produced in each well were solubilized in 200 μL of DMSO and the absorbance of the resulting solution was read at 570 nm using a microplate reader (Labsystems Multiskan MS). The sensitivity of the compounds (G-7, G-16, and G-18) against the subjected cell lines was expressed in terms of IC50 values (drug concentration that resulted in 50% reduction of cell growth), calculated on the regression line where the absorbance values at 570 nm were plotted against the logarithm of drug concentration. The experiment was performed in triplicate.
3. Results and discussion
3.1 Spectroscopic analysis
3.1.1 FT-IR and Raman spectroscopy.
FT-IR and Raman spectroscopic analyses were carried out in order to measure the vibrational energy modes of the synthesized ferrocenyl derivatives. The results of both the characterization techniques were found to be in close agreement with each other.
In the FT-IR and Raman data of the synthesized compounds the characteristic N–H band appeared between 3348–3246 cm−1, sp2 (C–H) at about 3094–3011 cm−1, and sp3 (C–H) at 2951–2886 cm−1 (Fig. S1, ESI†). The carbonyl peak in the case of thiourea appeared at 1692 cm−1, while the carbonyl stretching frequency for the guanidines was observed in the range of 1665–1645 cm−1. This indicates that the carbonyl band shifts towards lower frequency due to extended conjugation in the guanidine derivatives.38 The intensity of the carbonyl peak appears to be low due to the presence of hydrogen bonding between the oxygen of the carbonyl and hydrogen of the CN3 unit. The C
N stretching frequency was observed in the range of 1582–1575 cm−1 which is intermediate between single and double bond vibrational frequencies, attributing the conjugation between the all three nitrogen atoms of the guanidine moiety. The characteristic peak for long chain methylene (–CH2–) lies in the range of 720–715 cm−1, while the Fe–Cp peak was visible in the range of 486–470 cm−1.
3.1.2
1H and 13C-NMR spectroscopy.
In the 1H-NMR spectra of ferrocene-based thiourea and guanidine derivatives, the shifting of two –NH signals from 12.7 ppm to 10.6 ppm and 10.2 ppm to 9.5 ppm, indicating that the sulfur has been successfully replaced by an alkyl amine, which is a clear indication of the successful formation of guanidine as depicted in Fig. S2(a) and S3(a) (ESI†). The aromatic protons appeared in the range of 7.7–7.3 ppm and the protons of the aliphatic chain exhibited the chemical shift values in the region of 2.8–0.8 ppm. Terminal CH3 protons of both the aliphatic chains appeared at 0.8 ppm. The protons of the unsubstituted cyclopentadienyl ring of ferrocene displayed a very high intensity singlet at 4.1 ppm, whereas the signal for the four protons of the substituted cyclopentadienyl ring of ferrocene split up into two signals in the region of 4.3 ppm and 4.5 ppm, respectively.
The 13C-NMR data of ferrocene-based guanidines (G-18, G-16, and G-7) showed that the most downfield signals appeared in the range of 171.6–170.1 ppm which are attributed to the most deshielded carbon of the carbonyl group, while the guanidine carbon (CN3) appeared relatively up-field in the range of 152.3–150.4 ppm which indicates the successful formation of guanidines as depicted in Fig. S3(b) (ESI†).39 The unsubstituted carbon of cyclopentadienyl (Cp) ring of ferrocene yielded a peak of high intensity at 69.3 ppm, whereas the substituted (Cp) ring exhibited three peaks. The carbon of the Cp ring attached to the phenyl group (ipso carbon) gave a peak at around 87.2 ppm, the peak at 69.2 ppm is due to the ortho-carbon atoms, whereas the peak at 67.7 ppm is for the meta-carbons of the Cp ring of ferrocene. The disappearance of the peak at 178 ppm due to the thiocarbonyl moiety (C
S) was also noticed which provided strong evidence that the sulfur atom has been removed successfully validating the formation of guanidines. All the aromatic carbon atoms appeared in their respective region around 137.6–116.5 ppm, whereas the carbon atoms of the aliphatic chain appeared in the region of 40.6–20.8 ppm as presented in Fig. S3(b) (ESI†).
3.1.3 Elemental analysis (CHNS) and atomic absorption spectroscopy (AAS).
The results of elemental analysis and atomic absorption spectroscopy (AAS) were observed to be in good correlation with the calculated values which demonstrates the purity of the compounds in the bulk. The carbon, hydrogen and nitrogen percentages were determined with a CHNS analyzer, while the percentage of Fe was measured by AAS.
3.2 CMC measurements
3.2.1 Surface tensiometry.
Surface tensiometry is a standard method to determine the point of self-ordering (CMC). With the increase in the concentration of surfactant (G-7), a continuous decrease in the surface tension (γ) was observed as displayed in Fig. 1. The compounds possessing an alkyl chain length of 8–18 carbon atoms have the tendency to adsorb at the air/solvent interface. The synthesized surfactants being able to adsorb at the interface, dropped the surface tension of ethanol and the ethanol–water mixture up to the level of 9–11 mN m−1. Before attaining the plateau in the plot of γ vs. log concentration, the breaking point is located as CMC. The presence of a plateau is attributed to the formation of surface aggregates before the bulk micellization that causes the re-formation of the surface monolayer at the concentration > CMC.
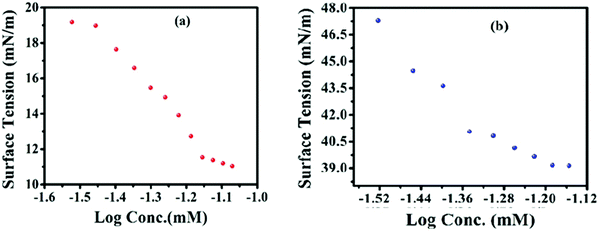 |
| Fig. 1 (a) CMC determination plot of a representative compound G-7 in pure ethanol by a surface tension method. (b) CMC determination plot of G-7 in the (ethanol + water) mixture by the surface tension method. | |
A slight decrease in CMC is observed in the presence of 20% water contents as is clearly depicted in Fig. 1, which can easily be explained on the basis of dielectric constant of the solvent system. The dielectric constant of solvent is directly related to polarity which in turn affects the solubility. The dielectric constant is higher in the case of water than in the case of ethanol, and the presence of two alkyl chains also imparts a more lipophilic character to the synthesized scaffolds. The two factors eventually cause the lowering of solubility and ease of micelles formation in the case of the water/ethanol system as depicted in Fig. 1. Thus, owing to the non-ionic character and very low HLB (∼2), a decline in measured CMC values is observed in the presence of more polar water contents (Table 1). Likewise, it also gives insight that any rise in water percentage from 8
:
2 would lead to a further decrease in solubility in the same order due to a higher solvophobic effect.
Table 1 Comparison of CMC values in different solvent systems
Code |
CMC (ethanol) by a UV-visible absorbance method |
CMC (ethanol + water) by a UV-visible absorbance method |
G-7
|
0.066 |
0.031 |
G-16
|
0.046 |
0.024 |
G-18
|
0.037 |
0.021 |
A progressive CMC diminution was observed when the alkyl chain length of one of the lipophilic tails was increased from 7 to 18 carbons. This indicates that the successive addition of a methylene (–CH2) group in the alkyl chain of the synthesized molecules is an important factor in the diminution of the critical micelles concentration (CMC).32 A comparison of the CMC of G-7, G-16 and G-18 suggested that G-7 has the highest CMC value (0.057 mM), whereas G-18 has the lowest CMC value (0.037 mM), which is attributed to the higher lipophilic character of G-18 as mentioned in Table 1. It is a known fact that lipophilicity facilitates the micelles formation by lowering its solubility in the solvent.
3.2.2 UV-visible spectroscopy.
The UV-visible absorption spectra with varying concentration of G-7 in pure ethanol and an ethanol–water mixture are presented in Fig. 2a and c, respectively. A linear increase in the absorption was observed as a result of the addition of 0.006 mM of surfactant solution, which is analogous to the Beer Lambert law, but after a certain concentration, no significant rise in the absorption intensity was noticed for the same addition of 0.006 mM that is against the Beer Lambert law. Owing to the amphiphilic nature, the synthesized compounds tend to self-assemble at a definite concentration range. These micelles assembles abstacle the passage of UV-visible radiations which results in the deviation from Beer Lambert Law.
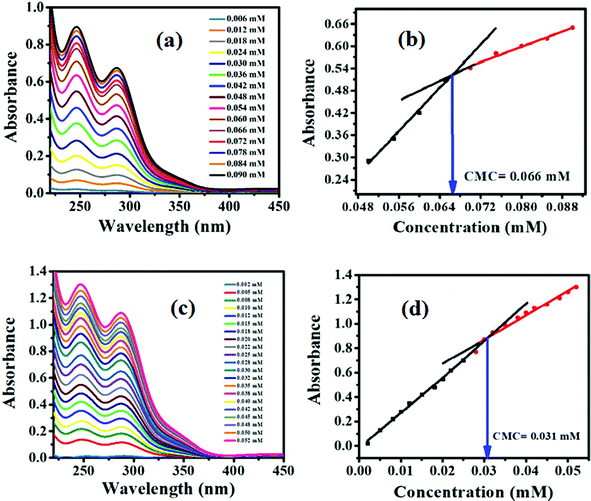 |
| Fig. 2 (a) Representative UV-visible absorbance spectrum of the G-7 compound in ethanol. (b) Absorbance vs. concentration plot of G-7 in ethanol. (a) Representative UV-visible absorbance spectrum of the G-7 compound in an ethanol–water mixture. (b) Absorbance vs. concentration plot of G-7 in an ethanol–water mixture. | |
A graph of absorbance and concentration (mM) gave two straight lines with dissimilar slopes. The breaking point of the two straight lines gave an indication of the CMC point as depicted in Fig. 2b and d.40
A comparison of the CMC results showed that the CMC values obtained through UV-visible spectroscopy and surface tensiometry are comparable, as illustrated in Table 2.
Table 2 Comparison of CMC values by UV-visible absorbance and a surface tension method
Compound codes |
CMC (ethanol) by a UV/Visible absorbance method |
CMC (ethanol) by a surface tension method |
G-7
|
0.066 |
0.057 |
G-16
|
0.046 |
0.040 |
G-18
|
0.037 |
0.037 |
A decrease in CMC values for longer alkyl chains is also clearly visible in the mentioned table.33 The same trend of decrease in the CMC in the ethanol–water mixture is also validated as mentioned in Table 1. G-7 has a smaller chain of seven carbon atoms and shows the highest CMC value, whereas G-18 with the longest alkyl chain retains the lowest CMC value, as calculated through both the methods. Non-ionic surfactants usually have a low CMC value than their ionic counterparts. All the synthesized surfactants are non-ionic in nature with two lipophilic tails and hence, have lower CMC values. The higher lipophilic portion in the structure of the synthesized surfactants with smaller HLB values in the range of 2.11 to 2.61 facilitates the micelles formation at the lower concentration.
3.3 DNA binding studies
DNA binding studies were executed with the aim to understand the binding affinity of the compounds with DNA. For this purpose, DNA binding studies were carried out through UV-visible spectroscopy and cyclic voltammetry (CV).
3.3.1 UV-visible spectroscopy.
The strong interaction of compound 13C4N with DNA was monitored by a change in the absorbance of the compound with substantial addition of DNA. The UV spectrum of the compound 13C4N (thiourea) displayed an intense peak with a shoulder peak in the UV range (255, 300 nm) (Fig. S7, ESI†). The intense peak appearing at 256 nm is attributed to the π–π* transition of electrons associated to the phenyl chromophore along with a shoulder peak at 279 nm due to the n–π* transition of the carbonyl group.27 With the gradual addition of DNA (3–24 μM), a decrease in the absorbance (hypochromism) with a slight red shift was noticed in the UV visible spectrum of the compound (13C4N). The hypochromic effect is thought to be due to the intercalative mode of association of the planar part of the compound with DNA. In close vicinity to DNA, the π* orbital of the binding moiety of compounds could couple with the π-orbital of DNA bases. Thus, the coupling of the π* orbital may result in the partial filling of electrons, thus alleviating the probability of transition which in turn causes hypochromism.27 The UV-visible spectrum of thiourea (13C4N) depicts the decreasing trend of absorbance with the addition of DNA to the compound. A straight-line graph of Ao/A − Aovs. 1/[DNA] is obtained that is used for calculating the binding constant (Kb) by using the Benesi–Hildebrand equation (Fig. S7, ESI†).
The characteristic UV spectrum of the compound G-18 gave two peaks in the UV region (Fig. 3). The peak at 247 nm is due to the π–π* transition of electrons in phenyl chromophore, while the other peak at 288 nm is due to the n–π* transitions in C
N and C
O and conjugation between C
O of carbonyl and C
N of guanidine moiety as well as the extended conjugation among three nitrogen atoms of guanidine. Following the substantial addition of DNA (3–18 μM), the UV-Vis spectrum showed a decrease in absorption with a slight blue shift (hypochromism) attributing to the electrostatic mode of association of G-18 with the DNA. The hypochromic effect is thought to be due to the interaction between the electronic states of the binding chromophore and those of the DNA bases.41 It is likely that the strength of this electronic interaction would decline as the cube of the distance of separation between the chromophore and the DNA bases.42 So, the noticeable hypochromism observed in our experiments proposed the close vicinity of the chromophore of the synthesized complex to the DNA bases. At the closest approach to DNA, the π* orbital of the binding moiety of the compounds could couple with the π orbital of purine or pyrimidine. The coupling π* orbital may get partially filled by electrons, thus decreasing the transition probabilities, and hence result in hypochromism.43
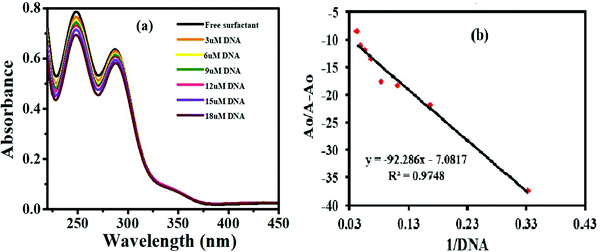 |
| Fig. 3 (a) Representative plots of absorbance vs. wavelength of 25 μM G-18 in ethanol with increasing concentration of DNA (3–18 μM). (b) Representative plot of Ao/A − Aovs. 1/[DNA] for the determination of the DNA binding constant of G-18. | |
3.3.2 Cyclic voltammetry.
The results of DNA interaction studies obtained through UV-visible spectroscopy were equally supported by cyclic voltammetry. Fig. 4 illustrates the DNA binding study of a representative ferrocenyl guanidine G-18 using cyclic voltammetry. The consistency of voltage at different scan rates (100–700 mV s−1) from the plot of I/mA vs. E/V for the drug favors a quasi-reversible electrochemical process (Fig. 4a). The anodic and cathodic peaks appeared at 0.396 and 0.243 V, respectively, vs. SCE. In the presence of an increasing concentration (3–12 μM) of DNA, the oxidation and reduction peak potentials of G-18 shifted cathodically with the decrease in peak current. The substantial decay in peak current is attributed to the formation of a slowly diffusing G-18-DNA supramolecular complex resulting in a lowering of the concentration of the free drug (that is mainly responsible for the transfer of current) (Fig. 4b). The DNA binding mode of a drug can be judged from the variation in the formal potential. The obvious negative peak potential shift (cathodic shift) in the CV behavior of surfactant G-18 is related to the electrostatic interaction of its positively charged ferrocenium state with the negatively charged oxygen of DNA. The diffusion coefficient of the surfactant–DNA adduct is 7.31 × 10−8 cm2 s−1, which is far less than the diffusion coefficient of free compound (9.56 × 10−8 cm2 s−1), indicating the slow diffusion of the high molecular weight surfactant–DNA adduct in comparison to the free drug (Fig. 4c). The value of binding constant was obtained through the plot of log
I/Io − I vs. log(1/DNA) as displayed in Fig. 4d.
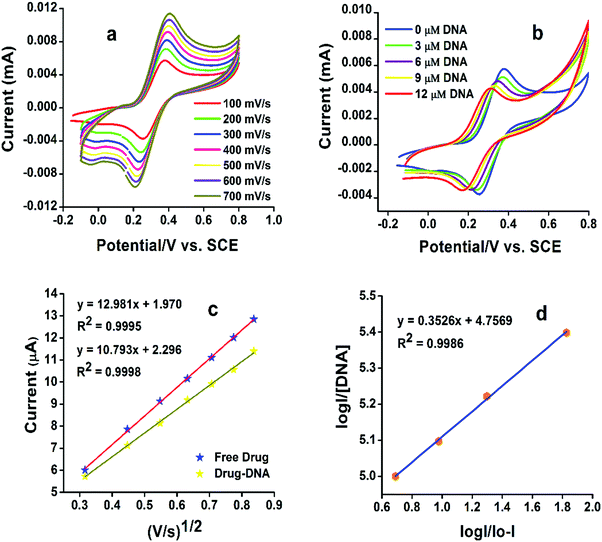 |
| Fig. 4 (a) Representative plots of current vs. potential/V (SCE) at different scan rates for G-18. (b) Cyclic voltammogram of 1 mM G-18 with 1 mL of 0.5 M TBAP as the supporting electrolyte in the absence and presence of 3–12 μM DNA showing a decrease in I from Io and a concentration dependent −ve shift in potential showing electrostatic interactions. (c) Representative plot of current vs. (V s−1)1/2, for the determination of diffusion coefficient of free G-18 and G-18-3 μM DNA. (d) Plot of log (I/Io − I) vs. log (1/[DNA]) for the determination of the binding constant of G-18. | |
The cytotoxic effect of many drugs is due to the interaction with DNA that ultimately inhibits the transcription and replication resulting in cell death.44–47 Helleday et al. have stated that “The control over DNA can control the growth of cancerous cells”.48 A plausible mechanism of the “drug” action of the ferrocenyl guanidines (G-18-G-16, and G-7) is related to the oxidation state of the central iron atom of the ferrocenyl group. The low oxidation potentials of the synthesized surfactants G-18-G-16, and G-7 (0.396 V, 0.432 V, and 0.504 V) as compared to ferrocene (0.518 V) supports the idea that the ferrocenyl moiety may be easily oxidized to the Fe3+ state. It is believed that it is mainly the Fe3+-ferrocenyl cation that undergoes electrostatic interaction with the phosphate backbone of the DNA. Electron transfer reactions involving Fe2+- and Fe3+-ferrocenyl species may generate reactive oxygen species (ROS), such as hydroxyl radicals (˙OH) in vivo that cause damage to DNA, and may also be responsible for the anticancer activity through the formation of radical metabolites that cause biological damage in the cancer cell.49–51
Binding constants obtained from UV-visible spectroscopic and cyclic voltammetric (CV) methods are comparable with each other. The presence of ferrocene enhances the DNA binding activity of guanidines. Ferrocene-based multifunctional alkyl guanidine derivatives, showed binding constants in the high range of 105 to 106 M−1 as compared to the already reported phenyl guanidine derivatives, which implies higher binding affinity to the DNA.27 These binding constant values show a linear dependency on the hydrophobicity of the compound and the longer aliphatic chain compounds exhibited higher binding constants (G-18 < G-16 < G-7). Gibb's free energy (ΔG) is negative for all the reactions which signifies the spontaneity of the compound–DNA interaction. All the DNA binding parameters of the synthesized products are listed in Table 3.
Table 3 Different parameters calculated from the DNA-binding studies (UV, CV)
Code |
Diff. coefficients (Do) (cm2 s−1) |
K
b (CV) M−1 |
K
b (UV) M−1 |
ΔG kJ mol−1 |
Free compound |
Compound + DNA |
G-7
|
7.09 × 10−4 |
5.71 × 10−4 |
2.75 × 105 |
1.22 × 105 |
−29.03 |
G-16
|
5.14 × 10−5 |
4.91 × 10−5 |
3.30 × 105 |
5.13 × 105 |
−33.56 |
G-18
|
9.56 × 10−8 |
7.31 × 10−8 |
6.63 × 106 |
7.60 × 105 |
−35.07 |
3.4 Computational studies
DFT studies were performed to obtain the optimized structures, frontier orbitals, Mulliken charge distribution and other quantum chemical parameters of all the synthesized products. For transition metal systems, the B3LYP version of DFT was found to be more associated. The compounds (G-7, G-16, and G-18) were geometrically optimized in order to calculate the quantum mechanical parameters using the B3LYP/6-31G(d,p) level in the gas phase as displayed in Table 4.33
Table 4 Quantum chemical parameters for ferrocene-based guanidine surfactants in the gas phase at B3LYP/6-31G(d,p)
Sample code |
E
(HOMO) (eV) |
E
(LUMO) (eV) |
Band gap (UV-visible) (eV) |
Band gap (DFT) (eV) |
χ (eV) |
η (eV) |
σ (eV) |
μ (D) |
G-7
|
−5.25312 |
−0.74844 |
4.81 |
4.50 |
3.0011 |
2.25 |
0.444 |
4.4721 |
G-16
|
−5.23530 |
−0.76707 |
4.78 |
4.46 |
3.0007 |
2.23 |
0.448 |
4.5179 |
G-18
|
−5.22666 |
0.77301 |
4.73 |
4.45 |
2.9998 |
2.22 |
0.450 |
4.6187 |
The frontier molecular orbitals (HOMO and LUMO) provide information about the oxidation and reduction sites of the compounds. The associated band gap energies (ΔE) calculated through UV-visible spectroscopy were equally validated by DFT calculations. Fig. S8 (ESI†) shows the optimized geometries of the three newly synthesized guanidines (G-18, G-16-G-7). Fig. 5 demonstrates the contour surfaces of the HOMO and LUMO orbitals of (G-18), whereas the Mulliken charges distribution is illustrated in Fig. 6 (G-18) and Fig. S9 (ESI†) (G-16).
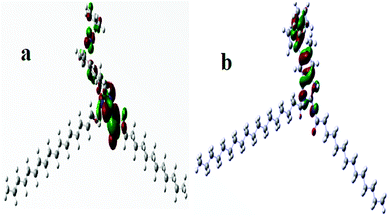 |
| Fig. 5 (a). Representative graphical demonstration of the HOMO orbitals of G-18. (b) Representative graphical demonstration of the LUMO orbitals of G-18. | |
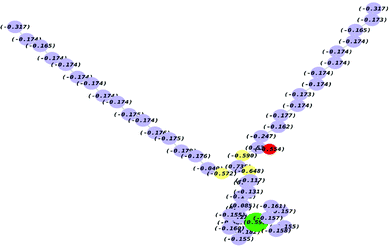 |
| Fig. 6 Representative Mulliken charge distribution on G-18. | |
Ionization energy (I.E.), electron affinity (E.A.), electronegativity (χ), global hardness (η), softness (σ) and dipole moment (μ) were calculated using the following eqn (4)–(9) and their calculated values are listed in Table 4.
The ionization energy (I.E.) and electron affinity (E.A.) refer to the capability of a molecule to donate or accept electrons, respectively. Lower values of EHOMO indicates the greater ability of molecules to donate electrons and vice versa. Electronegativity (χ) can be regarded as the potential of a molecule to act either as a Lewis acid or base. Lewis acids are recognized by higher (χ) values and bases by lower values. Partial electronic transition takes place from lower (Lewis base) to higher (Lewis acid) electronegativity (χ). All the studied surfactants depicted very close χ which ensures that the acidic or basic nature is dependent on the ferrocene (Fe-Cp) moiety and has no relation with the aliphatic chain length. A smaller frontier orbital gap indicates the greater polarizability of a molecule which is mostly associated with greater chemical reactivity and smaller kinetic stability. Global hardness (η) and softness (σ) parameters revealed the molecular stability and reactivity.21 These parameters are closely associated with the band gap in such a way that the hard molecules show large energy gap in comparison to soft molecules. It was observed that the molecules exhibiting a longer alkyl chain have a greater dipole moment and more soft character. These features aid in the easier adsorption which is a prime factor for the determination of surface activity. The hardness values indicate that G-7 is a kinetically more stable molecule, while G-18 is least stable. The higher reactivity of G-18 can be attributed to its longer alkyl chain that enhances the electron donation and makes it more prone to oxidation.
3.5 DPPH scavenging activity
DPPH exhibits a strong absorption band at 517 nm due to its odd electron and produces 1,1-diphenyl-2-picrylhydrazine when an antioxidant reacts with it. As a result, the band intensity of DPPH decreases. This changes the color of DPPH and a corresponding decrease in the absorption. The results obtained are demonstrated in terms of IC50 values (Table 5).
Table 5
In vitro DPPH scavenging activity of ferrocenyl guanidines
Code |
DPPH% scavenging activity |
% Inhibition |
IC50 (μM) |
PC = positive control i.e. ascorbic acid is taken as a reference. |
G-7
|
60 ± 0.23 |
37.6 ± 1.13 |
G16 |
73 ± 0.87 |
25.1 ± 0.98 |
G18 |
81 ± 0.45 |
17.3 ± 0.26 |
PC |
94 ± 1.02 |
9.6 ± 0.45 |
The complex G-18 exhibited a maximum antioxidant activity with a minimum IC50 (half-maximal inhibitory concentration), while compounds G-16 and G-7 showed significant to moderate free radical scavenging capabilities, respectively. Fig. 7 shows a representative plot of absorbance vs. wavelength of compound G-18 with an IC50 value of 17.3 μM.
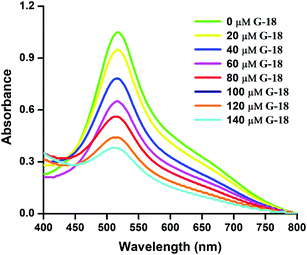 |
| Fig. 7 Representative plots of absorbance vs. wavelength of complex G-18 in DMSO for DPPH scavenging activity. | |
The results of the free radical scavenging assay revealed that the antioxidant potency is attributed to the ferrocenyl guanidine functionality which in turn is enhanced by the introduction of methyl groups to the lipophilic chain of amphiphilic guanidines. The greater electron donation ability of compounds bearing larger aliphatic chain augments the electron transfer aptitude through the molecular skeleton. The facile electron removal and more stabilized radical formation is possible due to easy electron localization from one end of the molecule to another.52
3.6 Cytotoxicity assay
An MTT reduction assay was employed to evaluate the anticancer activity of synthesized complexes (G-18, G-16, and G-7) against MCF-7 carcinoma cells and cisplatin was used as a control. The MTT reduction assay was also used to investigate the efficacy of ferrocenyl derivatives towards the non-cancerous cell line MCF-10A. It was observed that the screened compounds exerted significant anticancer activity (although less than cisplatin) as evidenced from the 50% inhibitory concentration (IC50) data (Table 6). In contrast, these ferrocenyl derivatives exerted fewer toxic effects in normal cells. This variation may be explained by the difference in mechanism through which ferrocenyl derivatives and cisplatin interact with the DNA.53 It is known that cisplatin interacts with nitrogenous bases in the DNA through covalent bonding. In contrast, the interaction of the synthesized compounds with DNA might involve electrostatic interaction, as indicated through electrochemical and spectroscopic studies. Indeed, the inhibitory effect of the tested compounds on the growth of tumor cells followed a dose-dependent manner (Fig. 8).
Table 6 Cytotoxicity of synthesized compounds (G-18-G-16, G-7) against human tumor (MCF-7) and normal cells (MCF-10A) after 72 h of incubation in comparison with cisplatin
IC50 (μM) |
Sample code |
MCF-7 |
MCF-10A |
G-7
|
6.19 ± 0.73 |
10.98 ± 0.57 |
G-16
|
4.65 ± 0.85 |
12.64 ± 0.33 |
G-18
|
3.02 ± 0.48 |
14.15 ± 0.60 |
Cisplatin |
1.55 ± 1.51 |
17.34 ± 0.75 |
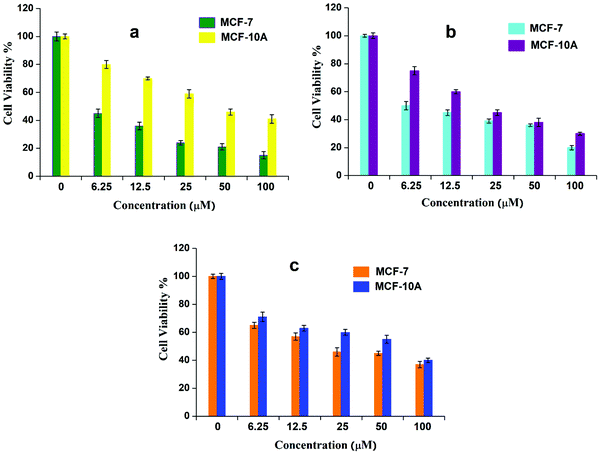 |
| Fig. 8 Cell viability (%) of cancerous and non-cancerous cells at various concentrations (0–100 μM) of (a) G-18, (b) G-16, and (c) G-7, after 72 h of incubation. | |
The time-dependent response of the ferrocenyl guanidines was also evaluated after regular intervals (24–120 h). The inhibitory responses against the cancerous cells were found to be maximum at 72 h incubation, while negligible change in cell viability was observed beyond this time (Fig. S10, ESI†).
The results of the cytotoxicity assays indicate that those complexes possessing long aliphatic chains demonstrated improved cell growth inhibitory effects. This indicates that the presence of electron-donating alkyl groups augments the lipophilicity and helps the molecules to interact or penetrate more through the cell membrane, thereby enhancing their cytotoxic action. The higher anticancer activity of G-18 and G-16 can be associated with the greater hydrophobicity of the longer alkyl chain which in turn decreases the basicity of guanidines.54
4. Conclusions
The work reported herein describes the synthesis of three new ferrocene-based guanidines having different alkyl chain lengths. The compounds were highly pure, as characterized by different spectroscopic methods in the solid state as well as in solution. Low CMC, high binding constant values, and significant antioxidant and cytotoxic activities have proved to be a smart approach to control their therapeutic and interfacial properties by incorporating a lipophilic alkyl chain. This idea of introducing an aliphatic chain into the structure of efficient drugs may prove valuable for the rational design of new drug delivery systems.
Conflicts of interest
There are no conflicts of interest to declare.
Acknowledgements
Faiza Asghar is highly obliged to the Higher Education Commission (HEC) of Pakistan for providing funding for this research.
References
-
S. Patai, The chemistry of amidines and imidates, Wiley. 1975 Search PubMed.
-
(a) R. G. S. Berlinck, A. C. B. Burtoloso and M. H. Kossuga, The chemistry and biology of organic guanidine derivatives, Nat. Prod. Rep., 2008, 25, 919 RSC;
(b) G. J. Durant, G. M. Smith, R. G. Spickett and S. H. Wright, Biologically active guanidines and related compounds. II. Some antiinflammatory aminoguanidines, J. Med. Chem., 1966, 9, 22 CrossRef CAS PubMed;
(c) K. A. Schug and W. Lindner, Non covalent bonding between guanidinium and anionic groups: focus on biological and synthetic based argininium/guanidinium interactions with phosp[on]ate and sulf[on]ate residues, Chem. Rev., 2005, 105, 67 CrossRef CAS PubMed.
- G. Murtaza, A. Badshah, M. Said, H. Khan, A. Khan, S. Khan, S. Siddiq, M. I. Choudhary, J. Boudreau and F. G. Fontaine, Urease inhibition and anti-leishmanial assay of substituted benzoyl guanidines and their copper(II) complexes, Dalton Trans., 2011, 40, 9202 RSC.
- G. Murtaza, M. K. Rauf, A. Badshah, M. Ebihara, M. Said, M. Gielen, D. deVos, E. Dilshad and B. Mirza, Synthesis, structural characterization and in vitro biological screening of some homoleptic copper(II) complexes with substituted guanidines, Eur, J. Med. Chem., 2011, 48, 26 Search PubMed.
- L. Meurling, M. Marquez, S. Nilsson and A. R. Holmberg, Polymer-conjugated guanidine is a potentially useful anti-tumor agent, Int. J. Oncol., 2009, 35, 281 CAS.
- S. L. Li, C. H. Huang, C. C. Lin, Z. N. Huang, J. H. Chern, H. Y. Lien, Y. Y. Wu, C. H. Cheng, C. Y. Chang and J. Y. Chuu, Antitumor effect of BPR-DC-2, a novel synthetic cyclic cyanoguanidine derivative, involving the inhibition of MDR-1 expression and down-regulation of p-AKT and PARP-1 in lung cancer, Invest. New Drugs, 2011, 29, 195 CrossRef CAS.
- Z. Brzozowski, F. Saczewski and M. Gdaniec, Synthesis, molecular structure and anticancer activity of 1-allyl-3-amino-2-(4-chloro-2-mercaptobenzenesulphonyl) guanidine derivatives, Eur. J. Med. Chem., 2002, 37, 285 CrossRef CAS.
- M. K. Rauf, R. Gul, Z. Rashid, A. Badshah, M. N. Tahir, M. Shahid and A. Khan, Synthesis, characterization, DNA binding and in vitro antimicrobial studies of a novel tetra-substituted N-Isopropyl-N-(4-ferrocenylphenyl)-N′-(2,6-diethylphenyl)-N′′-benzoylguanidine: crystallographic structure and quantum chemical computations, Spectrochim. Acta, Part A, 2015, 136, 1099 CrossRef CAS PubMed.
- P. J. Hajduk, S. Boyd, D. Nettesheim, V. Nienaber, J. Severin, R. Smith, D. Davidson, T. Rockway and S. W. Ferik, Identification of novel inhibitors of urokinase via NMR based screening, J. Med. Chem., 2000, 43, 3862 CrossRef CAS PubMed.
- E. J. Iwanowicz, S. H. Watterson, C. Liu, H. H. Gu, T. Mitt, K. Leftheris, J. C. Barrish, C. A. Fleener, K. Rouleau, N. Z. Sherbina and D. L. Hollenbaugh, Novel guanidine-Based inhibitors of inosine monophosphate dehydrogenase, Bioorg. Med. Chem. Lett., 2002, 12, 2931 CrossRef CAS.
- A. W. Tai, E. J. Lien, M. M. C. Lai and T. A. Khwaja, Novel N-hydroxyguanidine derivatives as anticancer and antiviral agents, J. Med. Chem., 1984, 27, 236 CrossRef CAS.
- P. Sima and V. Vetvicka, Bioactive substances with anti-neoplastic efficacy from marine invertebrates: porifera and coelenterata, World J. Clin. Oncol., 2011, 2, 355 CrossRef PubMed.
- K. Ohara, M. Smietana, A. Restouin, S. Mollard, J. Borg, Y. Collette and J. Vasseur, Amine-guanidine switch: a promising approach to improve DNA binding and antiproliferative activities, J. Med. Chem., 2007, 50, 6465 CrossRef CAS PubMed.
- R. J. T. Houk, S. L. Tobey and E. V. Anslyn, Abiotic guanidinium receptors for anion molecular recognition and sensing, Top. Curr. Chem., 2005, 255, 199 CrossRef CAS.
-
(a) T. Shimizu, H. Hamakawa, T. Tozuka and H. Ono, Pharmacological effects of [((octahydro-2-azocinyl)methyl) guanidine (guanazodine)]: Its action mechanism and hypotensive effect, Folia Pharmacol. Jpn., 1976, 72, 837 CrossRef CAS;
(b) B. J. Padanilam, Cell death induced by acute renal injury: a perspective on the contributions of apoptosis and necrosis, Am. J. Physiol. Renal. Physiol., 2003, 284, F608 CrossRef CAS PubMed.
- S. Top, A. Vessieres, G. Leclercq, J. Quivy, J. Tang, J. Vaissermann, M. Huche and G. Jaouen, Synthesis, biochemical properties and molecular modelling studies of organometallic specific estrogen receptor modulators (SERMs), the ferrocifens and hydroxyferrocifens: Evidence for an antiproliferative effect of hydroxyferrocifens on both hormone-dependent and hormone-independent breast cancer cell lines, Chem. – Eur. J., 2003, 9, 5223 CrossRef CAS.
- C. Biot, N. Chavain, F. Dubar, B. Pradines, X. Trivelli, J. Brocard, I. Forfar and D. Dive, Structure-activity relationships of 4-N-substituted ferroquine analogues: Time to re-evaluate the mechanism of action of ferroquine, J. Organomet. Chem., 2009, 694, 845 CrossRef CAS.
- Q. Z. Liu, Potential applications of ferrocene as a structural feature in antioxidants, Mini-Rev. Med. Chem., 2011, 11, 345 CrossRef PubMed.
- H. Kuwahara, M. Hamada, Y. Ishikawa and T. Kunitake, Self-organization of bilayer assemblies in a fluorocarbon medium, J. Am. Chem. Soc., 1993, 115, 3002 CrossRef CAS.
- Y. Ishikawa, H. Kuwahara and T. Kunitake, Self-assembly of bilayers from double chain fluorocarbon amphiphiles in aprotic organic solvents: thermodynamic origin and generalization of the bilayer assembly, J. Am. Chem. Soc., 1994, 116, 5579 CrossRef CAS.
- S. Ali, A. Badshah, H. Hussain, S. Fatima, A. H. Shah, A. Shah, S. Saifullah and M. R. Shah, Synthesis, characterization, and computational study of new ferrocene based Schiff bases as potential nonionic surfactants, J. Surfactants Deterg., 2019, 22, 897 CrossRef CAS.
- S. Fatima, A. Badshah, B. Lal, F. Asghar, M. N. Tahir, A. Shah and I. Ullah, Study of new ferrocene based thioureas as potential non-ionic surfactants, J. Organomet. Chem., 2016, 819, 194 CrossRef CAS.
- E. W. Kaler, A. K. Murthy, B. E. Rodriguez and J. Zasadzinski, Spontaneous vesicle formation in aqueous mixtures of single-tailed surfactants, Sci, 1989, 245, 1371 CrossRef CAS.
- F. Asghar, S. Fatima, S. Rana, A. Badshah, I. S. Butler and M. N. Tahir, Synthesis, spectroscopic investigation, and DFT study of N,N′-disubstituted ferrocene-based thiourea complexes as potent anticancer agents, Dalton Trans., 2018, 47, 1868 RSC.
- F. Asghar, S. Rana, S. Fatima, A. Badshah, B. Lal and I. S. Butler, Biologically active halo-substituted ferrocenyl thioureas: Synthesis, spectroscopic characterization, and DFT calculations, New J. Chem., 2018, 42, 7154 RSC.
- R. Gul, A. Khan, A. Badshah, M. K. Rauf, A. Shah, Z. U. Rehman, A. Bano, R. Naz and M. N. Tahir, New supramolecular ferrocenyl phenylguanidines as potent antimicrobial and DNA-binding agents, J. Coord. Chem., 2013, 66, 1959 CrossRef CAS.
- R. Gul, M. K. Rauf, A. Badshah, S. S. Azam, M. N. Tahir and A. Khan, Ferrocene-based guanidine derivatives: In vitro antimicrobial, DNA binding and docking supported urease inhibition studies, Eur. J. Med. Chem., 2014, 85, 438 CrossRef CAS.
- R. Gul, Z. Nawaz, A. Badshah, A. Khan, A. Junaid, R. Naz, M. K. Rauf and S. Hayat, Synthesis and Biological Evaluation of Some New Ferrocenyl Phenyl Guanidines as Antibacterial, Antifungal Agents, J. Drug. Des. Med. Chem., 2016, 2, 35 Search PubMed.
- I. Ullah, A. Naveed, A. Shah, A. Badshah, Z. U. Rehman, G. S. Khan and A. Nadeem, High yield synthesis, detailed spectroscopic characterization and electrochemical fate of novel cationic surfactants, J. Surfactants Deterg., 2014, 17, 243 CrossRef CAS.
-
(a) E. I. Rogers, D. S. Silvester, D. L. Poole, L. Aldous, C. Hardacre and R. G. Compton, Voltammetric characterization of the Ferrocene Ferrocenium and Cobaltocenium Cobaltocene Redox Couples in RTILs, J. Phys. Chem. C, 2008, 112, 2729 CrossRef CAS;
(b) S. L. Shen, J. H. Shao, J. Z. Luo, J. T. Liu, J. Y. Miao and B. X. Zhao, Novel chiral ferrocenylpyrazolo[1,5-a][1,4]diazepin-4-one derivatives: synthesis, characterization and inhibition against lung cancer cells, Eur. J. Med. Chem., 2013, 63, 256 CrossRef CAS PubMed.
- S. Zubair, F. Asghar, A. Badshah, B. Lal, R. A. Hussain, S. Tabassum and M. N. Tahir, New bioactive ferrocene-substituted heteroleptic copper(I) complex: synthesis, structural elucidation, DNA interaction, and DFT study, J. Organomet. Chem., 2019, 879, 60 CrossRef CAS.
- R. A. Hussain, A. Badshash, M. Sohail, B. Lal and A. A. Altaf, Synthesis, chemical characterization, DNA interaction and antioxidant studies of ortho, meta and para fluoro substituted ferrocene incorporated selenoureas, Inorg. Chim. Acta, 2013, 402, 133 CrossRef CAS.
- S. Fatima, R. Sharma, F. Asghar, A. Kamal, A. Badshah and H. B. Kraatz, Study of New Amphiphiles based on Ferrocene containing Thioureas as Efficient Corrosion Inhibitors: Gravimetric, Electrochemical, SEM and DFT Studies, J. Ind. Eng. Chem., 2019, 76, 374 CrossRef CAS.
- F. Asghar, A. Badshah, R. A. Hussain, M. Sohail, K. Akbar and I. S. Butler, Synthesis, structural characterization, DNA binding and antioxidant potency of new ferrocene incorporated acyl ureas, J. Organomet. Chem., 2015, 797, 131 CrossRef CAS.
-
(a) S. Zouari, M. Ketata, N. Boudhrioua and E. Ammar,
Allium roseum L. volatile compounds profile and antioxisdant activity for chemotype discrimination–Case study of the wild plant of Sfax (Tunisia), Ind. Crop Prod., 2013, 41, 172 CrossRef CAS;
(b) A. Choudhary, R. Sharma, M. Nagar, M. Mohsin and H. Meena, Synthesis, characterization and antioxidant activity of some transition metal complexes with terpenoid derivatives, J. Chil. Chem. Soc., 2011, 56, 911 CrossRef CAS.
- B. Lal, A. Badshah, A. A. Altaf, M. N. Tahir, S. Ullah and F. Huq, Study of new ferrocene incorporated N,N′-disubstituted thioureas as potential antitumor agents, Aus. J. Chem, 2013, 66, 1352 CrossRef CAS.
- T. Mosmann, Rapid colorimetric assay for cellular growth and survival: application to proliferation and cytotoxicity assays, J. Immunol. Methods, 1983, 65, 55 CrossRef CAS.
- F. Asghar, A. Badshah, B. Lal, I. S. Butler, S. Tabassum and M. N. Tahir, Bioactivity of new ferrocene incorporated N,N′-disubstituted ureas: Synthesis, structural elucidation and DFT study, Inorg. Chim. Acta, 2016, 439, 82 CrossRef CAS.
- J. Binoy, C. James, I. H. Joe and V. S. Jayakumar, Vibrational analysis and Y-aromaticity in bis (N,N′-diphenyl guanidinium) oxalate crystal: a DFT study, J. Mol. Struct., 2006, 784, 32 CrossRef CAS.
- I. Ullah, A. Shah, A. Badshah, A. Shah, N. A. Shah and R. Tabor, Surface, aggregation properties and antimicrobial activity of four novel thiourea-based non-ionic surfactants, Colloids Surf., A, 2015, 464, 104 CrossRef CAS.
- R. Fukuda, S. Takenaka and M. Takagi, Metal ion assisted DNA-intercalation of crown ether-linked acridine derivatives, J. Chem. Soc., Chem. Commun., 1990, 0, 1028 RSC.
-
C. R. Cantor and P. R. Schimmel, Biophysical Chemistry, W. H. Freeman & Co., San Franciso, 1980 Search PubMed.
- C. C. Ju, A. G. Zhang, C. L. Yuan, X. L. Zhao and K. Z. Wang, The interesting DNA-binding properties of three novel dinuclear Ru(II) complexes with varied lengths of flexible bridges, J. Inorg. Biochem., 2011, 105, 435 CrossRef CAS PubMed.
- E. R. Jamieson and S. J. Lippard, Structure, Recognition, and processing of cisplatin-DNA adducts, Chem. Rev., 1999, 99, 2467 CrossRef CAS.
- R. A. Alderden, M. D. Hall and T. W. Hambley, The discovery and development of cisplatin, J. Chem. Ed, 2006, 83, 728 CrossRef CAS.
- T. W. Hambley, Platinum binding to DNA: structural controls and consequences, J. Chem. Soc., Dalton Trans., 2001, 2711 RSC.
- R. J. Knox, F. Friedlos, D. A. Lydall and J. J. Roberts, Mechanism of cytotoxicity of anticancer platinum drugs: evidence that cis-diamminedichloroplatinum(II) and cis-diammine-(1,1-cyclobutanedicarboxylato)platinum(II) differ only in the kinetics of their interaction with DNA, Cancer Res., 1986, 46, 1972 CAS.
- T. Helleday, J. Lo, D. C. V. Gent and B. P. Engelward, DNA double-strand break repair: from mechanistic understanding to cancer treatment, DNA Repair, 2007, 6, 923 CrossRef CAS.
- D. Osella, M. Ferrali, P. Zanello, F. Laschi, M. Fontani, C. Nervi and G. Cavigiolio, On the mechanism of the antitumor activity of ferrocenium derivatives, Inorg. Chim. Acta, 2000, 306, 42 CrossRef CAS.
- D. Osella, H. Mahboobi, D. Colangelo, G. Cavigiolio, A. Vessieres and G. Jaouen, FACS analysis of oxidative stress induced on tumour cells by SERMs, Inorg. Chim. Acta, 2005, 358, 1993 CrossRef CAS.
- S. Saeed, N. Rashid, P. G. Jones, M. Ali and R. Hussain, Synthesis, characterization and biological evaluation of some thiourea derivatives bearing benzo thiazole moiety as potential antimicrobial and anticancer agents, Eur. J. Med. Chem., 2010, 45, 1323 CrossRef CAS PubMed.
- J. Patujo, M. Azeem, M. Khan, H. Muhammad, R. Ahmad, S. Fatima, B. Mirza, Z. Hussain and A. Badshah, Assessing the biological potential of new symmetrical ferrocene based bisthiourea analogues, Bioorg. Chem., 2020, 106, 104180 CrossRef.
- H. Tayyem, F. Huq, J. Q. Yu, P. Beale and K. Fisher, Synthesis and activity of a trinuclear platinum complex: [{trans-PtCl(NH3)2}2μ-{trans-Pt(3-hydroxypyridine)2(H2N(CH2)6NH2)2}] Cl4 in ovarian cancer cell lines, Chem. Med. Chem., 2008, 3, 145 CrossRef CAS.
- R. Gul, A. Badshah, A. Khan, A. Junaid and M. K. Rauf, Preliminary investigation of anticancer activity by determining the DNA binding and antioxidant potency of new ferrocene incorporated N,N′,N′′-trisubstituted phenylguanidines, Spectrochim. Acta, Part A, 2014, 117, 264 CrossRef CAS.
Footnotes |
† Electronic supplementary information (ESI) available. See DOI: 10.1039/d1nj03424c |
‡ Faiza Asghar and Jahangeer Patujo contributed equally. |
|
This journal is © The Royal Society of Chemistry and the Centre National de la Recherche Scientifique 2022 |