DOI:
10.1039/D1MA01247A
(Paper)
Mater. Adv., 2022,
3, 3267-3277
Improving the temperature-sensing performance of the SrZn0.33Nb0.67O3:Pr3+ phosphor via Ga3+ doping†
Received
27th December 2021
, Accepted 21st February 2022
First published on 23rd February 2022
Abstract
Optical thermometry offers promising applications in the fields of microelectronics and biomedicine, as well as in fire pre-warning systems. In this research, Pr3+-activated SrZn0.33Nb0.67O3 phosphors were successfully prepared via solid-state reactions. Under ultraviolet excitation, the SrZn0.33Nb0.67O3:x%Pr3+ (x = 0.25/0.75/1.25/2) phosphors exhibit bright blue and red emissions, located at 491 nm (3P0 → 3H4), 619 nm (1D2 → 3H4) and 651 nm (3P0 → 3F2). Specifically, two fluorescence intensity ratio (FIR) models of 1D2 → 3H4versus3P0 → 3H4 (Red1/Blue) and 1D2 → 3H4versus3P0 → 3F2 (Red1/Red2) in the temperature range of 300–500 K are adopted for temperature sensing. Our results show that the designed phosphor can serve as a novel potential self-calibrated optical thermometer. Moreover, the temperature sensitivity of the FIR thermometer is significantly enhanced when Ga3+ is incorporated into the SrZn0.33Nb0.67O3. Specifically, the maximum absolute and relative sensitivity for the 6% Ga3+ co-doped sample are increased five- and two-fold, respectively, compared with the undoped sample. This work may provide useful inspiration for effectively improving the temperature-sensing performance of optical thermometers.
Introduction
With rapid developments in the microelectronics industry, biomedical field and scientific research, accurate temperature information acquisition with high spatio-temporal resolution has been gaining greater importance. Compared with traditional mercury-in-glass thermometers and thermocouples, rare-earth-doped non-contact optical thermometers are becoming more and more popular for temperature measurements in harsh environments, such as in corrosive liquids and in environments with electromagnetic interference.1–4 Generally, the emission intensity/bandwidth, peak position, fluorescence intensity ratio (FIR) and lifetime of the luminescence center can all be used as indexes for temperature monitoring.5 Among these, the FIR technique has wide-ranging advantages due to its ease of operation, fast response and high reliability.6–9
Over the past decades, most of the studies based on FIR thermometry have focused on Er3+-doped up-conversion temperature-sensing materials that have two closely spaced temperature-sensitive thermally coupled levels (2H11/2, and 4S3/2).10–12 However, the narrow energy gap of the thermally coupled levels results in poor signal discrimination. Other luminescent materials, such as fluorescein dye, carbon dots, quantum dots, etc., have also been investigated for FIR thermometry.13–15 However, these materials are usually vulnerable to the physical and chemical environments, which restricts their practical application. Currently, the temperature-sensing strategy based on the diverse temperature responses of 4f–5d or 4f–4f transitions of Pr3+ is attracting much attention for its high signal discrimination and considerable temperature sensitivity.16–20 Pr3+ has abundant emission spectral lines, where blue, green, red and deep red emissions are derived from the 3P0 → 3H4, 3P1,0 → 3H5, 1D2 → 3H4 and 3P0 → 3F2 electronic transitions, respectively. Among these, the blue and red emissions that stem from the 3P0 and 1D2 states are usually used as signal peaks for research in FIR thermometry. As is known, influences from phonon-assisted cross-relaxation, multiphonon relaxation and the intervalence charge transfer state (IVCT) mean that the intensity ratio of these transitions is very vulnerable to temperature in some transition metal oxides. For example, Pr3+-doped (K0.5Na0.5)NbO3, La2MgTiO6, Na2La2Ti3O10 and LaMg0.402Nb0.598O3 have been reported as potential optical thermometers.21–24 It is worth noting that IVCT-affected thermal-quenching process is the key factor that contributes to the excellent temperature-sensing performance. Hence, choosing a suitable host and regulating the IVCT band are effective ways to achieve a high temperature-sensing performance.
The complex perovskite compound SrZn0.33Nb0.67O3 has drawn our attention. This compound belongs to the A3B′B′′2O9 family in which the A-sites are occupied by larger cations and the B-sites by smaller cations. The abundant lattice sites, wide spectral excitation and excellent chemical stability make it a suitable matrix for developing rare-earth luminescent materials.25–27 To the best of our knowledge, the thermo-sensitive properties of the SrZn0.33Nb0.67O3:Pr3+ phosphor have not been systematically investigated so far. Therefore, a study on the temperature-dependent luminescent properties of Pr3+ in SrZn0.33Nb0.67O3 will broaden the family of potential Pr3+-activated optical thermometers. In addition, co-doping with other ions is an effective way to achieve enhancement of the temperature-sensing performance.28–30 For example, the green up-conversion emission and thermal sensitivity of NaYF4:Yb,Er3+ are both increased when Ga3+ is introduced into the host.31 The luminescent intensity of CaAl12O19:Mn4+ is enhanced via Ga3+ doping.32 The thermal stability and red emission of Mn4+ in Li2MgZrO4 are improved when Ga3+ ions are incorporated into the phosphor.33 The above studies indicate a practicable strategy for us to promote the performance of Pr3+-activated self-calibrated optical thermometers.
Thus, in this work, we chose to study a novel Pr3+-activated SrZn0.33Nb0.67O3 thermo-sensitive phosphor and investigate the significant impact of Ga3+ co-doping on the temperature-sensing performance of this material. Herein, the SrZn0.33Nb0.67O3:x%Pr3+ (x = 0.25/0.75/1.25/2) phosphors were successfully prepared using a high-temperature solid-state method, and the impact of the Pr3+ concentration on the luminescence properties is discussed. Under ultraviolet excitation, the as-synthesized samples exhibit efficient blue and red emissions located at 491 nm (3P0 → 3H4), 619 nm (1D2 → 3H4) and 651 nm (3P0 → 3F2), and the FIR of different 4f configuration transitions changes significantly with temperature, demonstrating that the studied material can serve as a self-calibrated optical thermometer with good signal discrimination. Specifically, two FIR models of 1D2 → 3H4versus3P0 → 3H4 (Red1/Blue) and 1D2 → 3H4versus3P0 → 3F2 (Red1/Red2) in the temperature range of 300–500 K are adopted for temperature sensing. Notably, the temperature-sensing performance of the thermometer is improved markedly when Ga3+ is co-doped into the SrZn0.33Nb0.67O3:Pr3+ phosphor. It is hoped that the designed phosphor will be a potential candidate for applications in optical thermometry.
Experimental
Synthesis
The SrZn0.33Nb0.67O3:x%
Pr3+,y%
Ga3+(x = 0.25/0.75/1.25/2; y = 4/6/8) samples were synthesized using the high-temperature solid-state method. The strontium carbonate (SrCO3), zinc oxide (ZnO), niobium oxide (Nb2O5), praseodymium oxide (Pr6O11), and gallium oxide (Ga2O3) used in the experiments were purchased from the Aladdin Reagent Company. In a typical synthesis, stoichiometric amounts of SrCO3, Nb2O5, ZnO, Pr6O11, and Ga2O3 were mixed thoroughly and ground with flux using ethanol as a wetting agent, placed in a corundum crucible and heated at 800 °C for 2 h in air. After cooling to room temperature, the powder was ground and calcined at 1350 °C for 8 h in air.
Characterization
The X-ray diffraction (XRD) patterns were collected using a powder diffractometer with Cu Kα radiation (λ = 1.5418 Å). A scanning electron microscope (SEM, JSM-6700F) was used to characterize the morphology, energy dispersive X-ray spectroscopy (EDS) and elemental mapping of the samples. Diffuse reflectance spectra were recorded using a UV-Vis-NIR spectrophotometer (Lambda 950, PerkinElmer). An FLS 980 fluorescence spectrometer with a 450 W xenon lamp as the excitation light source was used to measure the photoluminescence excitation (PLE) and photoluminescence emission (PL) spectra and the fluorescence decay curves of the samples. Temperature-dependent PL spectra were measured using the above-mentioned spectrophotometer, which was equipped with a temperature-controlling stage.
Results and discussion
Fig. 1(a) shows the XRD patterns of SrZn0.33Nb0.67O3:x%
Pr3+,y%
Ga3+ (x = 0/0.25/0.75/1.25/2; y = 4/6/8). All the diffraction peaks can be well indexed to the standard PDF card (no. 39-1470) with no impurity peaks observed, which confirms that the synthesized samples are of pure phase. The doping of Pr3+ and Ga3+ ions did not change the crystal structure of SrZn0.33Nb0.67O3. When Pr3+ and Ga3+ ions are incorporated into SrZn0.33Nb0.67O3, the position of the diffraction peaks shifts to the larger-angle side, as exhibited in Fig. 1(a) for the strongest line located at 31.6°. Fig. 1(b) presents the crystal structure of SrZn0.33Nb0.67O3, which belongs to the cubic perovskite structure in the space group Fm
m. Zn and Nb atoms are disorderly distributed at the B site, coordinated with 6 oxygen atoms to form [Zn/NbO6] octahedra, while the Sr2+ ion is located at the A site with 12-fold coordinated oxygen. Due to the similar ionic radii of Pr3+ and Sr2+ (rPr3+ = 1.29 Å for CN = 12, rSr2+ = 1.44 Å for CN = 12), Pr3+ should substitute for the Sr2+ ion. By contrast, the ionic radius of Ga3+ (rGa3+ = 0.62 Å, CN = 6) is similar to that of the Nb5+ and Zn2+ ions (rZn2+ = 0.74 Å, rNb5+ = 0.64 Å for CN = 6), so the Ga3+ ion should occupy the Zn2+/Nb5+ site. SEM and elemental map observations, as displayed in Fig. 1(c–j), show that the as-prepared SrZn0.33Nb0.67O3:6%
Ga3+,1.25%
Pr3+ powder has a small particle size below 5 μm, and the Sr, Zn, Nb, O and Ga elements are homogeneously distributed throughout the observation area. Moreover, the peaks of the Sr, Zn, Nb, O and Ga elements all appear in the EDS energy spectrum, further proving the successful incorporation of Ga3+ ions into niobate matrix.
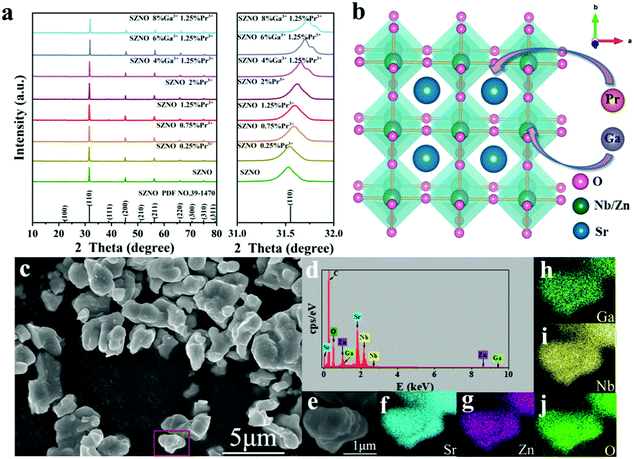 |
| Fig. 1 (a) XRD patterns of SrZn0.33Nb0.67O3:x% Pr3+,y% Ga3+ (x = 0/0.25/0.75/1.25/2; y = 4/6/8), referenced to the SrZn0.33Nb0.67O3 (PDF no. 39-1470) standard card. Magnification of patterns in the 31–32° range are on the right. (b) Schematic diagram of the SrZn0.33Nb0.67O3 crystal structure. (c) SEM image, (d) EDS spectrum and (e–j) elemental mapping images of SrZn0.33Nb0.67O3:1.25% Pr3+,6% Ga3+. | |
Fig. 2(a) shows the room-temperature PL spectra of the SrZn0.33Nb0.67O3 phosphors doped with different Pr3+-ion concentrations. It is found that under the excitation of 290 nm UV light, the as-prepared SrZn0.33Nb0.67O3:x% Pr3+ (x = 0.25/0.75/1.25/2) samples exhibit three main emission peaks at 491 nm, 619 nm and 651 nm, which correspond to the 3P0 → 3H4, 1D2 → 3H4 and 3P0 → 3F2 transitions of Pr3+, respectively. The emission intensity of the three emission peaks (491 nm, 619 nm and 651 nm) have similar line trends with the Pr3+ doping concentration. When the Pr3+ doping concentration was increased from 0.25% to 2%, the emission intensities of the peaks were all gradually increased, and any further increase in the doping concentration resulted in a lowered emission intensity due to concentration quenching; therefore, the optimal Pr3+ doping concentration is 1.25%. In order to investigate the energy-transfer mechanism of Pr3+ in the SrZn0.33Nb0.67O3 host, the critical distance Rc is calculated using the following equation,34
| 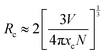 | (1) |
where
V is the unit cell volume,
xc is the critical doping concentration, and
N represents the number of lattice sites in the unit that can be occupied by activator ions. For SrZn
0.33Nb
0.67O
3:Pr
3+,
V = 64.1 Å
3,
xc = 1.25,
N = 2, and
Rc was calculated to be 3.68 Å; thus, the electric exchange interaction is responsible for the non-radiative energy transfer in SrZn
0.33Nb
0.67O
3.
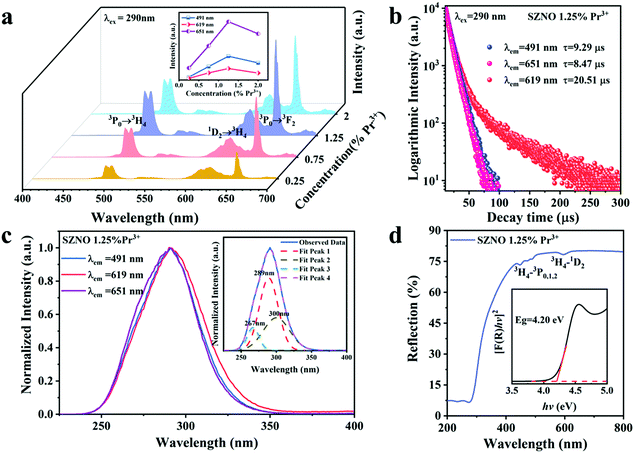 |
| Fig. 2 (a) PL spectra of SrZn0.33Nb0.67O3:x% Pr3+ (x = 0.25/0.75/1.25/2) at room temperature. The inset shows a comparison of the emission intensity for the 491 nm, 619 nm and 651 nm peaks for different Pr3+-ion concentrations. (b) Fluorescence decay curves and (c) excitation spectra (λem = 491 nm, 619 nm, and 651 nm) for SrZn0.33Nb0.67O3:1.25% Pr3+. (d) ultraviolet visible diffuse reflectance spectra (UV vis DRS) of the SrZn0.33Nb0.67O3:1.25% Pr3+, where the inset shows the relationship of [F(R)hν]2versus the energy hν. | |
Fig. 2(b) exhibits the fluorescence decay curves measured at the 491 nm (3P0 → 3H4), 619 nm (1D2 → 3H4), and 651 nm (3P0 → 3F2) emissions for SrZn0.33Nb0.67O3:1.25%
Pr3+. All curves exhibit triple-exponential decay behaviour, and can be fitted using the following formula,35
|  | (2) |
where
I(
t) is the fluorescence intensity of the emission peak monitored at time
t;
B1,
B2 and
B3 are constants, and
τ1,
τ2 and
τ3 are the lifetimes of each exponential component. The deviation from single-exponential decay is due to the existence of several de-excitation pathways including the radiative transition, cross-relaxation (CR) and multiphonon relaxation (MPR) processes as well as the intervalence charge transfer state (IVCT). The average lifetime (
τ) can be calculated:
| 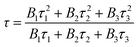 | (3) |
Finally, the fluorescence decay lifetime of the 1D2 energy level by via monitoring at the 619 nm emission is 20.51 μs, and the decay lifetime of the 3P0 level by monitoring at the 491 nm and 651 nm emissions are calculated as 9.29 μs and 8.47 μs, respectively. Due to its spin-allowed transition characteristics, the excited electrons at the 3P0 energy level decay much faster than at the 1D2 energy level. Fig. 2(c) shows the normal temperature PLE spectra of SrZn0.33Nb0.67O3:1.25% Pr3+ measured by monitoring the emission peaks at λem = 491 nm, 619 nm, and 651 nm. It is found that all the excitation spectra are similar, and the maximum excitation wavelength is around 290 nm. The inset of Fig. 2(c) shows the Gaussian fitting result of one typical PLE spectrum, where the three Gaussian deconvolution peaks at 267 nm, 289 nm and 300 nm are suggested to be assigned to the 4f2 → 4f5d transition of Pr3+, the host absorption and Pr3+–Nb5+ IVCT. From the diffuse reflectance spectra of the SrZn0.33Nb0.67O3 host and SrZn0.33Nb0.67O3:1.25%
Pr3+ displayed in Fig. S1 (ESI†) and Fig. 2(d), respectively, both show a strong host absorption in the 200–400 nm UV region. Besides the host fundamental absorption, there also exist several absorption dips in the 450–500 nm and 580–625 nm regions of SrZn0.33Nb0.67O3:1.25%
Pr3+, which originate from the 4f–4f intra-configuration transitions of the Pr3+ ion, i.e., the 3H4–3P0,1,2 and the 3H4–1D2 transition, respectively. Due to the parity-forbidden transition nature, their absorptions are very weak. According to the Tauc theoretical equation, the optical band gap of SrZn0.33Nb0.67O3 can be calculated using the following formula:
| [F(R)hν]n = A(hν − Eg) | (4) |
where
h is the Planck constant,
v is the incident photon frequency,
A and
Eg are the proportional constant and the optical band gap energy,
n is related to the transition type,
i.e.,
n = 1/2 for an indirect transition, and
n = 2 for a direct transition, and
R is the reflection coefficient. In the SrZn
0.33Nb
0.67O
3 host, the value of
n should be 2 due to it is direct bandgap.
25 The inset of
Fig. 2(d) shows the function relationship between [
F(
R)
hv]
2 and
hv for SrZn
0.33Nb
0.67O
3:1.25%
![[thin space (1/6-em)]](https://www.rsc.org/images/entities/char_2009.gif)
Pr
3+. The optical band gaps of the SrZn
0.33Nb
0.67O
3 host and SrZn
0.33Nb
0.67O
3:1.25%
![[thin space (1/6-em)]](https://www.rsc.org/images/entities/char_2009.gif)
Pr
3+ were calculated to be about 4.20 eV.
Next, temperature-dependent photoluminescence spectrum testing was performed on SrZn0.33Nb0.67O3:1.25%
Pr3+ to investigate its potential applications in temperature sensing. As shown in Fig. 3(a), with the temperature increasing from 300–500 K, the intensity of the blue emission at 491 nm (3P0 → 3H4) and the red emission at 651 nm (3P0 → 3F2) drop dramatically, whereas the red emission at 619 nm (1D2 → 3H4) shows insignificant quenching (Fig. 3b–d). Significantly, the FIR of 1D2 → 3H4 and 3P0 → 3H4 (Red1/Blue), as well as 1D2 → 3H4 and 3P0 → 3F2 (Red1/Red2) could be used as detection signals for temperature monitoring. The FIR versus 1/T plots for SrZn0.33Nb0.67O3:1.25%
Pr3+ are presented in Fig. 4(a and b). It can be seen that the FIR for the two models changes clearly with increasing temperature. The FIR can be expressed as follows:36
| FIR ≈ C + A exp (−B/kBT) | (6) |
where
kB is the Boltzmann constant,
T is the temperature, and
A,
B and
C are constants. Since sensitivity is a very important working parameter for temperature sensors, in order to quantitatively assess the thermo-sensitive properties of the phosphor, the absolute temperature sensitivity (
Sa) and the relative temperature sensitivity (
Sr) were calculated using the following equations:
36 | 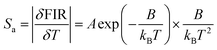 | (7) |
| 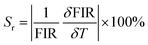 | (8) |
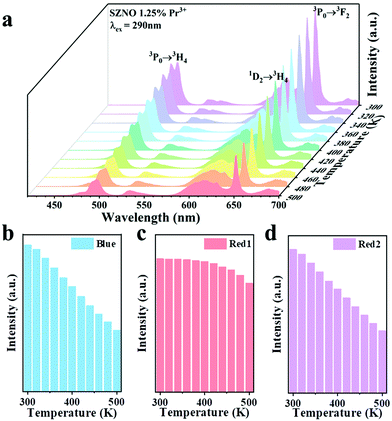 |
| Fig. 3 (a) Temperature-dependent PL spectra of the SrZn0.33Nb0.67O3:1.25% Pr3+ sample, recorded from 300 K to 500 K. (b), (c) and (d) Histograms displaying the luminescence intensity of the 491 nm, 619 nm and 651 nm peaks at different temperatures for the SrZn0.33Nb0.67O3:1.25% Pr3+ sample. | |
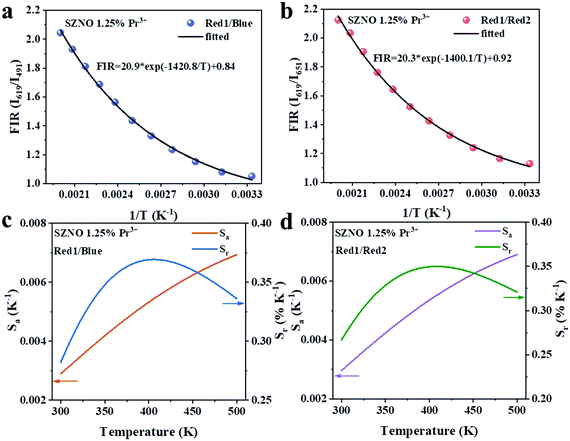 |
| Fig. 4 Experimentally measured and eqn (6)-fitted plots of (a) FIR (I619/I491) and (b) FIR (I619/I651) versus temperature. The measured and calculated plots of Sa and Srversus temperature based on (c) FIR (I619/I491) and (d) FIR (I619/I651) models. | |
The relationship between T and the absolute sensitivity Sa as well as the relative sensitivity Sr are shown in Fig. 4(c and d). The maximum values of Sa and Sr for SrZn0.33Nb0.67O3:1.25%
Pr3+ were calculated to be 0.007 K−1 (500 K) and 0.37% K−1 (400 K), respectively. The above results demonstrate that the as-prepared phosphor is a new potential thermo-sensitive material.
To further improve the temperature-sensing characteristics of SrZn0.33Nb0.67O3:1.25%
Pr3+,y%
Ga3+ (y = 4/6/8) ions were incorporated into the SrZn0.33Nb0.67O3 matrix. Fig. 5(a and c) shows the PL and PLE spectra of SrZn0.33Nb0.67O3:1.25%
Pr3+,6%
Ga3+ (the PL and PLE spectra for Ga3+-ion-doping concentrations of 4% and 8% are presented in Fig. S2, ESI†). The emission peaks are similar to those of SrZn0.33Nb0.67O3:1.25%
Pr3+ while the excitation bands shift towards a low energy for the 6% Ga3+-doped sample monitored at 491 nm, 619 nm and 651 nm. The smaller band gap determined via the diffuse reflectance spectrum for SrZn0.33Nb0.67O3:6%
Ga3+,1.25%
Pr3+ in Fig. 5(d) corroborates the changes mentioned above. The band gaps are reduced as well when different concentrations of Ga3+ ions were introduced into the matrix (Fig. S3, ESI†). In addition, the fluorescence intensity ratio of red light (1D2 → 3H4) and blue light (3P0 → 3H4) increased after Ga3+ doping, as demonstrated in Fig. 5(a), and to further understand this, the excited dynamics of the 1D2 and 3P0 energy levels were also investigated. Fig. 5(b) shows the fluorescence decay curves of SrZn0.33Nb0.67O3:6%
Ga3+,1.25%
Pr3+. The calculated fluorescence decay lifetime for the 1D2 energy level is 23.96 μs (619 nm, 1D2 → 3H4), which is longer than that measured in SrZn0.33Nb0.67O3:1.25%
Pr3+, while the fluorescence decay rate of the 3P0 state is accelerated slightly, resulting in a shorter decay time of the 3P0 energy level. These results suggest that Ga3+ doping can enhance the non-radiative transition possibility of the 3P0 state. At ambient temperature, the effect of multiphonon relaxation (MPR) is negligible. Therefore, the decay deviation could be mainly caused by cross-relaxation (CR) [3P0, 3H4] → [1D2, 3H6] and crossover to IVCT.
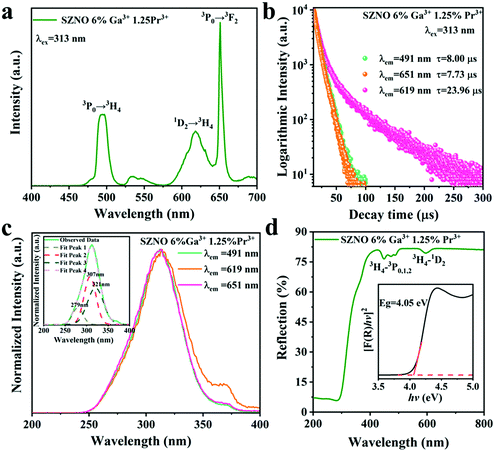 |
| Fig. 5 (a) PL spectra, (b) fluorescence decay curves, (c) PLE spectra (λem = 491 nm, 619 nm, 651 nm) and (d) the UV-vis DRS of the SrZn0.33Nb0.67O3:6% Ga3+,1.25% Pr3+, where the inset in (c) shows the Gaussian fitting performed on the excitation spectra, the inset in (d) shows the relationship of [F(R)hν]2versus energy hν. | |
Subsequently, the temperature-dependent spectra of the phosphors with different Ga3+ doping concentrations (4%, 6%, and 8%) were investigated. The temperature-dependent PL spectra of SrZn0.33Nb0.67O3:y%
Ga3+,1.25%
Pr3+ (y = 4/6/8) are shown in Fig. 6(a, c and d). It is demonstrated in Fig. 6(b) that the intensity of the 3P0 → 3H4 and 3P0 → 3H2 emissions drop more with increasing temperature after co-doping with Ga3+ ions. As a consequence, compared with SrZn0.33Nb0.67O3:1.25%
Pr3+, the thermal response of Red1/Blue and Red1/Red2 for the Ga3+ co-doped samples behave more dramatically. With the increase in temperature, the FIR value of Red1/Blue varies over 1.13–2.04 for SrZn0.33Nb0.67O3:1.25%
Pr3+, while it is 1.36–4.23 for the 6% Ga3+-doped sample. Similarly, the FIR value of Red1/Red2 varies over 1.13–2.13 for SrZn0.33Nb0.67O3:1.25%
Pr3+, while it is 1.57–4.14 for the 6% Ga3+-doped sample (Fig. S4, ESI†).
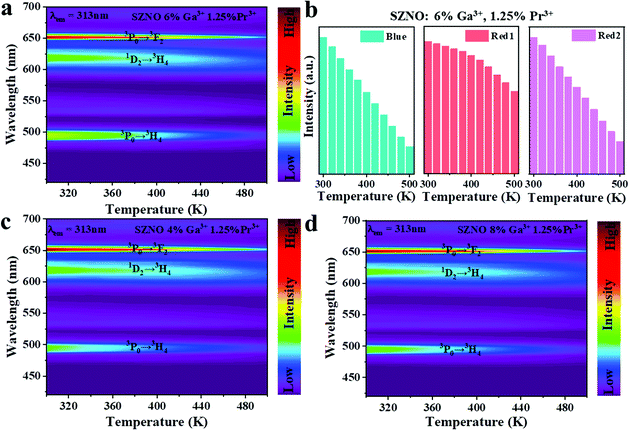 |
| Fig. 6 (a) Temperature-dependent PL spectra of the SrZn0.33Nb0.67O3:6% Ga3+,1.25% Pr3+, recorded from 300 K to 500 K. (b) Histograms displaying the luminescence intensity of the 491 nm (Blue), 619 nm (Red1) and 651 nm (Red2) peaks at different temperatures for the SrZn0.33Nb0.67O3:6% Ga3+,1.25% Pr3+. (c) Temperature dependent PL spectra of the SrZn0.33Nb0.67O3:4% Ga3+,1.25% Pr3+ and (d) SrZn0.33Nb0.67O3:8% Ga3+,1.25% Pr3+, recorded from 300 K to 500 K. | |
The absolute sensitivity Sa and the relative sensitivity Sr curves calculated using eqn (5) and (6) for the Ga3+,Pr3+ co-doped SrZn0.33Nb0.67O3 phosphors are shown in Fig. 7, and the maximum Sa and Sr values are also shown in Table 1. Clearly, in comparison with the Pr3+ single-doped SrZn0.33Nb0.67O3 phosphor, Sa and Sr are both significantly increased to some degree for the 4%, 6% and 8% Ga3+ co-doped phosphors. Remarkably, the maximal Sa and Sr values reach as high as 0.035 K−1 (500K) and 0.83% K−1 (500 K) for the 6% Ga3+ co-doped sample, which are increased by five- and two-fold, respectively, compared with the undoped sample. All these demonstrate that co-doping with Ga3+ ions effectively improves the performance of the SrZn0.33Nb0.67O3:Pr3+ thermo-sensitive phosphor.
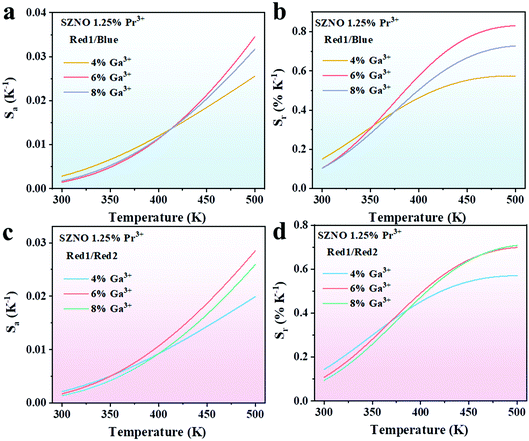 |
| Fig. 7 (a) Absolute sensitivity Sa and (b) relative sensitivity Srversus temperature for the Red1/Blue model for SrZn0.33Nb0.67O3:y% Ga3+,1.25% Pr3+ (y = 4/6/8). (c) Absolute sensitivity Sa and (d) relative sensitivity Srversus temperature for the Red1/Red2 model for SrZn0.33Nb0.67O3:y% Ga3+,1.25% Pr3+ (y = 4/6/8). | |
Table 1 Calculated maximum Sa and Sr values of SrZn0.33Nb0.67O3:y%
Ga3+,1.25%
Pr3+ (y = 4/6/8)
Transition |
Material |
Temperature range (K) |
S
a-max (K−1) |
S
r-max (% K−1) |
Pr3+: 1D2 → 3H4, 3P0 → 3H4 |
SrZn0.33Nb0.67O3 |
300–500 |
0.007 (500 K) |
0.37 (400 K) |
SrZn0.33Nb0.67O3:4% Ga3+ |
300–500 |
0.026 (500 K) |
0.58 (500 K) |
SrZn0.33Nb0.67O3:6% Ga3+ |
300–500 |
0.035 (500 K) |
0.83 (500 K) |
SrZn0.33Nb0.67O3:8% Ga3+ |
300–500 |
0.032 (500 K) |
0.73 (500 K) |
Pr3+: 1D2 → 3H4, 3P0 → 3F2 |
SrZn0.33Nb0.67O3 |
300–500 |
0.007 (500 K) |
0.35 (400 K) |
SrZn0.33Nb0.67O3:4% Ga3+ |
300–500 |
0.020 (500 K) |
0.57 (500 K) |
SrZn0.33Nb0.67O3:6% Ga3+ |
300–500 |
0.029 (500 K) |
0.70 (500 K) |
SrZn0.33Nb0.67O3:8% Ga3+ |
300–500 |
0.026 (500 K) |
0.70 (500 K) |
According to the above results, the markedly improved temperature-sensing performance is caused directly by the more significant thermal quenching of the 3P0 state after Ga3+ co-doping. To further understand the fluorescence quenching dynamics, the possible depopulation process for the 3P0 state was discussed. As observed in Fig. 8, three de-excitation modes may contribute to the thermal quenching of the 3P0-related luminescence, namely, phonon-assisted cross-relaxation(CR), multiphonon relaxation (MPR) and crossover to a low-lying IVCT state. Considering that the CR processes of [3P0, 3H4]
[1G4, 1G4] and [3P0, 3H4]
[1D2, 3H6] are non-resonant, whereas the [1D2, 3H4]
[1G4, 3F4] is resonant, as a consequence, the probability of the phonon-involved former CR processes is more significantly accelerated by elevating the temperature than that of the latter. However, the MPR process has less impact on the depopulation probability of the 3P0 state in niobate due to the large energy gap (ΔE ≈ 3500 cm−1) between the 3P0 and 1D2 states according to previous studies.21 Notably, the low-lying Pr3+–Nb5+ IVCT state is regarded as one of the most important depopulation pathways for the 3P0 level in SrZn0.33Nb0.67O3. As illustrated in in Fig. 8(b), both of the 3P0 and 1D2 emissions can be de-excited from Pr3+–Nb5+ IVCT with phonons assisting. However, the electrons in the 1D2 state have a higher cross-over energy than in 3P0 state, thus leading to the diverse thermal response of the 3P0 and 1D2 emissions. The empirical formula for estimating the IVCT energy in Pr3+-doped niobite is as follows:37
|  | (9) |
where
χopt(Nb
5+) represents the electronegativity of Nb
5+, and
d(Pr
3+–Nb
5+) represents the shortest distance between Pr
3+ and Nb
5+ in the host. When Ga
3+ ions are incorporated, the unit cell of SrZn
0.33Nb
0.67O
3 shrinks, as confirmed by the XRD results in
Fig. 1(a), which may reduce the distance between Pr
3+ and Nb
5+, consequently resulting in a lower-lying Pr
3+–Nb
5+ IVCT state. Therefore, the energy barrier Δ
E′ for the
3P
0 state in Ga
3+ co-doped SrZn
0.33Nb
0.67O
3:Pr
3+ is smaller than Δ
E in SrZn
0.33Nb
0.67O
3:Pr
3+. This explains why the doping of Ga
3+ accelerates the thermal quenching of the blue emission (
3P
0 →
3H
4) and the red emission (
3P
0 →
3F
2). Since the crossover energy from
1D
2 to the Pr
3+–Nb
5+ IVCT state is much larger, a small quantity of Ga
3+ doping has minor influence on the
1D
2 emission. Moreover, some of the electrons depopulated from the
3P
0 level may relax to the
1D
2 level through the Pr
3+–Nb
5+ IVCT bridge. Thus, all these factors together promote the temperature-sensing performance of the designed luminescent thermometer.
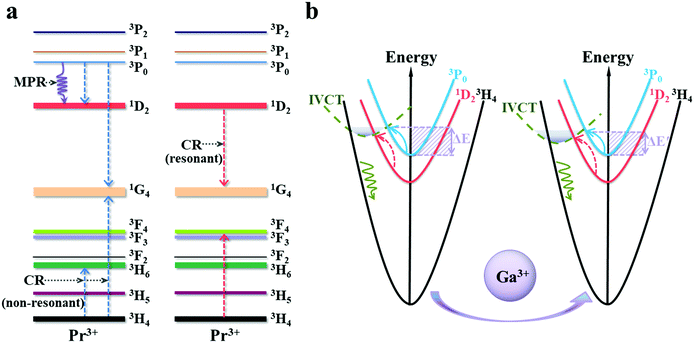 |
| Fig. 8 (a) Schematic energy-transfer diagram for the cross-relaxation and multiphonon relaxation processes of Pr3+. (b) Configurational coordinate diagrams showing the temperature increase-induced non-radiative relaxation processes of Pr3+ in SrZn0.33Nb0.67O3 and SrZn0.33Nb0.67O3:Ga3+. | |
It is known that the relative sensitivity Sr is a critical working parameter since it allows the standardization of diverse luminescence-based temperature sensors regardless of the difference in intuitive observations.1 To further evaluate the temperature-sensing performance of the Ga3+,Pr3+ co-doped SrZn0.33Nb0.67O3 phosphor, typical Pr3+-activated niobate, zirconate and germanate thermometric phosphors14,15,21,35–37 reported in recent years are displayed in Table 2 for comparison. As illustrated in the table, the SrZn0.33Nb0.67O3:Pr3+,6%
Ga3+ in this work has a higher Sr than the zirconate and germanate thermometric phosphors. Moreover, except for LaMg0.402Nb0.598O3:Pr3+, there are few investigations on temperature sensitivities based on two-FIR models. Clearly, compared with LaMg0.402Nb0.598O3:Pr3+, the SrZn0.33Nb0.67O3:Pr3+,6%
Ga3+ phosphor has made some progress to a certain degree. The analysis above indicates that the obtained SrZn0.33Nb0.67O3:Pr3+,Ga3+ phosphor could be employed as a promising candidate for self-calibrating temperature measurement.
Table 2 Maximum relative sensitivities of representative Pr3+-activated optical temperature-sensing materials in previous reports
Transition |
Material |
Temperature range (K) |
S
r-max (% K−1) |
Ref. |
Pr3+: 1D2 → 3H4, 3P0 → 3H4 |
YNbO4 |
303–543 |
1.56 (503 K) |
17
|
Sr2(Ge0.75Si0.25)O4 |
275–650 |
0.30 (460 K) |
18
|
LaMg0.402Nb0.598O3 |
298–533 |
0.71 (473 K) |
24
|
GdNbO4 |
300–700 |
0.70 (430 K) |
38
|
Sr2GeO4 |
300–600 |
0.60 (300 K) |
39
|
La0.4Gd1.6Zr2O7 |
15–650 |
0.81 (650 K) |
40
|
SrZn0.33Nb0.67O3:6% Ga3+ |
300–500 |
0.83 (500 K) |
This work |
Pr3+: 1D2 → 3H4, 3P0 → 3F2 |
LaMg0.402Nb0.598O3 |
298–533 |
0.73 (473 K) |
24
|
SrZn0.33Nb0.67O3:6% Ga3+ |
300–500 |
0.70 (500 K) |
This work |
Conclusion
In summary, SrZn0.33Nb0.67O3 phosphors doped with Pr3+,Ga3+ were successfully synthetized via a high-temperature solid-state reaction route, and the temperature-dependent luminescence of Pr3+ is investigated for the first time. Under ultraviolet excitation, the SrZn0.33Nb0.67O3:x%Pr (x = 0.25/0.75/1.25/2) phosphors exhibit bright blue and red emissions, which are located at 491 nm, 619 nm and 651 nm. Remarkably, the temperature sensitivities of the FIR thermometer are significantly enhanced when Ga3+ is incorporated. Specifically, the maximum absolute and relative sensitivities for the 6% Ga3+ co-doped sample are increased five- and two-fold, respectively, compared with the undoped sample. Analysis of the configurational coordinate diagram indicated that the changed thermo-sensitive properties are ascribed to the intervalence charge transfer state interfered Pr3+ luminescence. The results suggest the potential application of the SrZn0.33Nb0.67O3:Pr3+,Ga3+ phosphor in optical thermometry. In addition, this study offers new thoughts for improving the performance of FIR-based luminescent thermometers.
Conflicts of interest
There are no conflicts to declare.
Acknowledgements
This work was supported by the National Natural Science Foundation of China (52102186), the Guangdong Basic and Applied Basic Research Foundation (2019A1515110801), the Science Foundation for High-level Talents of Wuyi University (2018AL016), the Wuyi University–Macau University Joint Research Fund (2019WGALH08), and the Special projects in key fields of Guangdong Universities (2021ZDZX1022).
References
- C. Bradac, S. F. Lim, H. C. Chang and I. Aharonovich, Optical Nanoscale Thermometry: From Fundamental Mechanisms to Emerging Practical Applications, Adv. Opt. Mater., 2020, 8, 2000183 CrossRef CAS.
- X. D. Wang, O. S. Wolfbeis and R. J. Meier, Luminescent probes and sensors for temperature, Chem. Soc. Rev., 2013, 42, 7834–7869 RSC.
- D. Parker, J. D. Fradgley and K. L. Wong, The design of responsive luminescent lanthanide probes and sensors, Chem. Soc. Rev., 2021, 50, 8193–8213 RSC.
- Z. L. Ji, Y. Cheng, X. S. Cui, H. Lin, J. Xu and Y. S. Wang, Heating-induced abnormal increase in Yb3+ excited state lifetime and its potential application in lifetime luminescence nanothermometry, Inorg. Chem. Front., 2019, 6, 110–116 RSC.
- F. Jahanbazi and Y. B. Mao, Recent advances on metal oxide-based luminescence thermometry, J. Mater. Chem. C, 2021, 9, 16410–16439 RSC.
- Y. Y. Tu, S. L. Zhao, D. Y. He, T. Wu, H. Zhang, R. S. Lei, L. H. Huang and S. Q. Xu, A portable all-fiber thermometer based on the fluorescence intensity ratio (FIR) technique in rare earth doped TeO2–WO3–La2O3–Na2O glass, J. Mater. Chem. C, 2018, 6, 7063–7069 RSC.
- S. Senapati and K. K. Nanda, Red emitting Eu:ZnO nanorods for highly sensitive fluorescence intensity ratio based optical thermometry, J. Mater. Chem. C, 2017, 5, 1074–1082 RSC.
- J. Zhang, J. J. Chen and Y. I. Zhang, Temperature-sensing luminescent materials La9.67Si6O26.5:Yb3+–Er3+/Ho3+ based on pump-power-dependent upconversion luminescence, Inorg. Chem. Front., 2020, 7, 4892–4901 RSC.
- Z. Sun, M. C. Jia, Y. L. Wei, J. C. Cheng, T. Q. Sheng and Z. L. Fu, Constructing new thermally coupled levels based on different emitting centers for high sensitive optical thermometer, Chem. Eng. J., 2020, 381, 122654 CrossRef CAS.
- G. T. Xiang, Q. Xia, X. T. Liu and X. J. Wang, Optical thermometry based on the thermally coupled energy levels of Er3+ in the upconversion materials, Dalton Trans., 2020, 49, 17115–17120 RSC.
- H. Zhang, J. T. Ye, X. L. Wang, S. L. Zhao, R. S. Lei, L. H. Huang and S. Q. Xu, Highly reliable all-fiber temperature sensor based on the fluorescence intensity ratio (FIR) technique in Er3+/Yb3+ co-doped NaYF4 phosphors, J. Mater. Chem. C, 2019, 7, 15269–15275 RSC.
- P. Du, X. Y. Huang and J. S. Yu, Yb3+-Concentration dependent upconversion luminescence and temperature sensing behavior in Yb3+/Er3+ codoped Gd2MoO6 nanocrystals prepared by a facile citric-assisted sol–gel method, Inorg. Chem. Front., 2017, 4, 1987–1995 RSC.
- T. Hu, Y. Gao, M. Molokeev, Z. G. Xia and Q. Y. Zhang, Non-stoichiometry in Ca2Al2SiO7 enabling mixed-valent europium toward ratiometric temperature sensing, Sci. China Mater., 2019, 62, 1807–1814 CrossRef CAS.
- N. Z. Zhang, D. W. Zhang, J. Zhao and Z. G. Xia, Fabrication of dual-emitting dye-encapsulated metal–organic framework as a stable fluorescent sensor for metal ions detection, Dalton Trans., 2019, 48, 6794–6799 RSC.
- Y. Zhou, D. N. Zhang, J. Zeng, N. Gan and J. Cuan, A luminescent Lanthanide-free MOF nanohybrid for highly sensitive ratiometric temperature sensing in physiological range, Talanta, 2018, 181, 410–415 CrossRef CAS PubMed.
- Q. Wang, M. Liao, Q. M. Lin, M. X. Xiong, X. Zhang, H. F. Dong, Z. P. Lin, M. R. Wen, D. Y. Zhu, Z. F. Mu and F. G. Wu, The design of dual-switch fluorescence intensity ratio thermometry with high sensitivity and thermochromism based on a combination strategy of intervalence charge transfer and up-conversion fluorescence thermal enhancement, Dalton Trans., 2021, 50, 9298–9309 RSC.
- Y. Gao, F. Huang, H. Lin, J. Xu and Y. S. Wang, Intervalence charge transfer state interfered Pr3+ luminescence: A novel strategy for high sensitive optical thermometry, Sens. Actuators, B, 2017, 243, 137–143 CrossRef CAS.
- M. Sójka, J. F. C. B. Ramalho, C. D. S. Brites, K. Fiaczyk, L. D. Carlos and E. Zych, Bandgap Engineering and Excitation Energy Alteration to Manage Luminescence Thermometer Performance. The Case of Sr2(Ge,Si)O4:Pr3+, Adv. Opt. Mater., 2019, 7, 1901102 CrossRef.
- M. Sójka, C. D. S. Brites, L. D. Carlos and E. Zych, Exploiting bandgap engineering to finely control dual-mode Lu2(Ge,Si)O5:Pr3+ luminescence thermometers, J. Mater. Chem. C, 2020, 8, 10086–10097 RSC.
- Y. Gao, F. Huang, H. Lin, J. G. Zhou, J. Xu and Y. S. Wang, A Novel Optical Thermometry Strategy Based on Diverse Thermal Response from Two Intervalence Charge Transfer States, Adv. Funct. Mater., 2016, 26, 3139–3145 CrossRef CAS.
- W. Tang, Y. Sun, S. C. Wang, B. S. Du, Y. Q. Yin, X. Liu, B. Yang, W. W. Cao and M. Yu, Pr3+-Doped (K0.5Na0.5)NbO3 as a high response optical oxygen sensing agent, J. Mater. Chem. C, 2016, 4, 11508–11513 RSC.
- R. Shi, L. T. Lin, P. Dorenbos and H. B. Liang, Development of a potential optical thermometric material through photoluminescence of Pr3+ in La2MgTiO6, J. Mater. Chem. C, 2017, 5, 10737–10745 RSC.
- Y. J. Wang, V. Tsiumra, Q. Peng, H. B. Liang, Y. Zhydachevskyy, M. Chaika, P. Dluzewski, H. Przybylinska and A. Suchocki, Hole Trapping Process and Highly Sensitive Ratiometric Thermometry over a Wide Temperature Range in Pr3+-Doped Na2La2Ti3O10 Layered Perovskite Microcrystals, J. Phys. Chem. A, 2019, 123, 4021–4033 CrossRef CAS PubMed.
- H. Zhang, Z. Gao, G. G. Li, Y. L. Zhu, S. Q. Liu, K. Li and Y. J. Liang, A ratiometric optical thermometer with multi-color emission and high sensitivity based on double perovskite LaMg0.402Nb0.598O3: Pr3+ thermochromic phosphors, Chem. Eng. J., 2020, 380, 122491 CrossRef CAS.
- X. H. Li, L. Zhou, M. D. Dramićanin, Q. Tang, X. P. Jing, J. X. Shi, Y. Q. Xu and M. M. Wu, Broad-band emission of A3B′B′′2O9 complex perovskites (A = Ba, Sr; B′ = Zn; B′′ = Ta, Nb) realized by structural variations of the B site order–disorder, J. Mater. Chem. C, 2018, 6, 12566–12574 RSC.
- W. Y. Li, L. X. Ning and P. A. Tanner, Double perovskite structure: a vibrational and luminescence investigation providing a perspective on crystal field strength, J. Phys. Chem. A, 2012, 116, 7337–7344 CrossRef CAS PubMed.
- A. F. Fuentes, O. Hernández-Ibarra, G. Mendoza-Suarez, J. I. Escalante-Garcıa, K. Boulahya and U. Amador, Structural analysis of several W(VI) and Mo(VI) complex perovskites prepared by the polymeric precursors method, J. Solid State Chem., 2003, 173, 319–327 CrossRef CAS.
- S. Sinha, M. K. Mahata, H. C. Swart, A. Kumar and K. Kumar, Enhancement of upconversion, temperature sensing and cathodoluminescence in the K+/Na+ compensated CaMoO4:Er3+/Yb3+ nanophosphor, New J. Chem., 2017, 41, 5362–5372 RSC.
- N. An, H. L. Zhou, K. S. Zhu, L. H. Ye, J. R. Qiu and L.-G. Wang, Improved temperature sensing performance of YAG:Ho3+/Yb3+ by doping Ce3+ ions based on up-conversion luminescence, J. Alloys Compd., 2020, 843, 156057 CrossRef CAS.
- M. Wang, R. Wang, Y. Li, N. Lin and Y. Xu, The enhancement mechanism of Yb3+/Tm3+/12CaO·7Al2O3via Li+ incorporation and temperature sensing applications, Mate. Today Chem., 2019, 14, 100187 CrossRef CAS.
- M. L. Zhang, X. S. Zhai, P. P. Lei, S. Yao, X. Xu, L. L. Dong, K. M. Du, C. Y. Li, J. Feng and H. J. Zhang, Selective enhancement of green upconversion luminescence from NaYF4:Yb, Er microparticles through Ga3+ doping for sensitive temperature sensing, J. Lumin., 2019, 215, 116632 CrossRef CAS.
- L. Kong, Y. Y. Liu, L. P. Dong, L. Zhang, L. Qiao, W. S. Wang and H. P. You, Enhanced red luminescence in CaAl12O19:Mn4+ via doping Ga3+ for plant growth lighting, Dalton Trans., 2020, 49, 1947–1954 RSC.
- W. Yan, S. G. Xiao and X. L. Yang, Novel red-emitting phosphor Li2MgZrO4:Mn4+, Ga3+ for warm white LEDs based on blue-emitting chip, RSC Adv., 2019, 9, 5354–5361 RSC.
- X. A. Chen, Y. An and W. Q. Xiao, A new solid solution Bi1.48Eu0.52Pb0.5Sr0.5B2O7 and luminescent properties of the Bi2-xPb0.5Sr0.5B2O7:xEu3+ phosphors, J. Lumin., 2021, 237, 118137 CrossRef CAS.
- Y. Gao, Y. Cheng, T. Hu, Z. L. Ji, H. Lin, J. Xu and Y. S. Wang, Broadening the valid temperature range of optical thermometry through dual-mode design, J. Mater. Chem. C, 2018, 6, 11178–11183 RSC.
- S. X. Wang, S. W. Ma, J. M. Wu, Z. M. Ye and X. Cheng, A promising temperature sensing strategy based on highly sensitive Pr3+-doped SrRE2O4 (RE = Sc, Lu and Y) luminescent thermometers, Chem. Eng. J., 2020, 393, 124564 CrossRef CAS.
- C. M. Liu, F. J. Pan, Q. Peng, W. J. Zhou, R. Shi, L. Zhou, J. H. Zhang, J. Chen and H. B. Liang, Excitation Wavelength Dependent Luminescence of LuNbO4:Pr3+—Influences of Intervalence Charge Transfer and Host Sensitization, J. Phys. Chem. C, 2016, 120, 26044–26053 CrossRef CAS.
- W. G. Ye, C. Y. Ma, Y. B. Li, C. Zhao, Y. Z. Wang, Y. Z. Zuo, C. D. Zou, Z. C. Wen, Y. K. Li, X. Y. Yuan and Y. G. Cao, Anti-Thermal-Quenching Red-Emitting GdNbO4:Pr3+ Phosphors Based on Metal-to-Metal Charge Transfer for Optical Thermometry Application, J. Mater. Chem. C, 2021, 42, 15201–15211 RSC.
- C. D. S. Brites, K. Fiaczyk, J. F. C. B. Ramalho, M. Sójka, L. D. Carlos and E. Zych, Widening the Temperature Range of Luminescent Thermometers through the Intra- and Interconfigurational Transitions of Pr3+, Adv. Opt. Mater., 2018, 6, 1701318 CrossRef.
- J. Trojan-Piegza, C. D. S. Brites, J. F. C. B. Ramalho, Z. J. Wang, G. H. Zhou, S. W. Wang, L. D. Carlos and E. Zych, La0.4Gd1.6Zr2O7:0.1%Pr transparent sintered ceramic – a wide-range luminescence thermometer, J. Mater. Chem. C, 2020, 8, 7005–7011 RSC.
Footnote |
† Electronic supplementary information (ESI) available. See DOI: 10.1039/d1ma01247a |
|
This journal is © The Royal Society of Chemistry 2022 |