DOI:
10.1039/D1MA01246K
(Paper)
Mater. Adv., 2022,
3, 3143-3150
Failure mechanism of solid-state electrolyte Li10GeP2S12 in a moist atmosphere: a first-principles study
Received
27th December 2021
, Accepted 2nd February 2022
First published on 3rd February 2022
Abstract
All-solid-state lithium-ion batteries (ASSLIBs) have commercial potential for industrial applications in long-range electric vehicles. The chemical properties of solid-sate electrolytes (SSEs) play a key role in the performance of ASSLIBs. Among all kinds of SSEs, sulfide solid-sate electrolytes are advantageous to ASSLIBs due to their high ionic conductivity and excellent electrochemical stability. However, sulfide electrolytes have poor stability in air due to their propensity to react with H2O to generate toxic H2S gas. Herein to understand the specific failure mechanism, we use first-principles calculations to study the kinetic and thermodynamic mechanism of the reaction between Li10GeP2S12, which is a typical sulfide electrolyte, and H2O in the atmosphere. We find that the H2O molecules preferentially react with the sulfur atoms of the PS4 tetrahedra to produce H2S. As the sulfur atoms of the PS4 tetrahedra in the bulk continuously emerge towards the surface, this reaction occurs repeatedly. Meanwhile, the oxygen atoms from H2O molecules can also diffuse into the bulk. These reactions continue until the sulfur atoms of the PS4 tetrahedra in Li10GeP2S12 are completely replaced by oxygen atoms. Furthermore, we study the influence of Sb doping on Li10GeP2S12, and kinetically explain the mechanism of hydrolysis inhibition when doping Sb in Li10GeP2S12.
Introduction
With the growing demand for low-carbon technologies, lithium-ion batteries with a higher energy density, longer cycle life and better safety have become much more desirable to compete with gasoline vehicles. Traditional commercial lithium-ion batteries with a liquid organic electrolyte have the advantages of high theoretical specific capacity, environmental friendliness, etc.1–5 However, the organic electrolyte in lithium-ion batteries is flammable, and has a lower thermal stability and lower safety compared with inorganic solid-state electrolytes.6–8 The solid-state electrolytes used in solid-state lithium-ion batteries consist mainly of polymers,9,10 oxides11–13 and sulfides.14,15 Among them, sulfides have a relatively high ionic conductivity, and some are comparable to organic liquid electrolytes.16 However, sulfide solid-state electrolytes react easily with H2O in moist air to generate toxic H2S gas, which limits their practical applications. A great deal of experimental work has been done to solve the air-stability problem. Muramatsu et al.,17 have studied the structural changes of Li2S–P2S5 glass structures in the atmosphere. This study found that PS43− can reduce H2S gas for binary Li2S–P2S5 glass or glass ceramics. 75Li2S–25P2S5 has been found to have better water stability. Tin sulfides (Li4SnS4,18,19 and Li4SnS4-LiI20), As-substituted Li4SnS4,21 and Sb-substituted Li4SnS422,23 also show better stability in air. Based on the hard and soft acids and bases (HSAB) theory,24 P is a hard acid, Sn, Sb, and As are soft acids, and S is a soft base, Thus, the Sn–, Sb–, and As–S bonds are harder than the P–S bond. Hayashi et al.25 inhibited the production of H2S by doping MxOy (M = Fe, Zn, and Bi) in the Li3PS4 electrolyte, which can absorb H2S. Although metal oxide doping improves the air stability of sulfide solid-state electrolytes, it also reduces the ionic conductivity. In addition, Sun et al.26 improved the air stability of the sulfide solid-state electrolyte through introducing soft acid metals. The air stability is improved via Sb doping of Li10GeP2S12, where the Li10Ge(P1−xSbx)2S12 ionic conductivity is 17.3 ± 0.9 mS cm−1. After air exposure, the ionic conductivity can remain between 12.1 and 15.7 mS cm−1.
Kamaya et al. reported a superionic conductor Li10GeP2S12 with high ionic conductivity (12 mS cm−1) at room temperature.27 Compared with most sulfide solid-state electrolytes, Li10GeP2S12 has been more extensively studied. Its space group is P42/nmc, and consists of (Ge0.5P0.5)S4 tetrahedra, PS4 tetrahedra, LiS4 tetrahedra and LiS6 octahedra. It has three-dimensional lithium-ion channels.27–29 The diffusion paths along the c direction are considered as the dominant transport channel with the activation barrier of 0.16 eV, which is much lower than the diffusion barrier in the ab plane (0.26 eV).30 In addition, Li10GeP2S12 also has a wide electrochemical stability window (>5 V against Li/Li+) at room temperature. Mo et al.29 proposed that this is due to the decomposition of Li10GeP2S12 into Li2S and P2S5 passivation layers when Li10GeP2S12 comes into contact with the electrode, which then protects the solid-state electrolyte, resulting in a wide electrochemical window. However, as with other sulfide compounds, Li10GeP2S12 is sensitive to atmospheric moisture, which can react with H2O to produce the air pollutant H2S,31,32 and lead to a decreased ionic conductivity for Li10GeP2S12. These drawbacks limit the practical applications of sulfide solid electrolytes in industry. Although a lot of research into Li10GeP2S12 air stability has been carried out experimentally, few theoretical studies have been performed to explore the Li10GeP2S12 air stability. In this work, we systematically study the specific chemical reactions between Li10GeP2S12 and H2O in air based on first-principles calculations, and explore the failure mechanism of the solid-state electrolyte in a moist atmosphere.
Computational details
All calculations are performed based on density functional theory (DFT) using the generalized gradient approximation (GGA) with the Perdew–Burke–Ernzerhof (PBE) functional.33 The interactions between ion cores and valence electrons are treated using the projector augmented wave (PAW)34,35 method as implemented in the Vienna ab initio simulation package (VASP).36 The kinetic energy cutoff is set as 450 eV. In addition, the climbing image nudged elastic band (CI-NEB) method37 is used to obtain the specific chemical reaction paths. A 4 × 4 × 1 Monkhorst–Pack k-point grid is used to calculate the reaction coordinates and surface energies. All structures are fully relaxed until the residual forces are less than 0.01 eV Å−1 with the spin-polarized calculations. The crystal structure of Li10GeP2S12 is shown in Fig. 1. The calculated lattice constants are a = b = 8.74 Å, and c = 12.86 Å, which are consistent with an earlier report.38
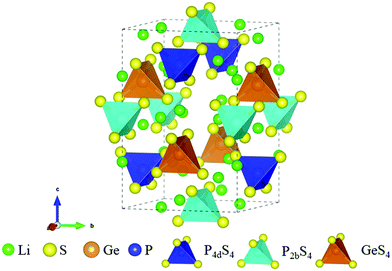 |
| Fig. 1 Crystal structure of Li10GeP2S12. | |
Results and discussion
In order to find the dominant surface of Li10GeP2S12, we first obtain the surface energies (ES) of various surfaces, which are calculated using eqn (1), | 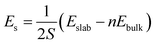 | (1) |
where S is the surface area, Eslab is the total energy of the surface structure, n is the number of unit cells, and Ebulk is the total energy of bulk Li10GeP2S12. As listed in Table 1, we find that the most stable surface is the (110) surface, which has lowest energy of 0.029 eV Å−2. According to the results of the surface energies, the (001) and (110) surfaces are more likely to be exposed to the air. However, we find that there are both PS4 tetrahedra and GeS4 tetrahedra on the exposed (110) surface (see Fig. 2), which is more representative.
Table 1 Surface energy (ES) values of Li10GeP2S12
Surface |
E
S (eV Å−2) |
(001) |
0.033 |
(010) |
0.075 |
(100) |
0.059 |
(110) |
0.029 |
(011) |
0.055 |
(101) |
0.064 |
(111) |
0.040 |
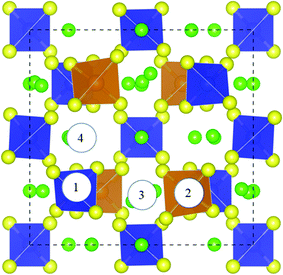 |
| Fig. 2 H2O adsorption site on the Li10GeP2S12(110) surface. Site ① is the adsorption site on the PS4 tetrahedron, site ② is the adsorption site on the GeS4 tetrahedron, and sites ③ and ④ are the adsorption sites on Li+. | |
As can be seen in Fig. 2, there are four possible sites that can be adsorption sites for H2O molecules: these are S sites on the PS4 tetrahedron (site ① in Fig. 2), S sites on the GeS4 tetrahedron (site ② in Fig. 2), and Li sites between the PS4 and GeS4 tetrahedra (sites ③ and ④ in Fig. 2). The adsorption energy (Eads) is calculated as viaeqn (2),
| Eads = Etot − EH2O − Esurface | (2) |
where the
Etot is the total energy of the H
2O-adsorbed surface,
EH2O is the energy of the H
2O molecule, and
Esurface is the total energy of the Li
10GeP
2S
12 slab. As listed in
Table 2, we find that, compared with the S atoms in PS
4 tetrahedra and the S atoms in GeS
4 tetrahedra, H
2O molecules are more likely to be adsorbed on Li
+. For site ③, the adsorption energy of H
2O is −0.43 eV. This is because O sp
3 hybridization in H
2O has an unshared electron pair, which can be combined with the vacant 2s orbital of the outermost layer of Li
+, thus forming the Li–O bond with greater adsorption energy.
Table 2 Adsorption energies of H2O at different sites on the (110) surface
Site |
① |
② |
③ |
④ |
E
ads (eV) |
0.15 |
−0.12 |
−0.43 |
−0.28 |
We now investigate the decomposition of H2O on the Li10GeP2S12(110) surface. According to the theoretical calculations of Mo et al.,39 the reaction between the Li10GeP2S12(110) surface and H2O is as follows:
| Li–PS4 + H2O → LiOH–PS3(SH) → Li–PS3O + H2S↑ | (3) |
| Li–GeS4 + H2O → LiOH–GeS3(SH) → Li–GeS3O + H2S↑ | (4) |
We show the reaction processes in Fig. 3. The adsorbed H2O molecules can be decomposed into H+ and OH− on the surface, where H+ reacts with the S atom on the PS4/GeS4 tetrahedra to form the H–S bond, and OH− can be further decomposed to form O2− and H+. The latter H+ reacts with S–H to form H2S, which is desorbed from the (110) surface.
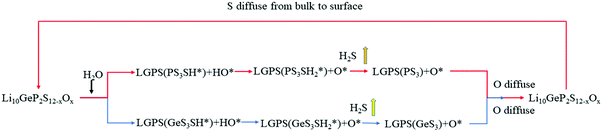 |
| Fig. 3 Reaction paths of Li10GeP2S12 surfaces and H2O, where the red path represents H2O reacting with S in the PS4 tetrahedra, and the blue one represents H2O reacting with S in the GeS4 tetrahedra. | |
Fig. 4(a and b) depict the energy profile of the hydrolysis reaction between H2O molecules and S of the PS4 tetrahedra and the relative intermediate reaction products. IS0 stands for the (110) surface with no H2O molecules adsorbed. IS1 stands for the (110) surface-adsorbed H2O molecules. TS1 stands for the transition state and MS1 stand for the intermediate state of H2O decomposed into H+ and OH−. MS2 stands for the second intermediate state, which relaxes the position of the H atom of OH−, and MS3 stands for the third intermediate state of OH− decomposition into H+ and O2−. FS stands for the final state of H2S desorbing from the Li10GeP2S12(110) surface. From the results of Table 2, H2O adsorbs on Li+ and the adsorption energy is −0.43 eV. For the process from IS1 to MS1, H2O decomposes into H+ and OH−, where H+ combines with S to form the H–S bond and the related energy barrier is 0.41 eV. OH− decomposes into H+ and O2− from MS2 to MS3, where H+ combines with the SH group in MS1 to generate H2S, which is adsorbed on the surface. Meanwhile, O2− combines with S of GeS4 to form the S–O bond, and the energy barrier for this is 0.65 eV. The process from MS3 to FS is the desorption of H2S from the surface, where the desorption energy is 0.27 eV. In addition, we also consider the similar reaction between H2O and the GeS4 tetrahedra on the Li10GeP2S12(110) surface, shown in Fig. 4(c and d). By comparing the energy profiles with different sulfur sources, we find that the reaction energy barrier of H2O decomposition on the GeS4 tetrahedra (0.80 eV, 0.72 eV) is higher than the reaction energy barrier on PS4 tetrahedra (0.41 eV, 0.65 eV). This suggests that H2O is more likely to react with S in the PS4 tetrahedra in Li10GeP2S12 to produce H2S. FS in Fig. 4(b and d) also shows the result of an adsorbed oxygen atom and a sulfur vacancy. Since the reaction on the PS4 tetrahedron is much easier than that on the GeS4 tetrahedron, we now study the existence of S vacancies on the PS4 tetrahedra.
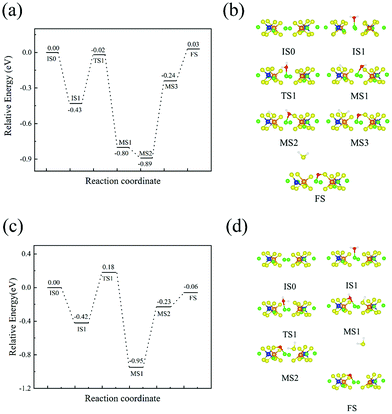 |
| Fig. 4 Reaction path profiles of H2O reactions on the Li10GeP2S12(110) surface. (a) H2O reaction energies related to the PS4 tetrahedra; (b) structural diagram of the minimum energy path for the H2O reaction with PS4 tetrahedra; (c) H2O reaction energy related to the GeS4 tetrahedra; and (d) structural diagram of the minimum energy path for the double H2O reaction with the GeS4 tetrahedra. (IS, initial state; TS, transition state; MS, meta-stable state; FS, final state). Color code: green, Li atoms; yellow, S atoms; blue, P atoms; orange, Ge atoms; red, O atoms; white, H atoms. | |
As experiments have shown that the degradation of Li10GeP2S12 releases a large amount of H2S,26 this process inevitably involves the sulfur atoms in the bulk. This means that S in Li10GeP2S12 will migrate to the surface. As shown in Fig. 5, three diffusion paths of the S atom are considered: S atom diffusion on the surface, S atom diffusion from a GeS4 tetrahedron to a PS4 tetrahedron, and S atom diffusion from a PS4 tetrahedron to a PS4 tetrahedron, and Fig. 5(a) shows the energy barriers of 0.45 eV, 1.07 eV and 0.82 eV, respectively. Fig. 5(b) shows the initial and final structures for the diffusion of an S atom on the (110) surface. We find the presence of the Li+ vacancy could be conducive to the diffusion of the S atom. This is because when the S atom diffuses from one tetrahedron to another tetrahedron, it could use the Li+ channel along the c direction. Fig. 5(c and d) show the S atom diffusion path from a PS4 tetrahedron to a PS4 tetrahedron and from a GeS4 tetrahedron to a PS4 tetrahedron, respectively. The diffusion barriers shows that the diffusion of the S atom from a PS4 tetrahedron to a PS4 tetrahedron is relatively facile. This is also consistent with HSAB theory, where the bond of the soft base S and soft acid Ge is stronger than the bond of the soft base S and the hard acid P, and the bond energy of Ge–S is higher than that of P–S.
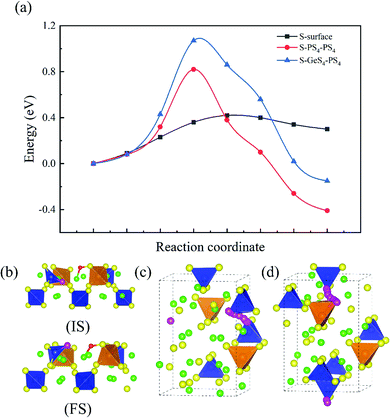 |
| Fig. 5 Minimum energy paths for sulfur diffusion. (a) Energy profiles of S atom diffusion. (b) Structural diagram of S atom diffusion on the surface (IS, initial state; FS, final state). (c) Structural diagram of S atom diffusion in the bulk from a PS4 tetrahedron to a PS4 tetrahedron. (d) Diagram of S atom diffusion in the bulk from a GeS4 tetrahedron to a PS4 tetrahedron. Color code: green, Li atoms; yellow, S atoms; blue, P atoms; orange, Ge atoms; pink, the diffusing S atom. | |
The decomposition of H2O also leaves an oxygen atom on the surface, while experimental results of the continuous release of H2S also show that those oxygen atoms do not form a protective layer on the surface to prevent Li10GeP2S12 from contacting the air. This also means O atoms on the surface can diffuse into the bulk. Since Fig. 5 shows that a S atom can diffuse from a GeS4 tetrahedron to a PS4 tetrahedron in the bulk, S vacancies will concentrate on the GeS4 tetrahedra. Therefore, oxygen atoms can diffuse from the surface to the bulk through the GeS4 tetrahedra. Fig. 6(a) shows the energy barriers during the process of O diffusing from the surface to the bulk, an O atom diffusing from GeS3O to PS3O, and an O atom diffusing from PS3O to PS3O, for which the energy barriers are 0.21 eV, 1.99 eV and 2.72 eV, respectively. Fig. 6(b) shows the diffusion path of an oxygen atom from the surface to the bulk. The path through the GeS4 tetrahedron into the bulk is found to have the lowest energy barrier (0.21 eV). However, the energy barriers for O atom diffusion in the bulk are 1.99 eV and 2.72 eV, which are too high for O atoms in the pristine Li10GeP2S12 crystal. Thus, it is hard for O ions to diffuse in the bulk. However, it will lead O ions being deposited in the same area, which will destroy the structure of Li10GeP2S12, and this is also the cause of the miscellaneous peaks in the XRD pattern of Li10GeP2S12 after exposure to air.25
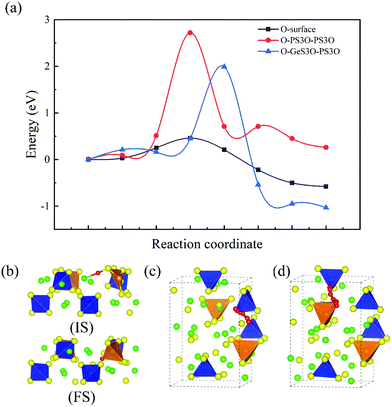 |
| Fig. 6 Minimum energy paths for O atom diffusion. (a) Energy profiles for O atom diffusion. (b) Structural diagram of O atom diffusion from the surface into the bulk. (c) Structural diagram of O atom diffusion in the bulk from a PS3O tetrahedron to a PS3O tetrahedron. (d) Diagram of O atom diffusion in the bulk from a GeS3O tetrahedron to a PS3O tetrahedron. Color code: green, Li atoms; yellow, S atoms; blue, P atoms; orange, Ge atoms; red, O atoms. | |
According to the XRD patterns obtained by Sun et al.,26 PO43− is found in the product of Li10GeP2S12 decomposition in the atmosphere. Mo et al.29 also claimed that Li10GeP2S12 is thermodynamically unstable. Considering the thermodynamic stability, we also calculated the thermodynamic stability of Li10GeP2S12 after being exposed to the air and the degree to which it reacts with H2O. Mo et al.29 also found that Li10GeP2S12 can be decomposed into Li3PS4 and Li4GeS4. The phase decomposition energy (ΔEpd) of Li10GeP2S12−xOx is calculated with respect to the most stable ternary compounds (Li3PS4, Li3PO4, Li4GeS4, and Li4GeO4) to further check the phase stability. The ΔEpd is defined as follows:
|  | (5) |
|  | (6) |
|  | (7) |
| ΔEpd(x = 12) = 2E(Li3PO4) + E(Li4GeO4) − E(Li10GeP2S12−xOx) | (8) |
A negative value of ΔEpd indicates thermodynamic phase stability against decomposition. For the degree of reaction with H2O in air, according to the reaction equilibrium Li10GeP2S12 + H2O →Li10 GeP2O12 + H2S, we define the energy of the generation of H2S as ΔEH2S:
| ΔEH2S = E(Li10GeP2O12)/12 + E(H2S) − E(Li10GeP2S12)/12 − E(H2O) | (9) |
A positive value of ΔEH2S indicates thermodynamic stability against H2S generation. As shown in Fig. 7, we find that the value of ΔEpd decreases as O atoms successively replace the S atoms in Li10GeP2S12. This indicates that the thermal stability of Li10GeP2S12 is continuously improved with the reaction. When x reaches 8 (ΔEH2S = 1.05 eV), Li10GeP2S4O8 is inert to H2O molecules in the air. At the same time, Li10GeP2S4O8 could be decomposed to Li3PO4 and Li4GeS4. This indicates that the S atoms of a PS4 tetrahedron are completely replaced by O atoms, proving that S in H2S comes from the PS4 tetrahedra.
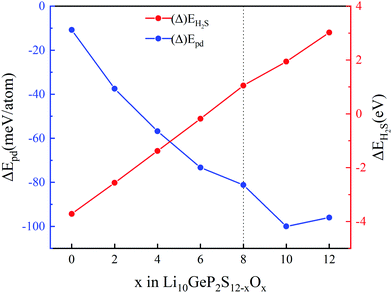 |
| Fig. 7 Phase decomposition (pd) stability (ΔEpd, reaction equilibrium in eqn (5)–(8)) and moisture stability with x in the Li10GeP2S12−xOx. | |
By doping with Sb, Sun et al. successfully inhibited Li10GeP2S12 hydrolysis.26 To analyze the mechanism, we investigated how Sb doping inhibits the hydrolysis of Li10GeP2S12. For the possible doping locations of Sb via thermodynamic calculations, the SbP formation energy (E) was calculated using eqn (10):
| E = E(xSb−LGPS) + x·EP − E(LGPS) − x·ESb | (10) |
We considered two Sb doping sites (on P2b or P4d sites) and five types of substitution (no Sb substitution, 100% on P2b substitution, 100% on P4d substitution, 50%P2b + 50%P4d substitution, and full substitution). The results of the formation energy when Sb occupies different P sites are shown in Table 3. We find that Sb is more inclined to occupy the P4d sites. Fig. 8(a) shows the structure of Li10Ge(P1−xSbx)2S12 with Sb occupying P4d sites. According to the previous calculation of surface energies, the (110) surface is more easily exposed. Fig. 8(b) shows the new surface when Sb is substituted on a surface P4d site. Fig. 8(c) shows the energy profile of H2O adsorption and reaction with an SbS4 tetrahedron on the Li10Ge(P1−xSbx)2S12(110) surface. Fig. 8(d) depicts the structures of the intermediates and the corresponding transition states during the reaction of H2O and a SbS4 tetrahedron on the Li10Ge(P1−xSbx)2S12(110) surface. In the process from IS0 to IS1, H2O molecules are adsorbed at the Li site, and the adsorption energy is −0.33 eV. For the process from IS1 to MS1, the H2O molecule is decomposed to form H+ and OH−, while H+ is bonded to the S on an SbS4 tetrahedron forming SH. The decomposition barrier is 0.22 eV; meanwhile, it releases 0.65 eV for this step. For the process from MS1 to MS2, OH− is decomposed to form H2S with HS adsorbed on the surface, while the remaining O atoms are adsorbed on Li atoms. The barrier of this step is 1.06 eV. MS2 to FS is the H2S desorption process, which needs 0.29 eV. From the decomposition of H2O molecules on SbS4 tetrahedra, the maximum reaction barrier required for the reaction of an H2O molecule with S in a SbS4 tetrahedron to produce H2S gas is 1.06 eV. In the previous calculation, the maximum energy barrier required for H2O molecules to react with S in PS4 tetrahedra to form H2S gas was 0.65 eV. With the increased barrier of OH− decomposition, the substitution of Sb can prevent the hydrolysis of Li10GeP2S12.
Table 3 Relative energy with Sb doping at different P sites
Sb |
No doping |
100% P2b |
100% P4d |
50% P2b + 50% P4d |
Full doping |
E (eV) |
0 |
5.16 |
4.64 |
4.65 |
9.96 |
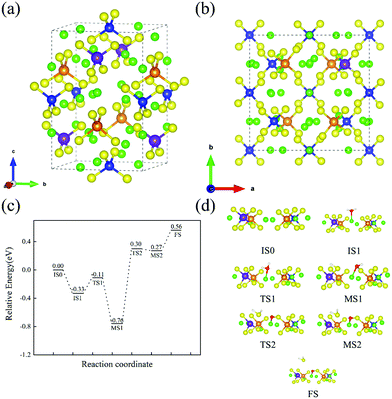 |
| Fig. 8 (a) Crystal structure of Sb substitution on P4d sites; (b) crystal structure of Sb substitution on a P4d site on the (110) surface; (c) H2O reaction energy profile on an SbS4 tetrahedron of the Li10Ge(P1−xSbx)2S12(110) surface; and (d) structural diagram of the minimum energy path for the reaction of H2O with an SbS4 tetrahedron on the Li10Ge(P1−xSbx)2S12(110) surface. Color code: green, Li atoms; yellow, S atoms; blue, P atoms; orange, Ge atoms; red, O atoms; white, H atoms; purple, Sb atoms. | |
In addition, we studied the effect of Sb doping on Li10GeP2S12. The data in Table 4 show that with an increase in Sb doping, the lattice constants of Li10GeP2S12 will also increase. Furthermore, the diffusion barrier of Li ions along the c direction will also increase, which is consistent with the experimental findings of Sun et al.26 that the conductivity of the Li ion decreases with Sb doping. The diffusion channel diameter (D) is defined as the average value of the minimum distances between tetrahedra along the c direction, as shown Fig. 9. From Table 4, we find that diffusion barrier (Eb) increases as D decreases.
Table 4 Lattice constants (a, and c), Li ion channel width D and Li ion diffusion barrier Eb in Li10Ge(P1−xSbx)2S12 related to the amount of Sb doping x
x
|
0 |
0.125 |
0.250 |
0.375 |
0.50 |
a (Å) |
8.75 |
8.78 |
8.79 |
8.81 |
8.82 |
c (Å) |
12.88 |
12.93 |
12.99 |
13.04 |
13.09 |
D (Å) |
4.47 |
4.45 |
4.43 |
4.42 |
4.42 |
E
b (eV) |
0.25 |
0.27 |
0.30 |
0.31 |
0.32 |
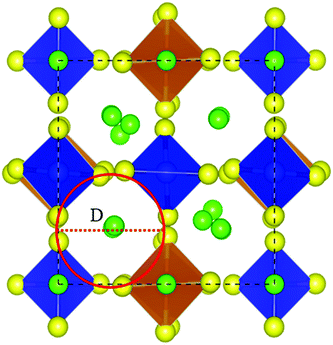 |
| Fig. 9 Lithium-ion diffusion channel in the c-direction (red circle), where the channel radius is D. Color code: green, Li atoms; yellow, S atoms; blue, P atoms; orange, Ge atoms. | |
Conclusion
Based on first principles calculations, we have unveiled the kinetic and thermodynamic mechanism in the reaction of Li10GeP2S12 with H2O molecules in air to produce H2S gas. The surfaces of Li10GeP2S12 exposed to the air are (001), (110) and (111), and H2O molecules are adsorbed on Li+ sites. The rate-determining step for the H2S-releasing reaction is the decomposition of OH− with an energy barrier of 0.65 eV. The S atoms can diffuse between PS4 tetrahedra, and the diffusion barrier is 0.82 eV. Moreover, S can diffuse from the bulk to the surface with a diffusion energy barrier of 0.45 eV. The O atoms can diffuse from the surface into the bulk, and the diffusion energy barrier is 0.21 eV. According to the thermodynamic analysis, the formation of Li10GeP2S4O8 will inhibit the reaction with H2O molecules and prevent the release of H2S gas. Sb doping could also prevent the hydrolysis of Li10GeP2S12 due to the increased energy barrier (1.06 eV) for the decomposition of H2O on the SbS4 tetrahedra. The diffusion barrier of the Li ions in Li10Ge(P1−xSbx)2S12 will also increase with the amount of Sb doping.
Conflicts of interest
There are no conflicts to declare.
Acknowledgements
This work is supported by the computation and simulation fund for material sciences of Ningbo University, and by the Guangdong Provincial Key Laboratory of Energy Materials for Electric Power under Grant No. 2018B030322001. Computing time was partially supported by the Center for Computational Science and Engineering of the Southern University of Science and Technology.
References
- C. Zhang, C. Lu, F. Zhang, F. Qiu, X. Zhuang and X. Feng, Two-dimensional organic cathode materials for alkali-metal-ion batteries, J. Energy Chem., 2018, 27(1), 86–98 CrossRef.
- X. Zhan, Z. Chen and Q. Zhang, Recent progress in two-dimensional COFs for energy-related applications, J. Mater. Chem. A, 2017, 5(28), 14463–14479 RSC.
- J. Xie, C. E. Zhao, Z. Q. Lin, P. Y. Gu and Q. Zhang, Nanostructured Conjugated Polymers for Energy-Related Applications beyond Solar Cells, Chem. – Asian J., 2016, 11(10), 1489–1511 CrossRef CAS PubMed.
- W. Liu, X. Li, D. Xiong, Y. Hao, J. Li, H. Kou, B. Yan, D. Li, S. Lu, A. Koo, K. Adair and X. Sun, Significantly improving cycling performance of cathodes in lithium ion batteries: The effect of Al2O3 and LiAlO2 coatings on LiNi0.6Co0.2Mn0.2O2, Nano Energy, 2018, 44, 111–120 CrossRef CAS.
- Y. Chu, Y. Shen, F. Guo, X. Zhao, Q. Dong, Q. Zhang, W. Li, H. Chen, Z. Luo and L. Chen, Advanced Characterizations of Solid Electrolyte Interphases in Lithium-Ion Batteries, Electrochem. Energy Rev., 2020, 3, 187–219 CrossRef.
- P.-J. Lian, B.-S. Zhao, L.-Q. Zhang, N. Xu, M.-T. Wu and X.-P. Gao, Inorganic sulfide solid electrolytes for all-solid-state lithium secondary batteries, J. Mater. Chem. A, 2019, 7(36), 20540–20557 RSC.
- D. Monroe, Building a better battery, MRS Bull., 2020, 45(3), 246–247 CrossRef.
- F. Zheng, M. Kotobuki, S. Song, M. O. Lai and L. Lu, Review on solid electrolytes for all-solid-state lithium-ion batteries, J. Power Sources, 2018, 389, 198–213 CrossRef CAS.
- G. Zhou, F. Li and H.-M. Cheng, Progress in flexible lithium batteries and future prospects, Energy Environ. Sci., 2014, 7(4), 1307–1338 RSC.
- E. Quartarone and P. Mustarelli, Electrolytes for solid-state lithium rechargeable batteries: recent advances and perspectives, Chem. Soc. Rev., 2011, 40(5), 2525–2540 RSC.
- X. Han, Y. Gong, K. K. Fu, X. He, G. T. Hitz, J. Dai, A. Pearse, B. Liu, H. Wang, G. Rubloff, Y. Mo, V. Thangadurai, E. D. Wachsman and L. Hu, Negating interfacial impedance in garnet-based solid-state Li metal batteries, Nat. Mater., 2017, 16(5), 572–579 CrossRef CAS PubMed.
- Y. Ren, K. Chen, R. Chen, T. Liu, Y. Zhang, C.-W. Nan and B. Vyas, Oxide Electrolytes for Lithium Batteries, J. Am. Ceram. Soc., 2015, 98(12), 3603–3623 CrossRef CAS.
- W. Xiao, J. Wang, L. Fan, J. Zhang and X. Lia, Recent advances in Li1+xAlxTi2−x(PO4)3 solid-state electrolyte for safe lithium batteries, Energy Storage Mater., 2019, 19, 379 CrossRef.
- E. Rangasamy, Z. Liu, M. Gobet, K. Pilar, G. Sahu, W. Zhou, H. Wu, S. Greenbaum and C. Liang, An iodide-based Li7P2S8I superionic conductor, J. Am. Chem. Soc., 2015, 137(4), 1384–1387 CrossRef CAS PubMed.
- Y. Kato, S. Hori, T. Saito, K. Suzuki, M. Hirayama, A. Mitsui, M. Yonemura, H. Iba and R. Kanno, High-power all-solid-state batteries using sulfide superionic conductors, Nat. Energy, 2016, 1, 4 Search PubMed.
- F. Wu, W. Fitzhugh, L. Ye, J. Ning and X. Li, Advanced sulfide solid electrolyte by core-shell structural design, Nat. Commun., 2018, 9(1), 4037 CrossRef PubMed.
- H. Muramatsu, A. Hayashi, T. Ohtomo, S. Hama and M. Tatsumisago, Structural change of Li2S–P2S5 sulfide solid electrolytes in the atmosphere, Solid State Ionics, 2011, 182(1), 116–119 CrossRef CAS.
- T. Kaib, S. Haddadpour, M. Kapitein, P. Bron, C. Schröder, H. Eckert, B. Roling and S. Dehnen, New Lithium Chalcogenidotetrelates, LiChT: Synthesis and Characterization of the Li+-Conducting Tetralithium ortho-Sulfidostannate Li4SnS4, Chem. Mater., 2012, 24(11), 2211–2219 CrossRef CAS.
- K. Kanazawa, S. Yubuchi, C. Hotehama, M. Otoyama, S. Shimono, H. Ishibashi, Y. Kubota, A. Sakuda, A. Hayashi and M. Tatsumisago, Mechanochemical Synthesis and Characterization of Metastable Hexagonal Li4SnS4 Solid Electrolyte, Inorg. Chem., 2018, 57(16), 9925–9930 CrossRef CAS PubMed.
- K. H. Park, D. Y. Oh, Y. E. Choi, Y. J. Nam, L. Han, J. Y. Kim, H. Xin, F. Lin, S. M. Oh and Y. S. Jung, Solution-Processable Glass LiI-Li4 SnS4 Superionic Conductors for All-Solid-State Li-Ion Batteries, Adv. Mater., 2016, 28(9), 1874–1883 CrossRef CAS PubMed.
- G. Sahu, Z. Lin, J. Li, Z. Liu, N. Dudney and C. Liang, Air-stable, high-conduction solid electrolytes of arsenic-substituted Li4SnS4, Energy Environ. Sci., 2014, 7(3), 1053–1058 RSC.
- Z. Zhang, J. Zhang, Y. Sun, H. Jia, L. Peng, Y. Zhang and J. Xie, Li4-Sb Sn1-S4 solid solutions for air-stable solid electrolytes, J. Energy Chem., 2020, 41, 171–176 CrossRef.
- H. Kwak, K. H. Park, D. Han, K.-W. Nam, H. Kim and Y. S. Jung, Li+ conduction in air-stable Sb-Substituted Li4SnS4 for all-solid-state Li-Ion batteries, J. Power Sources, 2020, 446 Search PubMed.
- R. G. Pearson, Hard and soft acids and bases, HSAB, part 1: Fundamental principles, J. Chem. Educ., 1968, 45, 9 CrossRef.
- A. Hayashi, H. Muramatsu, T. Ohtomo, S. Hama and M. Tatsumisago, Improvement of chemical stability of Li3PS4 glass electrolytes by adding MxOy (M = Fe, Zn, and Bi) nanoparticles, J. Mater. Chem. A, 2013, 1, 21 RSC.
- J. Liang, N. Chen, X. Li, X. Li, K. R. Adair, J. Li, C. Wang, C. Yu, M. Norouzi Banis, L. Zhang, S. Zhao, S. Lu, H. Huang, R. Li, Y. Huang and X. Sun, Li10Ge(P1−xSbx)2S12 Lithium-Ion Conductors with Enhanced Atmospheric Stability, Chem. Mater., 2020, 32(6), 2664–2672 CrossRef CAS.
- N. Kamaya, K. Homma, Y. Yamakawa, M. Hirayama, R. Kanno, M. Yonemura, T. Kamiyama, Y. Kato, S. Hama, K. Kawamoto and A. Mitsui, A lithium superionic conductor, Nat. Mater., 2011, 10(9), 682–686 CrossRef CAS PubMed.
- S. Adams and R. Prasada Rao, Structural requirements for fast lithium ion migration in Li10GeP2S12, J. Mater. Chem., 2012, 22(16), 7687–7691 RSC.
- Y. Mo, S. P. Ong and G. Ceder, First Principles Study of the Li10GeP2S12 Lithium Super Ionic Conductor Material, Chem. Mater., 2011, 24(1), 15–17 CrossRef.
- X. Liang, L. Wang, Y. Jiang, J. Wang, H. Luo, C. Liu and J. Feng, In-Channel and In-Plane Li Ion Diffusions in the Superionic Conductor Li10GeP2S12 Probed by Solid-State NMR, Chem. Mater., 2015, 27(16), 5503–5510 CrossRef CAS.
- A. Miura, N. C. Rosero-Navarro, A. Sakuda, K. Tadanaga, N. H. H. Phuc, A. Matsuda, N. Machida, A. Hayashi and M. Tatsumisago, Liquid-phase syntheses of sulfide electrolytes for all-solid-state lithium battery, Nat. Rev. Chem., 2019, 3(3), 189–198 CrossRef CAS.
- Y. Wang, Z. Liu, X. Zhu, Y. Tang and F. Huang, Highly lithium-ion conductive thio-LISICON thin film processed by low-temperature solution method, J. Power Sources, 2013, 224, 225–229 CrossRef CAS.
- J. P. Perdew, K. Burke and M. Ernzerhof, Generalized gradient approximation made simple (vol 77, pg 3865, 1996), Phys. Rev. Lett., 1997, 78(7), 1396 CrossRef CAS.
- P. E. Blochl, Projector augmented-wave method, Phys. Rev. B: Condens. Matter Mater. Phys., 1994, 50(24), 17953–17979 CrossRef PubMed.
- G. Kresse and D. Joubert, From ultrasoft pseudopotentials to the projector augmented-wave method, Phys. Rev. B: Condens. Matter Mater. Phys., 1999, 59(3), 1758–1775 CrossRef CAS.
- G. Kresse and J. Furthmuller, Efficient iterative schemes for ab initio total-energy calculations using a plane-wave basis set, Phys. Rev. B: Condens. Matter Mater. Phys., 1996, 54(16), 11169–11186 CrossRef CAS PubMed.
- G. Henkelman, B. P. Uberuaga and H. Jónsson, A climbing image nudged elastic band method for finding saddle points and minimum energy paths, J. Chem. Phys., 2000, 113(22), 9901–9904 CrossRef CAS.
- K. Oh, D. Chang, B. Lee, D.-H. Kim, G. Yoon, I. Park, B. Kim and K. Kang, Native Defects in Li10GeP2S12 and Their Effect on Lithium Diffusion, Chem. Mater., 2018, 30(15), 4995–5004 CrossRef CAS.
- Y. Zhu and Y. Mo, Materials Design Principles for Air-Stable Lithium/Sodium Solid Electrolytes, Angew. Chem., Int. Ed., 2020, 59(40), 17472–17476 CrossRef CAS PubMed.
|
This journal is © The Royal Society of Chemistry 2022 |